- 1Department of Urology, The Second Hospital of Anhui Medical University, Hefei, Anhui, China
- 2Peking University Shenzhen Hospital, Shenzhen, China
Three-dimensional (3D) printing technology involves the application of digital models to create 3D objects. It is used in construction and manufacturing and has gradually spread to medical applications, such as implants, drug development, medical devices, prosthetic limbs, and in vitro models. The application of 3D printing has great prospects for development in orthopedics, maxillofacial plastic surgery, cardiovascular conditions, liver disease, and other fields. With in-depth research on 3D printing technology and the continuous update of printing materials, this technology also shows broad development prospects in renal medicine. In this paper, the author mainly summarizes the basic theory of 3D printing technology, its research progress, application status, and development prospect in renal diseases.
Introduction
Three-dimensional (3D) printing technology builds special materials into established models by printing several times based on 3D digital models (1). It originated in 1986 and expanded to the construction and manufacturing industries in the 1990s. In recent years, it has played an increasingly important role in aerospace (2), experimental research (2), medicine and medical treatment (3), and other fields. In the medical field, 3D printing technology is primarily a process of transforming medical images, including computed tomography (CT) (4), magnetic resonance imaging (MRI) (5), and ultrasound (6), into solid models. This is also known as additive manufacturing (AM) or rapid prototyping (RP). It creates 3D objects from stereolithography (STL) files through layer-by-layer continuous superposition using various technologies. In recent years, many countries are attempting to establish a 3D printing industry and apply this technology to various fields, including business, mechanical engineering, and medicine (7). It has great applicability and development prospects in medical fields, such as implant and anatomical models, tissue and organ manufacture, and prosthesis customization (8).
In the last decade, research on 3D printing in medical fields has grown dramatically, covering various aspects such as orthopedics (9, 10), plastic surgery (11), thoracic surgery, cardiac surgery (12, 13), tissue engineering of medical devices, and drug research. Its research and application in urology have also increased significantly and have become a focus of attention and research in the field of urology, especially nephrology (14). Previously, we could only perform preoperative analysis and design of urology surgery through X-ray, intravenous pyelography, CT, and other imaging data, but these imaging data are not as intuitive in reflecting the severity of the lesion site and surrounding tissues and anatomical malformations as they are in the intraoperative eye view. Therefore, surgical success often depends on the surgeon’s exploration of intraoperative lesions and rich clinical practice experience. If the preoperative and intraoperative judgment of the lesion location is inaccurate, it may directly affect the therapeutic effect, safety, and success rate of the operation. At present, 3D printing technology can directly and accurately print the lesion model and anatomical structure of the surgical area according to the preoperative imaging data of patients, such as MRI and CT, and provide patients with customized high-precision surgical plans and implants in the field of surgery. In this way, the success rate of complicated surgical procedures can be improved, more accurate surgical plans can be made, possible intraoperative risks can be assessed in advance, and intraoperative emergency plans can be made. Surgeons can also use it for surgical planning and training in a physical model. Finally, it can make the operation easier and more mature, shorten the operation time, and reduce the risk and failure rates. The maturity and development of 3D printing technology have brought good news to patients with kidney diseases. In view of this, the author reviewed the application of 3D printing technology in renal medicine and summarized its application status and prospects in renal surgery combined with a literature review.
Technology base
3D printing is a rapid prototyping technology, and a new digital prototyping technology based on computer digital model files. According to the principle of “layered manufacturing, layer-by-layer stacking,” adhesive materials such as powder, metal, and polymer plastic can be accurately printed and stacked layer-by-layer through a 3D printing system to obtain arbitrary complex shaped products (15). Currently, the manufacturing process of 3D printing technology in medicine mainly includes the following steps: (1) The tissue to be printed is examined by CT or MRI, and the data extracted from the imaging files are saved in Digital Imaging and Communications in Medicine format. (2) The sectional image is segmented by Mimics and other software processing systems, and then the printed tissue is extracted by the segmentation method of threshold and region growth. Finally, it is processed by Laplacian smoothing and transmitted to a 3D printer in the STL format. (3) After the 3D printer completes the model printing, it requires 4–6 h to make the model dry and stable. (4) The specific substance completely covers the surface of the model for easy absorption by the model. Model penetration avoids adhesion and poor elasticity between adjacent tissues (4). Compared to two-dimensional (2D) imaging, 3D models can measure anatomical structures more accurately. The resolution of 3D printing technology in horizontal and vertical directions can reach 0.01 and 0.2 mm, which is in line with the resolution of the original data. Therefore, 3D printing technology can meet the requirements of human anatomical structure printing. A basic flowchart of the 3D printing model design is shown in Figure 1.
3D printing technologies can be classified according to the type of technology and material used. Specialized techniques include stereolithography apparatus (SLA), multijet printing, selective laser sintering (SLS), direct metal laser sintering (DMLS), fused deposition modeling (FDM), and so on (3). The material classification includes thermoplastic polymer materials, metals, ceramics, photopolymers, paper, foils, plastic films, titanium alloys, and biological materials (3). In medicine, various kinds of 3D printing can be divided into four types according to their applications: (1) For the preoperative model, only the anatomical structure of the lesion and its adjacent relationship with the surrounding area need to be visually displayed, and the biological properties of the material are not high. SLA and FDM are generally used, such as 3D models of liver cancer (16) and renal carcinoma (17). (2) Personalized internal implants require biocompatible materials and the mechanical properties of the printed structure. SLS and DMLS are used as bone tissue scaffolds (18) and personalized vertebral prostheses (19). (3) Tissue engineering scaffolds require printing materials with good biocompatibility and biodegradability, which can be used for FDM and 3D bioprinting, such as vascular scaffolds (20). (4) For cellular structures or tissue-like organs, scaffolds and cells must be printed at the same time, and the operating environment has high requirements; therefore, 3D bioprinting is adopted, such as cartilage tissue-like structures (21) and tissue units (22).
