- Department of Pulmonology, University Hospital Zurich, University of Zurich, Zurich, Switzerland
Background: Pure oxygen breathing (hyperoxia) may improve hemodynamics in patients with pulmonary hypertension (PH) and allows to calculate right-to-left shunt fraction (Qs/Qt), whereas breathing normobaric hypoxia may accelerate hypoxic pulmonary vasoconstriction (HPV). This study investigates how hyperoxia and hypoxia affect mean pulmonary artery pressure (mPAP) and pulmonary vascular resistance (PVR) in patients with PH and whether Qs/Qt influences the changes of mPAP and PVR.
Study Design and Methods: Adults with pulmonary arterial or chronic thromboembolic PH (PAH/CTEPH) underwent repetitive hemodynamic and blood gas measurements during right heart catheterization (RHC) under normoxia [fractions of inspiratory oxygen (FiO2) 0.21], hypoxia (FiO2 0.15), and hyperoxia (FiO2 1.0) for at least 10 min.
Results: We included 149 patients (79/70 PAH/CTEPH, 59% women, mean ± SD 60 ± 17 years). Multivariable regressions (mean change, CI) showed that hypoxia did not affect mPAP and cardiac index, but increased PVR [0.4 (0.1–0.7) WU, p = 0.021] due to decreased pulmonary artery wedge pressure [−0.54 (−0.92 to −0.162), p = 0.005]. Hyperoxia significantly decreased mPAP [−4.4 (−5.5 to −3.3) mmHg, p < 0.001] and PVR [−0.4 (−0.7 to −0.1) WU, p = 0.006] compared with normoxia. The Qs/Qt (14 ± 6%) was >10 in 75% of subjects but changes of mPAP and PVR under hyperoxia and hypoxia were independent of Qs/Qt.
Conclusion: Acute exposure to hypoxia did not relevantly alter pulmonary hemodynamics indicating a blunted HPV-response in PH. In contrast, hyperoxia remarkably reduced mPAP and PVR, indicating a preserved vasodilator response to oxygen and possibly supporting the oxygen therapy in patients with PH. A high proportion of patients with PH showed increased Qs/Qt, which, however, was not associated with changes in pulmonary hemodynamics in response to changes in FiO2.
Background
Due to the increase of global mobility and advances in infrastructure, every year millions of people expose themselves to hypobaric hypoxic environments, such as high altitude or commercial air travel. In healthy individuals, a low inspired and alveolar oxygen concentration causes hypoxic pulmonary vasoconstriction (HPV), which in turn increases pulmonary vascular resistance (PVR) and pulmonary arterial pressure (PAP) (1). When exposed to a hypoxic environment, HPV could be detrimental in patients with pre-existing precapillary pulmonary hypertension (PH) due to pulmonary vascular disease (2–4), including pulmonary arterial and chronic thromboembolic PH (PAH and CTEPH). To date, only few studies have measured PAP and PVR in precapillary PH under hypoxia during the right heart catheterization (RHC) (5, 6).
Contrarily, acute exposure to high fractions of inspiratory oxygen (FiO2) may reduce PAP and PVR in patients with PH through pulmonary vasodilation, though there are only few studies with hemodynamic data obtained during RHC (6–8). The exact mechanism of pulmonary vasodilation in response to oxygen in precapillary PH remains under investigation. Some evidence suggests that the predominantly alveolar endothelial oxygen sensing and, to a lesser degree, direct oxyhemoglobin sensing in mixed venous blood are involved, but data on the influence of mixed venous oxygen partial pressures on the hemodynamic response to oxygen in patients with PH are lacking (7). Pulmonary vasodilation by oxygen supplementation might provide a physiological basis for supporting long-term oxygen therapy in PH, which was shown to improve exercise capacity and symptom relief in domiciliary and nocturnal settings (9, 10).
Breathing pure oxygen (hyperoxia, FiO2 = 1.0) additionally allows calculating the fraction of right-to-left shunt (Qs/Qt) (11). We hypothesized that the size of Qs/Qt may influence PAP and PVR and their response to changes in FiO2 in patients with precapillary PH. Right-to-left shunts are physiological or anatomical short circuits, which occur when blood flows from the right to the left side of the heart without passing through the pulmonary capillaries. Physiological shunts occur when non-ventilated alveoli are perfused (12). Anatomical right-to-left shunts are most frequently located in the heart, with the patent foramen ovale (PFO) constituting the most common pathology with an estimated prevalence of 17–30% in the general population and similarly in patients with PH (13–18), or are located in the lungs as intrapulmonary arteriovenous anastomoses (IPAVA) without direct contact to the alveolar system (19). An increased Qs/Qt might be associated with distinct exaggerated hemodynamic changes under hypoxia (3) or could reflect a potential escape valve mechanism on increased PAP.
In this study, we aim to investigate the effect of hypoxia (FiO2 0.15) and hyperoxia (FiO2 1.0) on pulmonary hemodynamics (mPAP, PVR) in a large cohort of patients with PH assessed by RHC as well as the influence of Qs/Qt on hemodynamic parameters, which might provide insight on physiological mechanisms supporting the use of therapeutic oxygen in this patient collective.
Methods
Study Design and Participants
This is a retrospective study analyzing data obtained in patients with precapillary PH due to pulmonary vascular disease (PAH/CTEPH) during clinically indicated RHC at the University Hospital Zurich, 490 m altitude. This study was conducted in concordance with the amended Helsinki declaration, approved by the cantonal ethics committee Zurich (No. 2020-01541) and all patients provided written informed consent.
We included men and women, aged ≥18 years with mean PAP (mPAP) > 20 mmHg, pulmonary artery wedge pressure (PAWP) ≤ 15 mmHg) and revealing a PVR > 2.0 WU (20–22) referred to our clinic between March 2016 and June 2020 for RHC and who had undergone measurements of hemodynamic parameters while breathing ambient air (normoxia FiO2 0.21), hypoxia (FiO2 0.15), and hyperoxia (FiO2 1.0) and who were classified as PAH or CTEPH according to a thorough investigation, including pulmonary function tests, CT-pulmonary angiography, ventilation-perfusion scan, serology, and other measures as required. We excluded severely hypoxemic patients (PaO2 <7.3 kPa on ambient air) who could not undergo hypoxia testing (21), patients after lung transplantation, and patients with other PH-classifications (2).