Preoperative communication and education
In recent years, more 3D printed models have been used for preoperative doctor-patient communication and education, as shown in Figure 2, helping patients and their families to understand the condition and surgery from many aspects and achieve better doctor-patient communication. For example, the University of Southern California urology Institute has completed a doctor-patient communication study on a 3D-printed renal carcinoma model; their research found that the communication effect of the 3D-printed renal carcinoma model was significantly better than a simple text explanation (23). Similarly, Zhang et al. (24) also conducted a doctor-patient communication evaluation study using a 3D-printed renal carcinoma model, proving that the model is conducive to understanding the diagnosis and treatment process by patients and their families. The 3D model recreated the entire lesion organ and its adjacent structures. It facilitates doctor-patient communication and enables patients and their families to understand the entire treatment process. More importantly, it is conducive to active cooperation in the treatment and follow-up care after discharge.
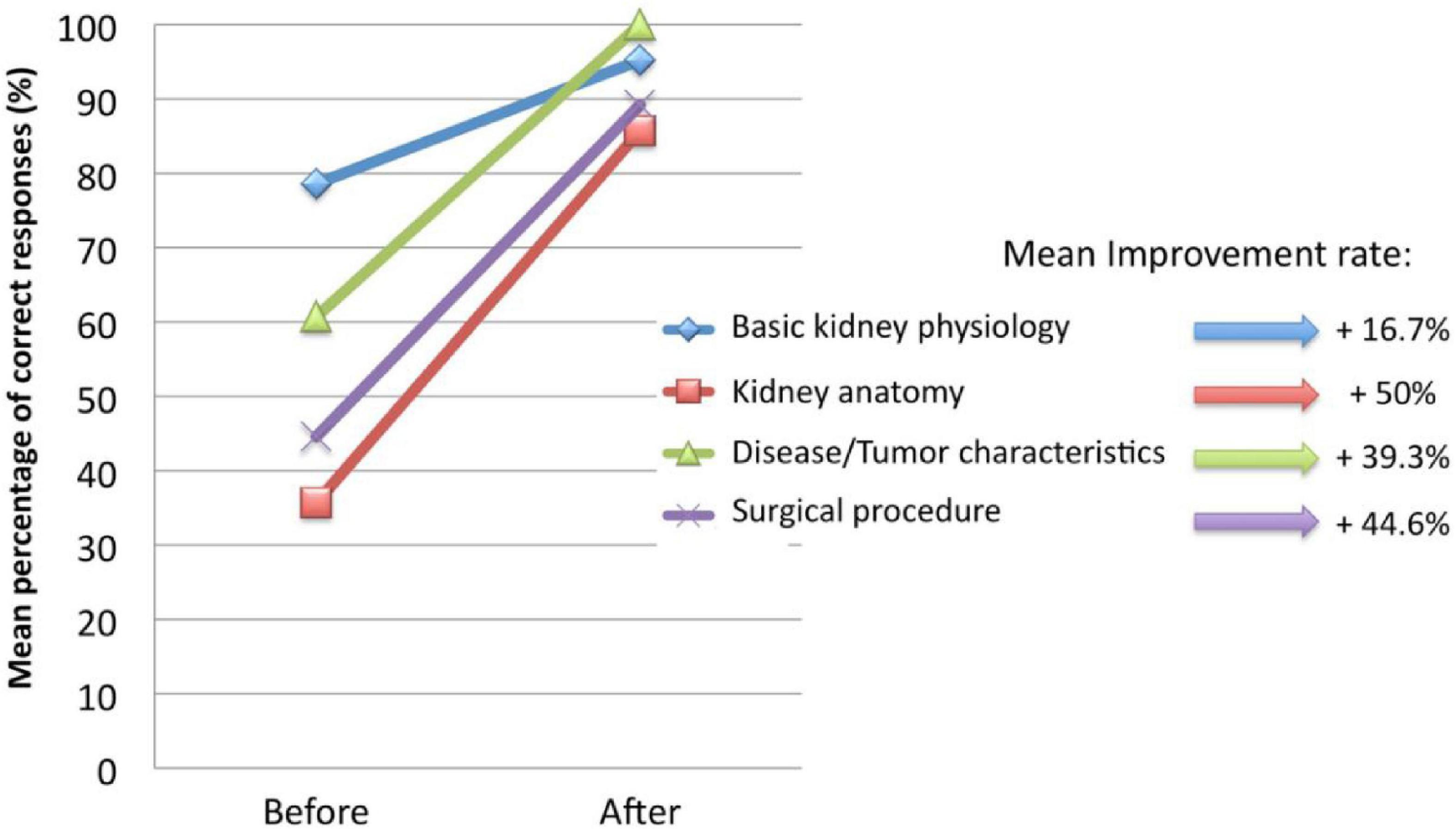
Figure 2. Patients’ understanding changes in several aspects before and after using the 3D printing model (23).
Clinical medicine has strong practicality and applications. The perfection of the teaching system directly affects the cultivation of high-quality talents. Traditional clinical teaching methods are relatively simple, abstract, and lack visualization. Although some multimedia teaching can meet students’ visual learning needs, it cannot achieve hands-on practice, and it is impossible to have enough patients for students to learn and operate clinically. Therefore, many young physicians lack the tactile and 3D anatomical experience that surgery can provide. 3D printing model perfectly compensates for this defect. It can display 2D images in the form of objective 3D objects and provide 3D tactile experience and simulate surgical operations, which is of great positive significance for the technical maturity of young physicians and medical students. Compared with 2D scans, the benefits of 3D-printed models for educating patients, medical students, and physicians have been initially demonstrated. Knoedler et al. (25) asked first-year medical students to complete the RENAL nephrometry score according to CT and 3D models and compared the results. The experimental results showed that the 3D model significantly improved the trainees’ renal function scoring accuracy. It showed consistency among trainees and less difference than expert surgeons, indicating that the 3D model enhanced students’ understanding and familiarity with kidney diseases. The 3D printing technology can be individualized for different cases, and the model can clearly reflect the lesion site and adjacent anatomical relationships. More importantly, it can simulate a real surgical field completely. Using this individualized model in teaching can make students more intuitive in understanding the disease and more skilled in related operations, thereby greatly improving the quality of teaching. Of course, 3D printing also has some limitations in clinical communication and teaching. This new approach is an unprecedented challenge for physicians, patients, and medical students. It not only requires doctors to conduct more in-depth analysis and explanation based on the actual model, but also requires medical students and patients to adapt and cooperate with this new method. We believe that good interactive communication and a certain ability to understand 3D space are the keys to the success of 3D printing in communication and education.
Medical equipment and surgical implants
The development of 3D-printed medical devices in urology is not as advanced as that in orthopedics, and it is still in the research and development stage; however, some progress has also been made in recent years. George et al. (26) used 3D printing to manufacture hemostatic forceps, needle drivers, scalpel handles, retractors, and forceps and asked urologists to conduct simulated surgical evaluations and constantly adjust the design and improve the method. Del et al. (27) used 3D printing technology to produce personalized ureteral stents and performed perfusion experiments with traditional standard stents in an isolated pig urinary system. They then performed statistical analyses of the total, extraluminal, and intraluminal flows. In conclusion, the effect of 3D-printed ureteral stents was comparable to that of standard stents. We believe that with the continuous upgrading of technology, the application of ureteral stents tailored to patient anatomical characteristics is not far away in clinical practice. Park et al. (28) reported a more active mindset. Based on Del et al.’s report, they used 3D printing to produce a new type of ureteral stent with a polymeric flap to protect against reflux, as shown in Figure 3. The results of the in vitro experiments showed that this new type of stent effectively prevented the reverse flow of urine and minimized the reduction in forward flow. The technology of 3D printing surgical instruments and implants is not yet mature, and there are difficulties, such as material selection, material mixing ratio, and printing time control. To date, this technology needs to be explored and researched. In addition, whether it is medical equipment or implants, most current studies focus on effectiveness and lack safety evaluation (27). We believe that as more human and animal experiments are carried out, the practicability and safety of 3D printing in this aspect will be further confirmed.
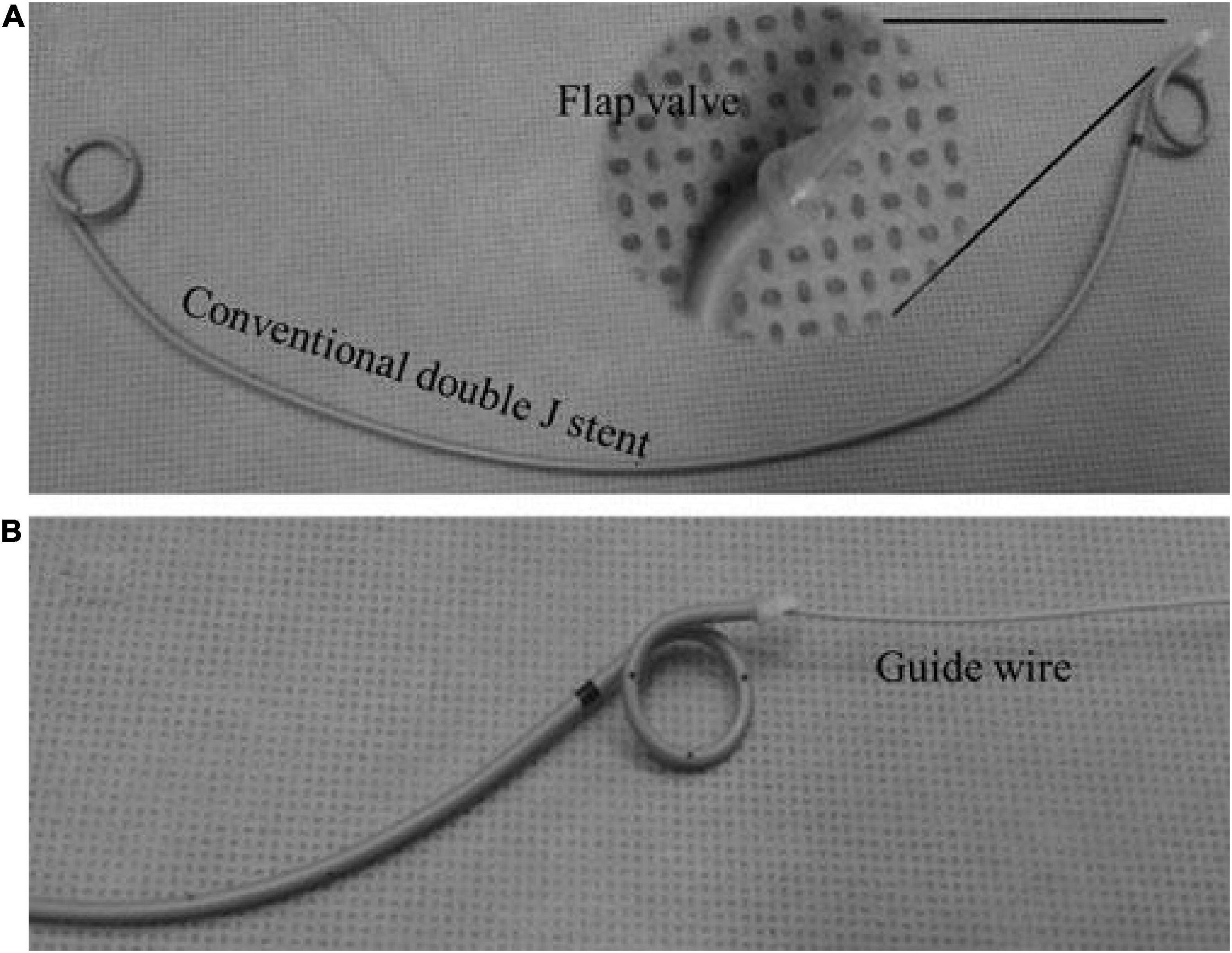
Figure 3. Panel (A) shows an anti-reflux ureteral stent, and panel (B) shows it attached to a guide wire (28).