Measurements
Supine resting RHC was performed via jugular venous access with a balloon-tipped, triple-lumen, fluid-filled 7.5 French Swan-Ganz catheter (Baxter/Edwards, Deerfield, IL, USA) as described (6, 23). Transducers were placed at the mid-thoracic level and zeroed to atmospheric pressure (24, 25). Systolic, mean, and diastolic PAP, right atrial pressure (RAP), and PAWP were averaged over several respiratory cycles (Dräger, Liebefeld, Switzerland) (23, 25, 26). CO was measured by thermodilution (Baxter/Edwards, Deerfield, IL, USA) and indexed for body surface area (BSA) to obtain cardiac index (CI). Blood samples were drawn from radial artery puncture and pulmonary artery catheter tips were analyzed directly (RapidPoint 500, Siemens, Zurich, Switzerland) to obtain arterial partial pressures of oxygen and carbon dioxide (PaO2, PaCO2), arterial saturation of oxygen (SaO2), mixed venous partial pressure (PmvO2) and saturation of oxygen (SmvO2), hemoglobin (Hb), and lactate.
Patients were asked to rest supine for at least 10 min and baseline hemodynamic parameters and blood gas samples were obtained under normoxia (FiO2 0.21). Patients then received hypoxia (FiO2 0.15) over an airtight mouthpiece (AltiTrainer, SMTEC, Nyon, Switzerland) for at least 10 min, after which hemodynamics and blood gas samples were obtained a second time while still breathing hypoxia. Following a washout phase of at least 10 min, patients received pure oxygen (hyperoxia, FiO2 1) for 10 min administered via a non-rebreathing valve from a reservoir bag (AmbuSPUR-II, Synmedic, Zurich, Switzerland), after which hemodynamics and blood samples were obtained for the third time while still breathing hyperoxia and shunt fraction was calculated with the following formula (11):
The standard barometric atmospheric pressure at 490 m (PB) was assumed to be 717.7 mmHg, the partial pressure of water at body temperature (PH2O) assumed to be 47.1 mmHg at 37°C, the respiratory quotient (R) was assumed to be 0.8, and pulmonary capillary oxygen saturation (ScapO2) was assumed to be 100% under FiO2 1.0. The factor 0.0031 is the solubility coefficient of oxygen in the blood and the factor 1.34 is the ratio of oxygen in milliliters per gram of Hb.
Outcomes
The main outcomes consisted of the acute change of mPAP and PVR under hypoxia (FiO2 0.15) and hyperoxia (FiO2 1.0). Further outcomes included the size of Qs/Qt and the influence of Qs/Qt, PaO2, and PmvO2 on hemodynamic parameters (mPAP, PVR, CI, and PAWP) measured under normoxia, hypoxia, and hyperoxia, and subgroup-analysis separately for PAH and CTEPH.
The statistical analysis included all available measurements under normoxia, hypoxia, and hyperoxia. Single missing values were not replaced. All statistical analyses were performed using R Studio (version 1.2.1578, R Studio Inc., San Francisco, CA, USA). Analyses of hemodynamic measures (mPAP, PVR, PVR Index, CI, and PAWP) were performed in univariable (FiO2 = normoxia vs. hypoxia vs. hyperoxia) and multivariable mixed linear regression models, with Qs/Qt, PH group (PAH or CTEPH), age, sex, carbon monoxide transfer coefficient (KCO), PaO2, and PmvO2 as independent variables, and grouped by subject. Coefficients are expressed as mean change and 95% CI. We tested regression model fitting with QQ-plots and fitted residual and random intercept plots. Multi-collinearity was excluded with variance inflation factor testing. Continuous data are reported as mean ± SD and categorical data as number (percentage). A two-sided p-value of < 0.05 or a 95% CI not crossing 0 was considered statistically significant.
Results
Study Population
We included 149 patients with PH, 79 PAH, and 70 CTEPH (Figure 1). Patients with PAH were predominantly women (i.e., 70% women), whereas sex distribution was balanced in patients with CTEPH (47% women) (Table 1; Supplementary Table 1). The vast majority of patients were incident without any therapy (90% PAH and 87% CTEPH), a minority of patients with CTEPH had RHC 6–12 months after pulmonary endarterectomy and some patients with PAH were medically pretreated. Pulmonary function tests from the patient records revealed mildly decreased forced expiration in the first second of expiration (FEV1) and forced vital capacity (FVC), as well as mildly decreased diffusing capacity of lung carbon monoxide (DLCO) and KCO values.
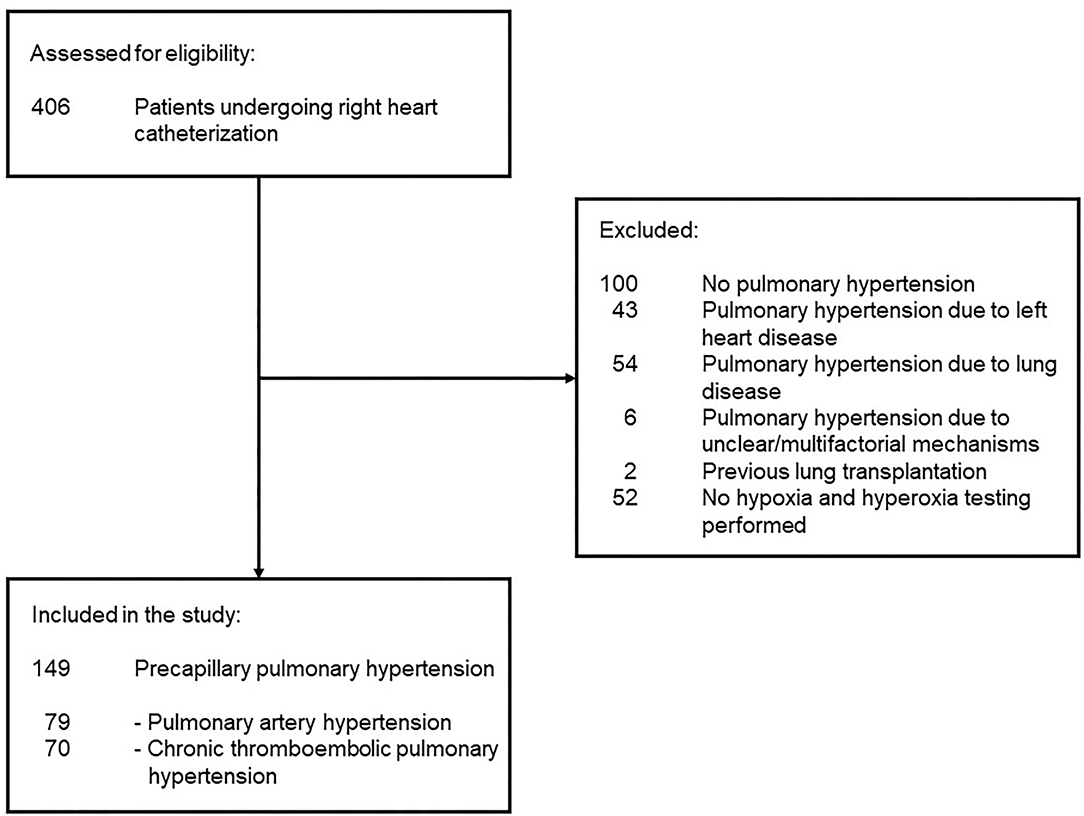
Figure 1. Patient flow is shown. No pulmonary hypertension classified patients with a mean pulmonary artery pressure <25 mmHg and a pulmonary vascular resistance <2 WU.
Resting Hemodynamics Under Different FiO2
Under normoxia, patients with PH showed an increased mPAP and PVR and mild hypoxemia according to inclusion criteria (Table 2 and Figure 2). Under hypoxia, a univariable mixed linear regression analysis adjusted for FiO2 showed that the mPAP did not change significantly compared with normoxia, while the PVR increased by 0.35 WU and the PAWP decreased by 0.5 mmHg. Arterial and mixed venous oxygen and carbon dioxide decreased.
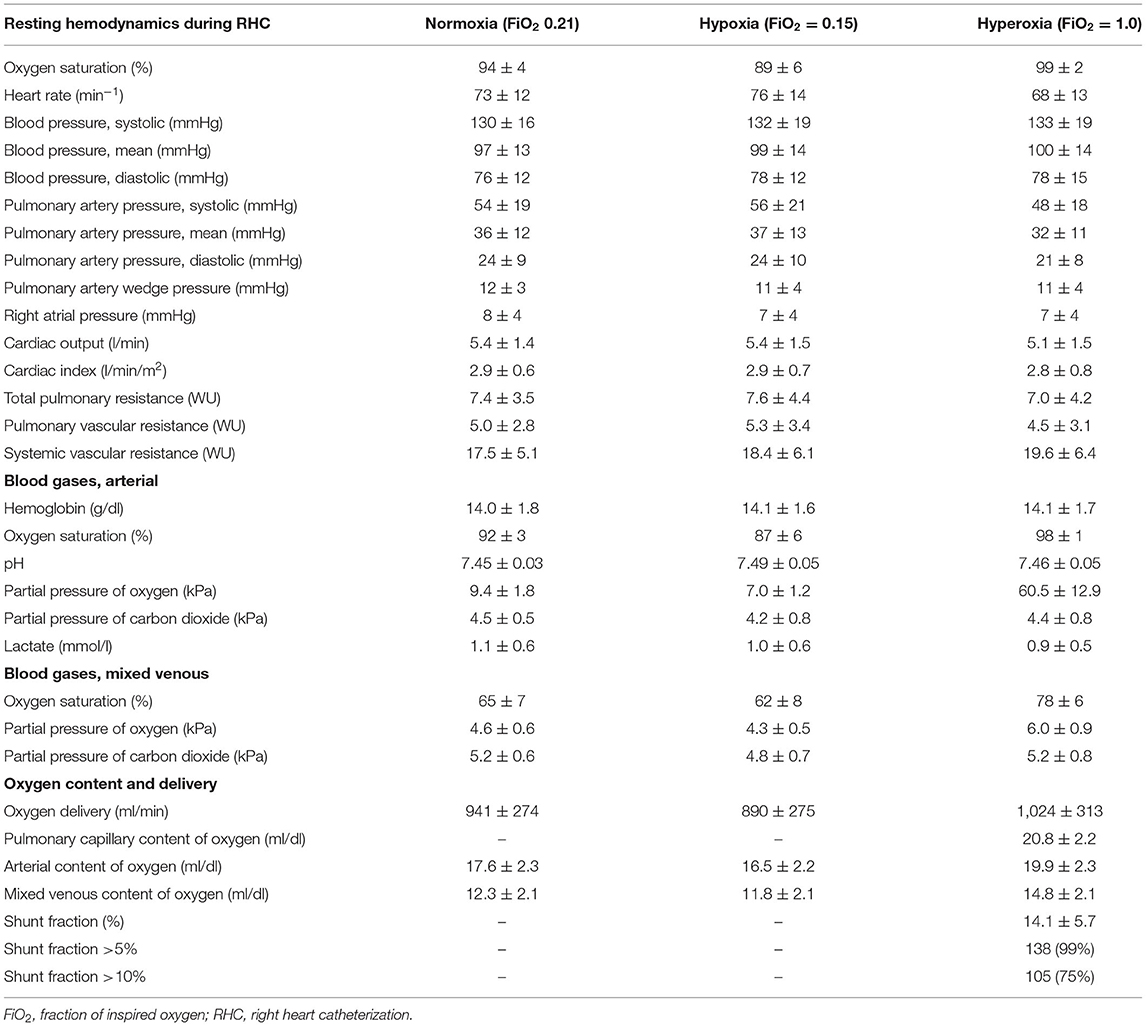
Table 2. Resting hemodynamics and blood gases under normoxic, hypoxic, and hyperoxic air breathing (normoxia, hypoxia, and hyperoxia).