Renal carcinoma
A clear understanding of renal tumors and their relationship to neighboring structures is critical to developing surgical strategies and outcomes, and the 3D printing of renal carcinoma models can well help physicians to achieve this goal (25). A specific case is illustrated in Figure 4. As shown in the figure, the 3D model can comprehensively show the location, size, relationship with the collecting system, and distribution of the blood vessels of the tumor. These models, combined with CT and other related imaging data, help the surgeon make the best decision on the surgical approach and accurately resect the lesion and preserve the nephron as much as possible. With technological advancement, 3D printing will provide sufficient technical support for partial nephrectomy in the future, reduce technical difficulties, and promote this surgical method, so that more patients can receive effective treatment.
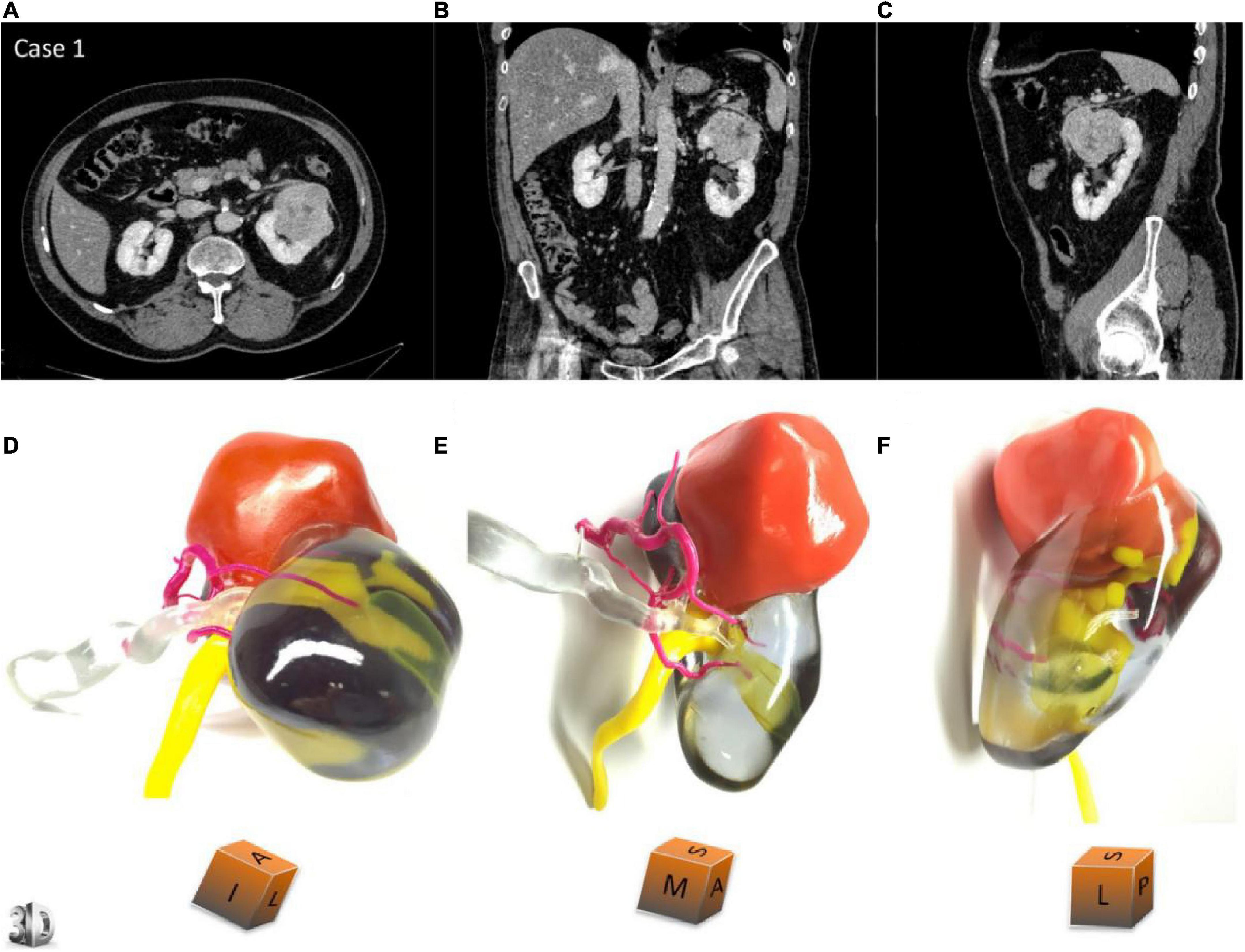
Figure 4. Panels (A–C) show the transverse section, coronal plane and sagittal plane of the patient’s kidney during the development phase of CT. Panels (D–F) are the corresponding 3D printing models, respectively (23).
There are many applications of 3D printing technology in renal carcinomas. Komai et al. (29) successfully performed a minimally invasive partial nephrectomy using a 3D-printed kidney model. The results indicated that the tumor and margin of the surgically resected specimens were almost identical to those of the 3D printed model, demonstrating the feasibility of 3D printing technology for preoperative planning and intraoperative navigation of partial nephrectomy. Wake et al. (5) studied the influence of 3D printing model on preoperative planning of surgery for the complex renal carcinoma. The outcomes proved that compared with the 2D image data, the operation plan made by the surgeon according to the 3D model had a high degree of matching with the actual operation. The 3D model helped us refine the preoperative plan and preserve as many nephrons as possible during the operation. Bernhard et al. (23) printed seven renal carcinoma models for preoperative planning and treatment notification. Through their research, the patients’ cognition was improved, and it also provided some tips and help for doctors to perform renal carcinoma surgery to a certain extent. Many studies have shown that the 3D kidney model is helpful in accurately locating tumors and reducing surgical risks and complications, which is worthy of clinical application. On the other hand, we also see some shortcomings in the application of 3D printing in renal carcinoma. Considering the physiological complexity of tumor tissue, the printed model of kidney cancer lacks the texture and hemodynamic characteristics of the natural organ, which may not fully reflect tumor details. This can lead to some errors and deviations in the preoperative plan. With the development of our understanding of cancer and kidney, it is reasonable to believe that these limitations will be optimized and avoided in the near future.