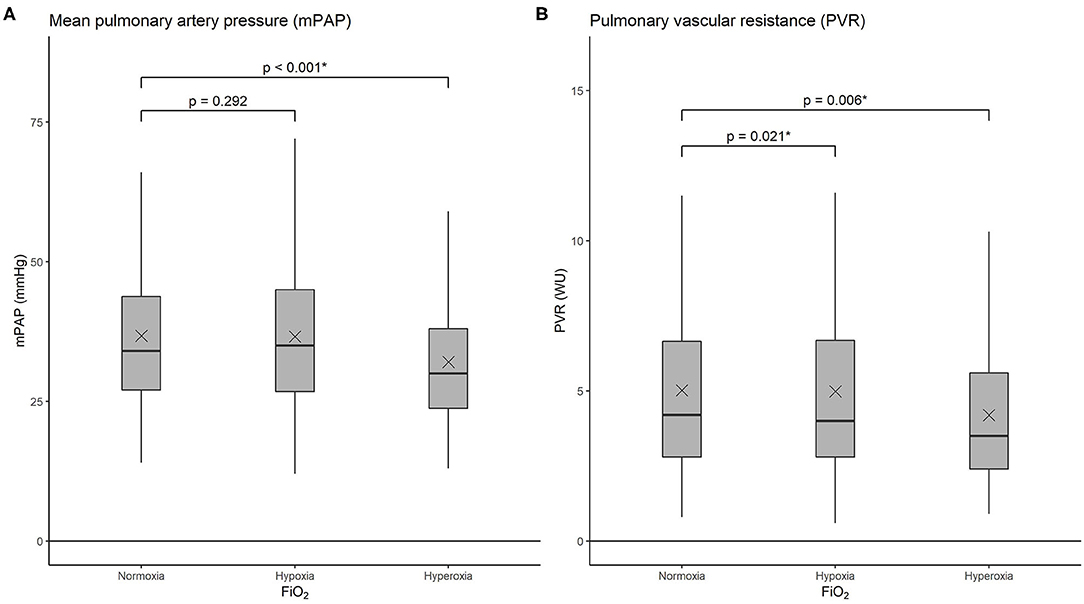
Figure 2. Boxplots showing mean pulmonary artery pressure (mPAP) (A) and pulmonary vascular resistance (PVR) (B) under normoxia (FiO2 0.21), hypoxia (FiO2 0.15), and hyperoxia (FiO2 1.0). The horizontal lines represent the median values, upper and lower box edges represent the 25th and 75th percentiles, and “x” indicates the mean. FiO2: fraction of inspired oxygen.
During acute exposure to hyperoxia, univariable mixed models showed a significant reduction in mPAP of 4.4 mmHg and PVR decreased by 0.43 WU. PAWP (−0.5 mmHg) and CI (−0.15 l/min/m2) significantly decreased compared with normoxia. PaO2 and PmvO2 rose (Tables 2, 3). These changes in hemodynamic parameters persisted after adjustment for age, sex, PH-classification, KCO, and Qs/Qt in multivariable mixed models (Table 3). The magnitude of these hemodynamic changes remained largely similar if the two large subgroups, PAH and CTEPH, were analyzed separately (Supplementary Tables 1–4).
Univariable regression models adjusted for arterial and mixed venous oxygen partial pressures (independent of administered FiO2), revealed that mPAP and PVR were negatively associated with PaO2 and PmvO2 (Table 4), also when PAH and CTEPH were analyzed separately. Whereas, CI was negatively associated with PaO2 but not PmvO2, PAWP was independent of both (Table 4). Combined in a multivariable mixed linear regression analysis adjusted for age, sex, PH-class, and Qs/Qt, neither mPAP and PVR were significantly inversely associated with PaO2, while both were independently associated with PmvO2 (Table 4).
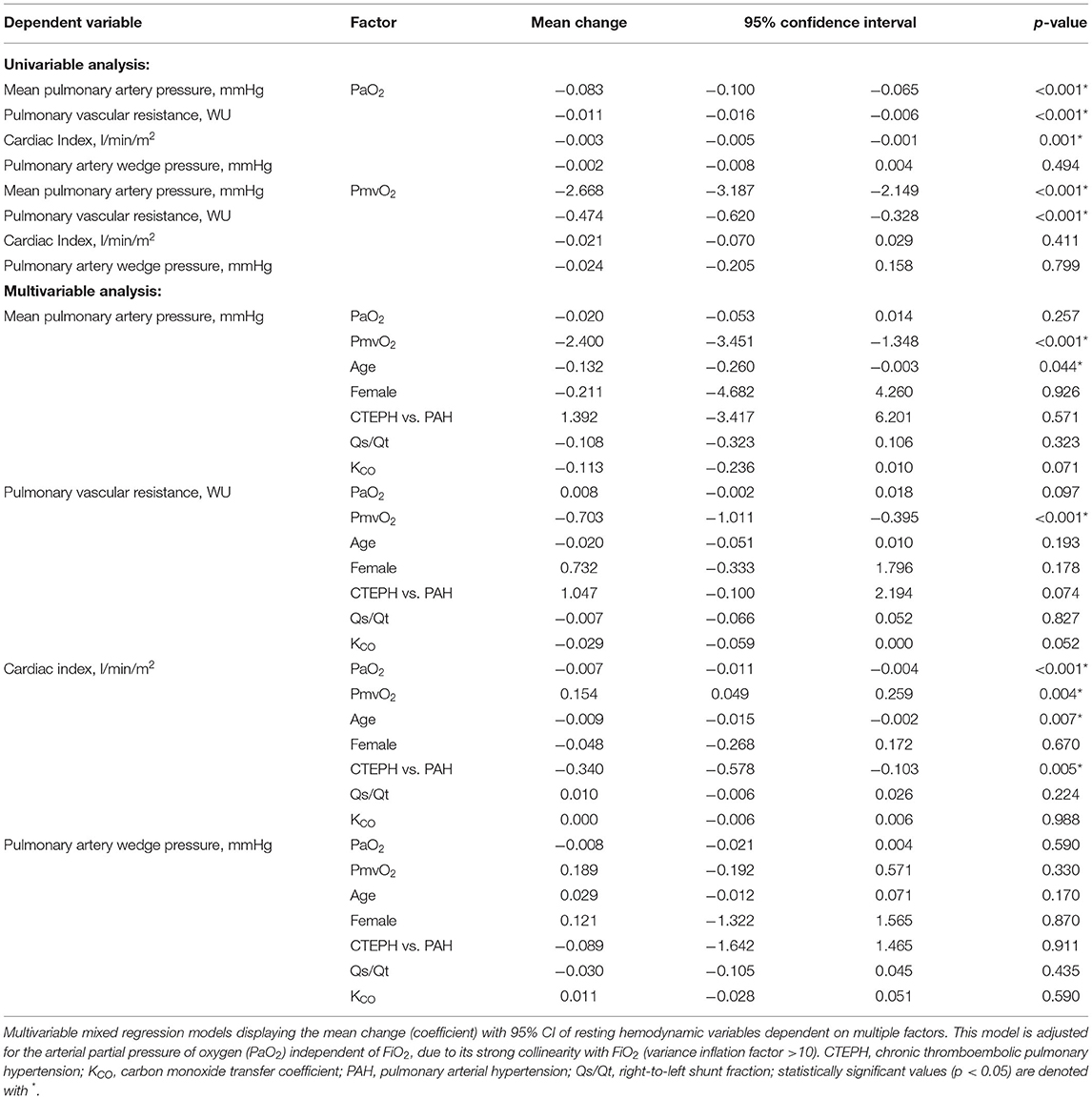
Table 4. Mixed linear regression analysis of hemodynamic parameters dependent on arterial and mixed oxygen partial pressures, independent of FiO2.
Fraction of right-to-left shunt (Qs/Qt) calculated under hyperoxia was 14.1 ± 5.7%, with 138/139 (99%) subjects showing Qs/Qt levels above 5% and 105/139 (75%) above 10%. There was no significant difference in Qs/Qt between patients with PAH (14.5 ± 5.5%) and CTEPH (14.2 ± 6.7%). The Qs/Qt values could not be calculated in 10 subjects due to missing blood gas values (Table 2). Both mPAP and PVR showed a significant negative association with KCO, but were unaffected by age, sex, PH class, and Qs/Qt, while mPAP and PAWP were independent of all adjusting variables, including KCO. CI showed a positive association with Qs/Qt, a negative association with age and was decreased in patients with CTEPH compared with PAH, but independent of sex (Table 4).
Discussion
In this large cohort of patients with PAH/CTEPH assessed by RHC, we found that breathing pure oxygen for 10 min significantly reduced mPAP on average by 4.4 mmHg, a reduced size that is remarkable and within the range of vasodilator drugs, along with a decrease in PVR by 0.43 WU, and the size of these hemodynamic changes was similar in the PAH and CTEPH subgroups (27, 28). This finding indicates an acute vasodilatory effect of oxygen and encourages to further explore the therapeutic value of oxygen in pulmonary vascular diseases in short- and long-term settings and may provide an underlying physiological explanation for the benefit from nocturnal or domiciliary oxygen therapy in terms of improvement of exercise capacity and symptoms in PH. Contrarily, the mPAP was unchanged in response to hypoxia, which may indicate that the altered pulmonary vasculature in PH may not reveal HPV, but still dilates to hyperoxia. In regard to the unchanged mPAP and CI, the increased PVR with hypoxia was solely driven by a minor but significant decrease in PAWP. The changes of mPAP and PVR under different FiO2 were not associated with the size of the Qs/Qt.
Pulmonary Hemodynamics Under Different FiO2
This hitherto largest study investigating pulmonary hemodynamics by RHC in patients with pulmonary vascular diseases showed that changes in FiO2 had distinct effects on resting hemodynamics. Under hypoxia, we confirmed our previous and potentially unforeseen finding that in this large collective of patients with PH, hypoxia had no effect on mPAP (6). We did observe an increase in PVR, but as CI did not change under hypoxia, the increase in PVR under hypoxia was driven by a minor decrease in PAWP, so that the total pulmonary resistance remained unchanged. However, as PAWP was independent of both PaO2 and PmvO2, it seems that the change of PAWP was unrelated to the changes in oxygen partial pressures. Moreover, we observed a slight, but significant decrease of PAWP during hyperoxia, further indicating that the changes in PAWP are likely unrelated to FiO2 or oxygen partial pressures. We hypothesize that breathing through a tube connected to the airtight mouthpiece might have slightly modified intrathoracic pressures which could have contributed to the slightly lowered venous return and thus the PAWP during both hypoxia and hyperoxia exposure. Thus, the present results indicate that patients with PH with a chronically diseased pulmonary vasculature, acute HPV as found in healthy subjects may be blunted (29–32). Whether this is due to the altered mitochondrial redox signaling or altered response of potassium or calcium channels of diseased pulmonary artery smooth muscle cells in PH remains to be determined (33). It equally remains to be seen, whether long-term exposure to hypoxia, as during mountain travel, would worsen the pulmonary hemodynamics in patients with PH.
On the other hand, when patients with PH breathed pure oxygen (hyperoxia), we observed a large decrease in mPAP and PVR. The decreased PVR is especially remarkable as also the CI and the PAWP decreased during oxygen breathing, which would both, in turn, increase the PVR. Both the decrease in CI and PAWP is consistent with previous reports (7). A possible explanation for the largely improved pulmonary hemodynamics with oxygen might be the high FiO2 used, both in the present and in previous studies (7). However, a previous study by Leuchte et al. found that oxygen given at a flow rate of 5 l/min to achieve a SpO2 > 90% improved mPAP and PVR in patients with PH, suggesting that lower flow rates could already achieve clinically important improvements (8). It is further remarkable, that pure oxygen breathing in this acute setting gave for 10' comparably improved hemodynamics in PAH and CTEPH. Whether oxygen therapy would reveal a beneficial effect on pulmonary hemodynamics and especially functional parameters in long-term studies and which level of FiO2 would be sufficient remains to be determined in future well-designed randomized-controlled trials.
The mechanism of action of oxygen therapy in patients with pulmonary vascular disease is not exactly known. A recently published review suggested that acute oxygen induced pulmonary vasodilation is predominantly mediated by alveolar mechanisms and may not even require correction of hypoxemia, although data on mixed venous oxygen content levels were not reported (7). To further explore this mechanism, we calculated linear regression models focusing on the effects of PaO2 and PmvO2 on pulmonary hemodynamics. We found that mPAP and PVR were inversely and linearly dependent on PaO2 and PmvO2, with the effect of PmvO2 on both mPAP and PVR being greater in magnitude than PaO2. While there is no evidence that HPV is both dependent on PaO2 and PmvO2, with PaO2 as directly related to the alveolar PO2 being the more important factor (34), these models suggest that the mixed venous hypoxemia plays a role in pulmonary vasoconstriction. Patients with PH reveal mostly normal lung functions so that alveolar hypoxia inducing HPV should not majorly contribute to hypoxemia, which is usually attributed to a low mixed venous oxygenation induced by a low cardiac output (35). In precapillary PH, the culprit vascular lesions that contribute to the increased pressure and resistance are mainly located upstream of the pulmonary capillaries and alveoli before the most gas exchange takes place, although capillary and pulmonary venular remodeling is also found (36, 37). Whether changes in pulmonary precapillary vasculature modify mixed venous oxygen sensing and thus pulmonary vasoconstriction and dilation remain to be investigated.