Renal calculus
Percutaneous nephrolithotomy is an important treatment method for complex renal calculi. The design and establishment of the percutaneous nephrolithotomy puncture channel are key to the treatment outcomes. We usually judge the general situation of calculi according to preoperative CT and intraoperative B-ultrasound and then establish the channel in combination with clinical experience. Usually, because surgeons do not intuitively and accurately understand the anatomical relationship between perirenal organs, renal vascular distribution, and stone location, it is difficult for them to find the optimal puncture channel, leading to a high incidence of complications such as bleeding, adjacent organ injury, and urinary sepsis (30). However, with the application of 3D printing technology in renal calculus diseases, preoperative stone models can help us understand the shape of calyces and the details of calculi more comprehensively. This is helpful for doctors to choose the best puncture channel before surgery and to improve the accuracy of puncture to reduce surgery-related complications.
Relevant studies have demonstrated the importance of 3D printing in treating renal calculi. Kang et al. (31) reported that the specific location of renal calculi could be observed using 3D printing technology. The surgeons planned and simulated the operation on the 3D printed model to make the operation more accurate. They could avoid blood vessels, quickly locate the stone, and treat it accurately. According to one study, 3D printing technology has significantly improved the success rate of surgery by avoiding unnecessary surgical procedures and significantly reducing the operation time. Orecchia et al. (32) developed a series of 3D printed models of upper urinary tract and stones to train retrograde intrarenal surgery for renal calculus disease. The models they designed have the advantages of anatomical similarity, low cost and repeatability. What’s more, it can improve surgical skills in a risk-free environment. This reduces the number of cases required for doctors to achieve surgical proficiency while ensuring patient safety to some extent. Xu et al. (33) printed three models for these 12 patients included in the trial and selected three puncture points from the kidney’s upper, middle, and lower calyx to simulate percutaneous nephrolithotomy. They recorded the stone clearance rate and used the puncture site that yielded the highest stone clearance rate in the actual surgery. The results showed a high consistency and correlation between the postoperative calculus volume of patients and the models. It can be seen that a 3D printing model and in vitro simulation technology can help us select calyces and establish the best renal puncture channel in the treatment of complex calculi. Successful cases using 3D printing technology in the field of renal calculi are shown in Figure 5. The printed model provides more favorable information for preoperative planning, surgical simulation, and preoperative stone location, as well as support for selecting the best puncture point and angle to guarantee surgical success. While there are many advantages, we should also see some limitations of 3D printing in the application of renal calculus. The position of renal calculus in the human body is not static even in a short time, so the specific situation of the stone shown by 3D printing may not be exactly the same as what is seen during surgery. Scheduling surgery as soon as possible may be one way to solve this problem. In addition, it is also a problem to consider whether different parts and different components of stones need to be represented by different printing materials. All in all, 3D printing technology undoubtedly plays an important role in the diagnosis and treatment of renal calculus, and its development prospects are quite broad as well.
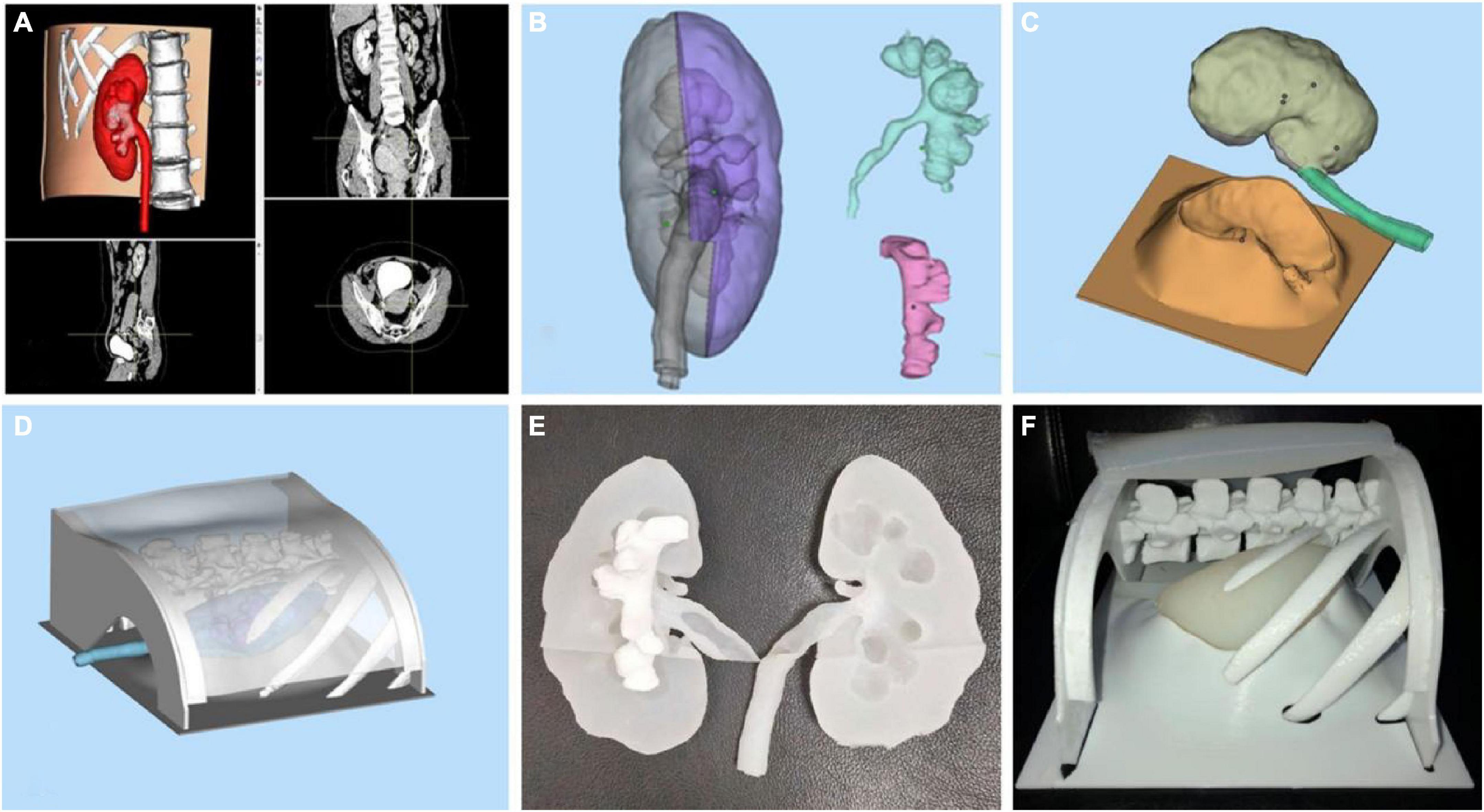
Figure 5. The 3D-printed model of kidney stones. Panel (A) is the software modeling. Panels (B–D) are the model constructions before printing. Panels (E,F) are 3D printed models of kidney related structures and stones (33).