To summarize, although the duration of exposure to oxygen was short and the FiO2 high, our findings may support the therapeutic value of oxygen in pulmonary vascular diseases in a short-term setting and may provide some physiological explanation of the effect of nocturnal or domiciliary oxygen therapy on improvement of exercise capacity and symptoms as previously shown, but future studies are needed to investigate the long-term effects on pulmonary hemodynamics (9, 10).
The Influence of Diffusion Capacity for Carbon Dioxide (KCO) on Pulmonary Hemodynamics
In the multivariable regression analyses, we saw that both mPAP and PVR are negatively associated with the carbon monoxide transfer coefficient (Kco). These findings indicate that patients with more severely compromised pulmonary hemodynamics reveal higher impairments of pulmonary gas exchange, or vice versa, which might be associated with more severe vascular remodeling. Strikingly, even after adjustment for KCO, the effect size of both hypoxia and hyperoxia remained the same, indicating that the effect of different FiO2 in our regression model remains robust.
Hemodynamics Dependent on Qs/Qt
Almost all patients (99%) showed Qs/Qt above the physiological threshold of 5 and 75% above the clinically more frequently used threshold of 10% (11). This is comparable with a previous study in which all 87 patients with PH revealed increased Qs/Qt (17). Interestingly, in the present study, changes in resting hemodynamic parameters under hypoxia and hyperoxia were all independent of the size of Qs/Qt. Thus, our data did not support the hypothesis that patients with an increased right-to-left shunt fraction, e.g., through a PFO or IPAVA, would reveal exaggerated responses in mPAP and PVR to hypoxia or hyperoxia, for instance by acting as an escape valve mechanism. This is in line with retrospective studies in subjects with PH and PFO that did not show any association with mPAP or PVR (16–18). However, due to the retrospective nature of the present study, we cannot provide data on the PFO as we do not systematically screen patients with saline contrast echocardiography. As we only calculated Qs/Qt under hyperoxia, we cannot exclude the possibility that Qs/Qt might change depending on the current FiO2. Future approaches could include contrast echography under normoxia and hypoxia to detect potential changes in Qs/Qt at different FiO2 (38) or investigate whether therapies for pulmonary vascular disease would influence shunt fractions.
Influence of Age and Sex on Pulmonary Hemodynamics
Adjustment for age, sex, and PH disease classification (PAH vs. CTEPH) (2) in the multivariable regression model revealed that mPAP, PVR, and PAWP were independent of age and PH-classification. CI showed a negative association with age.
Limitations
Exposure to hypoxia and hyperoxia was only of short duration (at least 10 min) and further studies are required to investigate whether longer exposure would consistently change hemodynamics and whether different oxygen fractions used would result in hemodynamic or clinical improvements on long-term. We assumed that a washout period of at least 10 min between different FiO2 levels would suffice to avoid a significant carry-over effect of the interventions. Additionally, as all patients received the same order of FiO2 intervention (first hypoxia and then hyperoxia), we cannot exclude any order or time effects, which could potentially affect results. For physiological reasons, the Qs/Qt from blood gases can only be calculated under FiO2 1.0 and we do not know whether a herewith-calculated shunt fraction remains stable under different FiO2, an assumption that has been questioned (11, 19).
Conclusion
In this large cohort of patients with PH, acute exposure to hypoxia did not significantly alter mPAP or total pulmonary resistance, which indicates that HPV is blunted in patients with preexisting pulmonary vascular disease. Short-term pure oxygen breathing markedly reduced both mPAP and PVR and supports further exploration into the effect of long-term oxygen treatment on prognosis in pulmonary vascular disease. A large proportion of patients with PH reveal an increased Qs/Qt, which did not affect pulmonary hemodynamics under different FiO2.
Data Availability Statement
The raw data supporting the conclusions of this article will be made available by the authors, without undue reservation.
Ethics Statement
The studies involving human participants were reviewed and approved by Cantonal Ethics Committee of Zurich (No. 2020–01541). The patients/participants provided their written informed consent to participate in this study.
Author Contributions
SU is the guarantor and takes responsibility for the content of the manuscript, including the data and analysis. AC contributed to acquiring, analyzing, and interpreting the data, writing and revising the article critically for important intellectual content, and providing final approval of the version to be published. ML, CB, SS, ES, SRS, MF, and KB contributed to data collection and analysis and revising the article critically for important intellectual content. ML and SU conceived the project, contributed to data collection, analysis, interpretation, writing the manuscript, revising the article critically for important intellectual content, and providing final approval of the version to be published. All authors took responsibility for all aspects of the reliability and freedom from bias of the data presented and their discussed interpretation. All authors contributed to the article and approved the submitted version.
Conflict of Interest
SU reports grants from Johnson and Johnson SA, Switzerland, during the conduct of the study; grants from the Swiss National Science Foundation, grants from Zurich Lung, grants from Orpha Swiss, personal fees from Actelion SA, Switzerland, personal fees from MSD Switzerland, outside the submitted work.
The remaining authors declare that the research was conducted in the absence of any commercial or financial relationships that could be construed as a potential conflict of interest.
Publisher's Note
All claims expressed in this article are solely those of the authors and do not necessarily represent those of their affiliated organizations, or those of the publisher, the editors and the reviewers. Any product that may be evaluated in this article, or claim that may be made by its manufacturer, is not guaranteed or endorsed by the publisher.
Supplementary Material
The Supplementary Material for this article can be found online at: https://www.frontiersin.org/articles/10.3389/fmed.2022.791423/full#supplementary-material
Abbreviations
BMI, body mass index; BP, blood pressure; BSA, body surface area; CI, cardiac Index; CO, cardiac Output; DLCO, diffusing capacity of lung carbon monoxide; CTEPH, chronic thromboembolic pulmonary hypertension; FEV1:, forced expiration in the first second of expiration; FVC, forced vital capacity; FiO2, fraction of inspired oxygen; Hb, hemoglobin; HR, heart rate; IPAVA, intrapulmonary arteriovenous anastomosis; KCO, carbon monoxide transfer coefficient; mPAP, mean pulmonary artery pressure; PaCO2, arterial partial pressure of carbon dioxide; PAH, pulmonary arterial hypertension; PAO2, alveolar partial pressure of oxygen; PaO2, arterial partial pressure of oxygen; PAWP, pulmonary artery wedge pressure; PB, barometric pressure at the elevation of 490 m; PH2O, partial pressure of water at body temperature; PmvO2, mixed venous partial pressure of oxygen; PVR, pulmonary vascular resistance; Qs/Qt, fraction of right-to-left shunt; R, respiratory quotient; SaO2, arterial saturation of oxygen; ScapO2, pulmonary capillary oxygen saturation; SD, standard deviation; SmvO2, mixed venous saturation of oxygen; WHO, World Health Organization; WU, wood unit.
References
1. Sylvester JT, Shimoda LA, Aaronson PI, Ward JP. Hypoxic pulmonary vasoconstriction. Physiol Rev. (2012) 92:367–520. doi: 10.1152/physrev.00041.2010
2. Galiè N, Humbert M, Vachiery JL, Gibbs S, Lang I, Torbicki A, et al. 2015 ESC/ERS Guidelines for the diagnosis and treatment of pulmonary hypertension: the Joint Task Force for the Diagnosis and Treatment of Pulmonary Hypertension of the European Society of Cardiology (ESC) and the European Respiratory Society (ERS): Endorsed by: Association for European Paediatric and Congenital Cardiology (AEPC), International Society for Heart and Lung Transplantation (ISHLT). Eur Heart J. (2016) 37:67–119. doi: 10.1093/eurheartj/ehv317
3. Seccombe LM, Chow V, Zhao W, Lau EM, Rogers PG, Ng AC, et al. Right heart function during simulated altitude in patients with pulmonary arterial hypertension. Open Heart. (2017) 4:e000532. doi: 10.1136/openhrt-2016-000532
4. Ghofrani HA, Voswinckel R, Reichenberger F, Weissmann N, Schermuly RT, Seeger W, et al. Hypoxia- and non-hypoxia-related pulmonary hypertension - established and new therapies. Cardiovasc Res. (2006) 72:30–40. doi: 10.1016/j.cardiores.2006.07.025
5. Aldashev AA, Sarybaev AS, Sydykov AS, Kalmyrzaev BB, Kim EV, Mamanova LB, et al. Characterization of high-altitude pulmonary hypertension in the Kyrgyz: association with angiotensin-converting enzyme genotype. Am J Respir Crit Care Med. (2002) 166:1396–402. doi: 10.1164/rccm.200204-345OC
6. Groth A, Saxer S, Bader PR, Lichtblau M, Furian M, Schneider SR, et al. Acute hemodynamic changes by breathing hypoxic and hyperoxic gas mixtures in pulmonary arterial and chronic thromboembolic pulmonary hypertension. Int J Cardiol. (2018) 270:262–7. doi: 10.1016/j.ijcard.2018.05.127
7. Green S, Stuart D. Oxygen and pulmonary arterial hypertension: effects, mechanisms, and therapeutic benefits. Eur J Prev Cardiol. (2021) 28:127–36. doi: 10.1093/eurjpc/zwaa001
8. Leuchte HH, Baezner CJ, Baumgartner RA, Mernitz P, Neurohr C, Behr J. Acute hemodynamic responses to supplemental oxygen and their prognostic implications in pulmonary hypertension. Respiration. (2013) 85:400–7. doi: 10.1159/000340009
9. Ulrich S, Saxer S, Hasler ED, Schwarz EI, Schneider SR, Furian M, et al. Effect of domiciliary oxygen therapy on exercise capacity and quality of life in patients with pulmonary arterial or chronic thromboembolic pulmonary hypertension: a randomised, placebo-controlled trial. Eur Respir J. (2019) 54:2762019. doi: 10.1183/13993003.002762019
10. Ulrich S, Keusch S, Hildenbrand FF, Lo Cascio C, Huber LC, Tanner FC, et al. Effect of nocturnal oxygen and acetazolamide on exercise performance in patients with pre-capillary pulmonary hypertension and sleep-disturbed breathing: randomized, double-blind, cross-over trial. Eur Heart J. (2015) 36:615–23. doi: 10.1093/eurheartj/eht540
11. Gossage JR, Kanj G. Pulmonary arteriovenous malformations. A state of the art review. Am J Respir Crit Care Med. (1998) 158:643–61. doi: 10.1164/ajrccm.158.2.9711041
12. Petersson J, Glenny RW. Gas exchange and ventilation-perfusion relationships in the lung. Eur Respir J. (2014) 44:1023–41. doi: 10.1183/09031936.00037014
13. Hagen PT, Scholz DG, Edwards WD. Incidence and size of patent foramen ovale during the first 10 decades of life: an autopsy study of 965 normal hearts. Mayo Clin Proc. (1984) 59:17–20. doi: 10.1016/S0025-6196(12)60336-X
14. Meissner I, Whisnant JP, Khandheria BK, Spittell PC, O'Fallon WM, Pascoe RD, et al. Prevalence of potential risk factors for stroke assessed by transesophageal echocardiography and carotid ultrasonography: the SPARC study. Stroke Prev. (1999) 74:862–9. doi: 10.4065/74.9.862
15. Sharan L, Stackhouse K, Awerbach JD, Bashore TM, Krasuski RA. Effect of patent foramen ovale in patients with pulmonary hypertension. Am J Cardiol. (2018) 122:505–10. doi: 10.1016/j.amjcard.2018.04.014
16. Nootens MT, Berarducci LA, Kaufmann E, Devries S, Rich S. The prevalence and significance of a patent foramen ovale in pulmonary hypertension. Chest. (1993) 104:1673–5. doi: 10.1378/chest.104.6.1673
17. Vodoz JF, Cottin V, Glérant JC, Derumeaux G, Khouatra C, Blanchet AS, et al. Right-to-left shunt with hypoxemia in pulmonary hypertension. BMC Cardiovasc Disord. (2009) 9:15. doi: 10.1186/1471-2261-9-15
18. Gallo de Moraes A, Vakil A, Moua T. Patent foramen ovale in idiopathic pulmonary arterial hypertension: Long-term risk and morbidity. Respir Med. (2016) 118:53–7. doi: 10.1016/j.rmed.2016.07.007
19. Lovering AT, Romer LM, Haverkamp HC, Pegelow DF, Hokanson JS, Eldridge MW. Intrapulmonary shunting and pulmonary gas exchange during normoxic and hypoxic exercise in healthy humans. J Appl Physiol. (2008) 104:1418–25. doi: 10.1152/japplphysiol.00208.2007
20. Simonneau G, Montani D, Celermajer DS, Denton CP, Gatzoulis MA, Krowka M, et al. Haemodynamic definitions and updated clinical classification of pulmonary hypertension. Eur Respir J. (2019) 53:1801913. doi: 10.1183/13993003.01913-2018
21. Maron BA, Brittan EL, Hess E, Waldo SW, Baron AE, Huang S, et al. Pulmonary vascular resistance and clinical outcomes in patients with pulmonary hypertension: a retrospective cohort study. Lancet Respir Med. (2020) 8:873–84. doi: 10.1016/S2213-2600(20)30317-9