Renal transplantation
Surgeons have difficulty performing vascular anastomosis during renal transplantation in patients with renal failure due to renal disease and other risk factors. Preoperative imaging also makes it very difficult to evaluate numerous vascular calcification foci, posing great obstacles to renal transplantation. The current international supply of renal transplants is far from meeting demand, and with the development of 3D printing in renal diseases, we can save several lives without the need for donors. We believe this will completely free patients with chronic renal insufficiency from dependence on organ donors and hope for renal transplantation.
At present, the research of 3D printing in renal transplantation is well underway. Denizet et al. (34) reported that 3D printing technology can reduce the operation time and the incidence of complications at the anastomotic site, and provide help for surgeons in renal transplantation. Chandak et al. (35) pointed out in their study that for children with end-stage renal disease, it is difficult to transplant adult-sized living donor kidneys into children. Three children with unique complex surgical anatomy received living kidney transplants using 3D-printed models. In this experiment, the 3D printing model enhanced the preoperative review and surgical simulation, and all the surgeries were successfully completed. At the most recent follow-up (>16 months), all patients had good renal transplant function. With the aggravation of the aging of society, the number of patients with chronic renal insufficiency is increasing day by day, and the demand for renal transplantation is increasing naturally. The number of organ donations has remained stable or decreased slightly, resulting in a shortage of kidneys. As shown in Figure 6, 3D printing offers hope for kidney transplants by printing functional tissues and even organs (36). However, the kidney generated by 3D printing is still faced with some problems and limitations in the real application of renal transplantation, such as whether the materials used will cause rejection, whether the printed kidney structure is highly compatible with the body. This requires not only a breakthrough in the development of biological materials, but also a deep understanding of the renal anatomy and microenvironment. The 3D-printed tissues and organs have not yet been used in the clinic, but we believe that with the development of related technology, we will be able to reap the fruits of this technological progress.
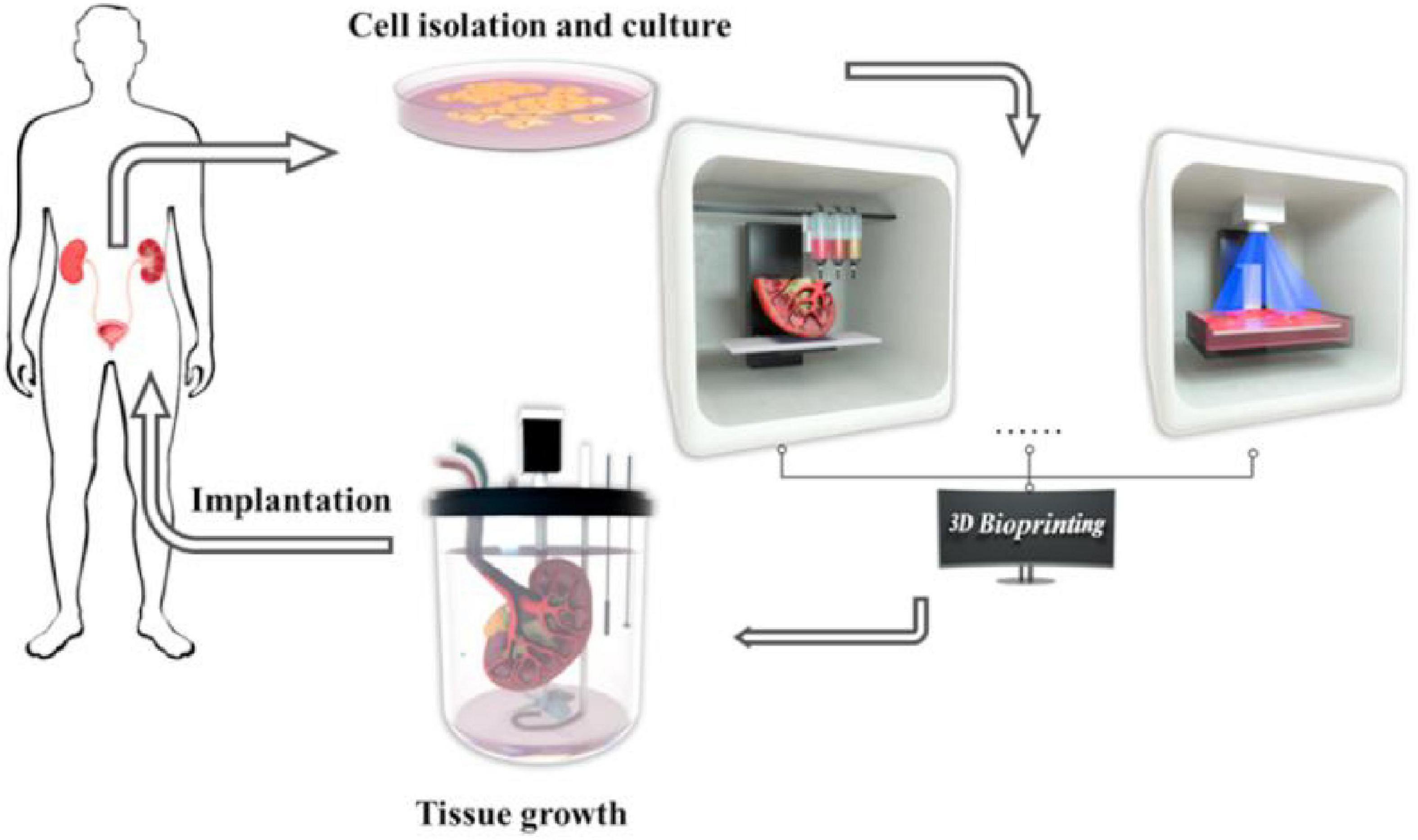
Figure 6. Tissue engineering and three-dimensional (3D) bioprinting technologies for urological diseases (36).