22. Xanthouli P, Jordan S, Milde N, Marra A, Blank N, Egenlauf B, et al. Haemodynamic phenotypes and survival in patients with systemic sclerosis: the impact of the new definition of pulmonary arterial hypertension. Ann Rheum Dis. (2020) 79:370–8. doi: 10.1136/annrheumdis-2019-216476
23. Hasler ED, Müller-Mottet S, Furian M, Saxer S, Huber LC, Maggiorini M, et al. Pressure-flow during exercise catheterization predicts survival in pulmonary hypertension. Chest. (2016) 150:57–67. doi: 10.1016/j.chest.2016.02.634
24. Kovacs G, Avian A, Olschewski A, Olschewski H. Zero reference level for right heart catheterisation. Eur Respir J. (2013) 42:1586–94. doi: 10.1183/09031936.00050713
25. Kovacs G, Avian A, Pienn M, Naeije R, Olschewski H. Reading pulmonary vascular pressure tracings. How to handle the problems of zero leveling and respiratory swings. Am J Respir Crit Care Med. (2014) 190:252–7. doi: 10.1164/rccm.201402-0269PP
26. Ryan JJ, Rich JD, Thiruvoipati T, Swamy R, Kim GH, Rich S. Current practice for determining pulmonary capillary wedge pressure predisposes to serious errors in the classification of patients with pulmonary hypertension. Am Heart J. (2012) 163:589–94. doi: 10.1016/j.ahj.2012.01.024
27. Ghofrani HA, Galie N, Grimminger F, Grunig E, Humbert M, Jing ZC, et al. Riociguat for the treatment of pulmonary arterial hypertension. N Engl J Med. (2013) 369:330–40. doi: 10.1056/NEJMoa1209655
28. Milger K, Felix JF, Voswinckel R, Sommer N, Franco OH, Grimminger F, et al. Sildenafil versus nitric oxide for acute vasodilator testing in pulmonary arterial hypertension. Pulm Circ. (2015) 5:305–12. doi: 10.1086/680355
29. Hilty MP, Müller A, Flück D, Siebenmann C, Rasmussen P, Keiser S, et al. Effect of increased blood flow on pulmonary circulation before and during high altitude acclimatization. High Alt Med Biol. (2016) 17:305–14. doi: 10.1089/ham.2016.0004
30. Maggiorini M, Léon-Velarde Léon-Velarde F. High-altitude pulmonary hypertension: a pathophysiological entity to different diseases. Eur Respir J. (2003) 22:1019–25. doi: 10.1183/09031936.03.00052403
31. Bärtsch P, Mairbäurl H, Maggiorini M, Swenson ER. Physiological aspects of high-altitude pulmonary edema. J Appl Physiol. (2005) 98:1101–10. doi: 10.1152/japplphysiol.01167.2004
32. Dehnert C, Mereles D, Greiner S, Albers D, Scheurlen F, Zügel S, et al. Exaggerated hypoxic pulmonary vasoconstriction without susceptibility to high altitude pulmonary edema. High Alt Med Biol. (2015) 16:11–7. doi: 10.1089/ham.2014.1117
33. Dunham-Snary KJ, Wu D, Sykes EA, Thakrar A, Parlow LRG, Mewburn JD, et al. Hypoxic pulmonary vasoconstriction: from molecular mechanisms to medicine. Chest. (2017) 151:181–92. doi: 10.1016/j.chest.2016.09.001
34. Marshall C, Marshall B. Site and sensitivity for stimulation of hypoxic pulmonary vasoconstriction. J Appl Physiol. (1983) 55:711–6. doi: 10.1152/jappl.1983.55.3.711
35. Ulrich S, Schneider SR, Bloch KE. Effect of hypoxia and hyperoxia on exercise performance in healthy individuals and in patients with pulmonary hypertension: a systematic review. J Appl Physiol. (2017) 123:1657–70. doi: 10.1152/japplphysiol.00186.2017
36. Lang IM, Dorfmüller P, Vonk Noordegraaf A. The pathobiology of chronic thromboembolic pulmonary hypertension. Ann Am Thorac Soc. (2016) 13(Suppl 3):S215–21. doi: 10.1513/AnnalsATS.201509-620AS
37. Humbert M. Pulmonary arterial hypertension and chronic thromboembolic pulmonary hypertension: pathophysiology. Eur Respir Rev. (2010) 19:59–63. doi: 10.1183/09059180.00007309
Keywords: pulmonary arterial hypertension (PAH), chronic thromboembolic pulmonary arterial hypertension (CTEPH), right heart catheterization, oxygen, hypoxia, right to left shunting
Citation: Carta AF, Lichtblau M, Berlier C, Saxer S, Schneider SR, Schwarz EI, Furian M, Bloch KE and Ulrich S (2022) The Impact of Breathing Hypoxic Gas and Oxygen on Pulmonary Hemodynamics in Patients With Pulmonary Hypertension. Front. Med. 9:791423. doi: 10.3389/fmed.2022.791423
Received: 08 October 2021; Accepted: 10 January 2022;
Published: 11 February 2022.
Edited by:
Christophe Guignabert, Institut National de la Santé et de la Recherche Médicale (INSERM), FranceReviewed by:
Laurent Savale, Bicêtre Hospital, FranceAlys Clark, The University of Auckland, New Zealand
Karin Boomars, Erasmus Medical Center, Netherlands
Copyright © 2022 Carta, Lichtblau, Berlier, Saxer, Schneider, Schwarz, Furian, Bloch and Ulrich. This is an open-access article distributed under the terms of the Creative Commons Attribution License (CC BY). The use, distribution or reproduction in other forums is permitted, provided the original author(s) and the copyright owner(s) are credited and that the original publication in this journal is cited, in accordance with accepted academic practice. No use, distribution or reproduction is permitted which does not comply with these terms.
*Correspondence: Silvia Ulrich, c2lsdmlhLnVscmljaCYjeDAwMDQwO3Vzei5jaA==; dWxyaXMmI3gwMDA0MDtibHVld2luLmNo