3D bio-printing
With the development of technology and the update of materials, the combination of various biological components and 3D printing technology produces an emerging technology, called 3D bio-printing technology (37, 38). It successfully processes cell-filled bio-inks into tissues and organs with the advantages of automation, high precision, printing adaptability, reproducibility and repeatability on a wide range of materials (37, 38). We believe that it will have broad applications in disease modeling (39), organ-on-a-chip (40), drug discovery and testing (41), high-throughput screening (42), and regenerative medicine (43).
3D bio-printing technology has a wide range of applications in the construction of functional tissues, including multi-layer skin (44), bone (45), vascular grafts (46), trachea (47), myocardial tissue (48), and cartilage structures (49). They can replace damaged or diseased tissues to meet the need for suitable tissues and organs for transplantation. Organ transplantation is currently the best treatment for ESRD; however, the number of existing kidney supplies is far from meeting the increasing demands of the ESRD population. Organovo created the world’s first whole-cell kidney tissue using 3D bio-printing. King et al. (41) used 3D bio-printing technology to create a 3D proximal tubule tissue model in vitro, as shown in Figure 7. Their research has undoubtedly opened the door to kidney regeneration and organ transplantation. 3D bio-printing of kidney organs is a new technology aimed at developing and printing organs with kidney function (50). In the near future, the emerging technology is expected to be combined with specific biological materials and tissue regeneration engineering to print kidney organs with normal physiological function, thereby improving the current situation of kidney organ shortage and the quality of life.
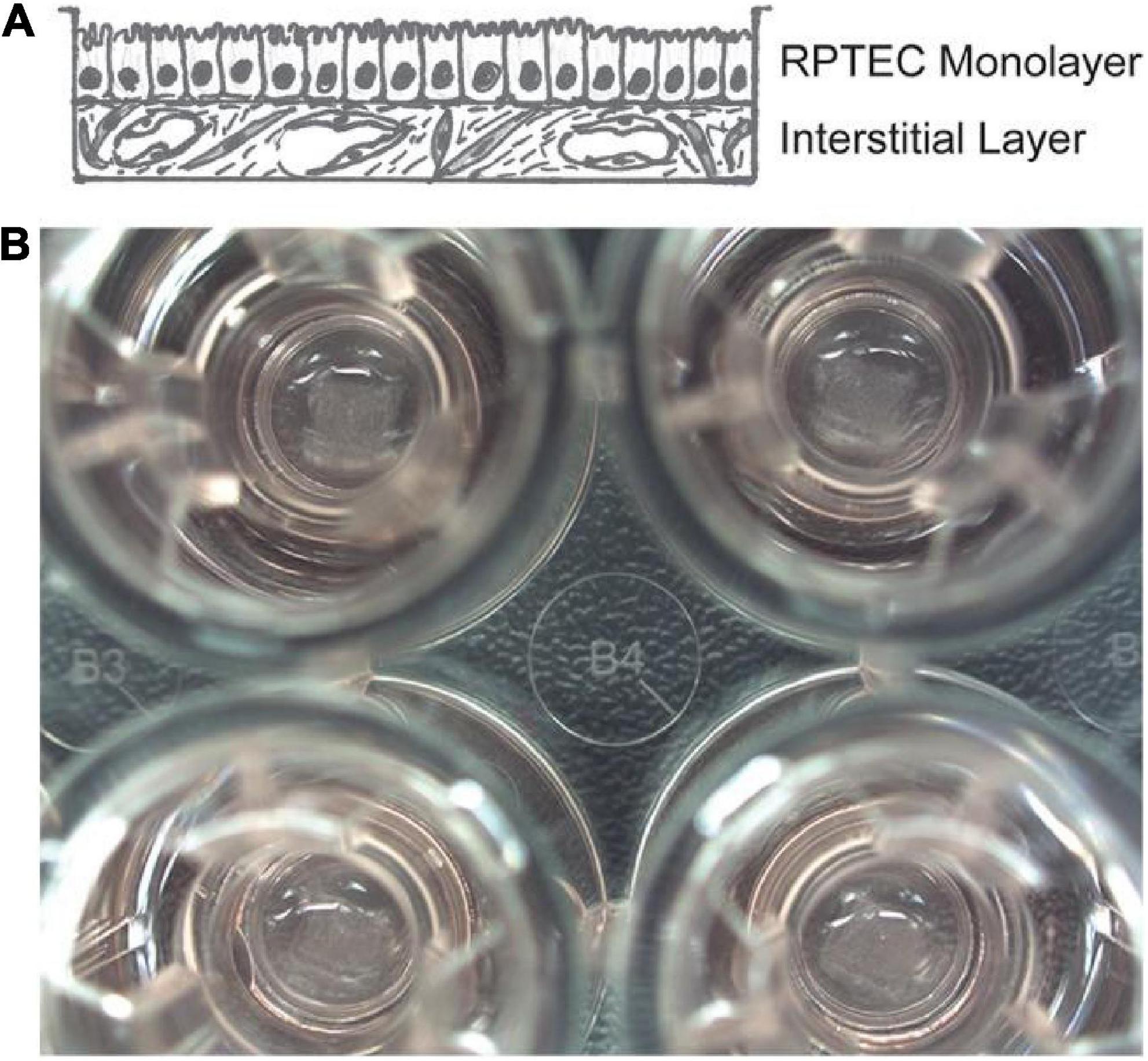
Figure 7. The 3D model of the interstitial interface of the kidney tubule using 3D bio-printing. Panel (A) is a hand-drawn view of the supporting epithelial and multicellular interstitial layers. Panel (B) is a macro view of the 3D bio-printed proximal tubule tissue in the Transwell experiment on a 24-well plate (41).
Discussion
Some important research and application progress of 3D printing technology in nephrology are summarized in Table 1. The application of 3D printing technology in renal diseases is still limited. Relevant research and applications have not been conducted in the human body on a large scale, and the development prospects are quite broad.
Although the application time of medical 3D printing technology in drug development and nephrotoxic drug screening is relatively short, its technological advantages and application potential are likely to play a significant role in promoting its development in these fields. 3D printing technology is widely used in the development of drug delivery systems (53), such as controlled-release (54) and sustained-release (55) drugs. Its application is to change the drug release mode and dosage form to apply the minimum drug dose to achieve an effective blood concentration and reduce drugs’ systemic toxic side effects. Cyclosporine A (CsA) is an immunosuppressive agent. Song et al. (56) successfully printed a drug carrier loaded with a CsA polymeric microsphere gel and equipped it with a 3D-printed anti-stress framework, effectively inhibiting animal immune rejection for 4 weeks. It can achieve the purpose of local, continuous, and effective medication, effectively treating the primary disease and reducing the drug dosage and potential adverse effects. This study is currently limited to animal experiments, and the selection of drug excipients in the printing process is limited (57). A series of issues, such as effectively controlling drug release and drug release time, require further study. The pathogenesis of glomerular-related diseases is closely associated with abnormal immune responses. Currently, glucocorticoids and immunosuppressants are the mainstay drugs; however, they have many side effects. Therefore, it is very important to reduce drug dosage and blood concentration. 3D printing technology still has many limitations and deficiencies in drug research. First of all, 3D printing technology for individualized manufacturing according to patients is not very consistent with the need for mass production of drugs (53). Secondly, drugs based on 3D-printed kidney models are undoubtedly more expensive, which also limits their development. We believe that with the optimization of material selection and the progress of printing technology, these problems can be solved to some extent. Although there are many problems to be solved in the application of 3D print-loaded multi-polymer drugs for the treatment of renal diseases, 3D printing technology is still expected to bring about a revolution in drug applications in the direction of renal drug development.
Traditional kidney microarray (58) is a technology used to study the effect of drugs on renal cell function in vitro using a 2D model with a single-layer microfluidic system of renal proximal tubular epithelial cells. This technique can only study the effects of drugs on renal proximal tubular cells. The research system lacks the physiological characteristics of a normal renal tissue 3D structure, which cannot fully reflect the physiological microenvironment of the human body and lacks objectivity for drug screening and toxicity tests (59, 60). Early 3D in vitro perfusion models (61) required highly specialized manufacturing techniques, which limited their routine application. The combination of renal cell chip technology and submillimeter 3D printing technology extends the structure of the renal function chip system to three dimensions. This can make it closer to normal human physiological kidney function, which is more conducive to the accurate and timely detection of drugs with deleterious effects on the kidney. Homan et al. (62) reproduced a microenvironment similar to the in vivo phenotype and function of human proximal renal tubular cells through 3D bioprinting and 3D cell culture combined with organ chip manufacturing technology. This type of kidney chip made by 3D technology can be applied to the drug preclinical trial stage, improving the screening rate and prediction ability of drugs with harmful potentials to the kidneys and avoiding animal experiments. Moreover, it can significantly boost new drug development by reducing the cost of developing new, safer drugs. Although research has only been done on a chip, it has opened up a new avenue for biological 3D printing of kidney tissue structures.
In conclusion, 3D printing technology has gradually gained attention for treating renal diseases. It has shown great value in medical staff training, patient education, surgical planning, and preoperative simulation. However, development prospects in drug research and transplantation are also exciting. Of course, there are some limitations to this technique, especially for patients with chronic kidney disease or allergies to contrast media that make imaging modeling difficult. In addition, when we do 3D print modeling using the traditional methods of native CT scanning, manually delineating the area of interest is often time consuming and not always reasonable. Solutions to these drawbacks are also prospects for future development. Although 3D printing has many shortcomings, such as material, cost, lack of professional personnel, limitations of imaging technology, and patient factors, it will continue to be improved with the development of science and technology, and it is expected to achieve comprehensive individualized treatment of 3D models. In addition, 3D bioprinting using tissue cells as materials will be an epoch-making innovation in the field of renal medicine. We believe that printing cells, tissues, and organs and successfully transplanting them into the human body will contribute to the development of medical science from the perspective of precision medicine.
Author contributions
LB proposed the idea and general direction of this research. SD wrote the manuscript with the advice and help of other authors. All authors contributed to the article and approved the submitted version.
Funding
The related research work in this manuscript was funded by the Research Fund of Anhui Institute of Translational Medicine under grant (2021zhyx-C62); National Natural Science Foundation of China under grant (81572107). This research was supported by funding agencies in terms of databases and funding.
Acknowledgments
Thanks to LB for providing important guidance, thanks to QW, ZJ, CL, XT, SY, DX, and ZT for providing important references and suggestions for the content of this article.
Conflict of interest
The authors declare that the research was conducted in the absence of any commercial or financial relationships that could be construed as a potential conflict of interest.
Publisher’s note
All claims expressed in this article are solely those of the authors and do not necessarily represent those of their affiliated organizations, or those of the publisher, the editors and the reviewers. Any product that may be evaluated in this article, or claim that may be made by its manufacturer, is not guaranteed or endorsed by the publisher.
References
1. Arai K, Iwanaga S, Toda H, Genci C, Nishiyama Y, Nakamura M. Three-dimensional inkjet biofabrication based on designed images. Biofabrication. (2011) 3:034113. doi: 10.1088/1758-5082/3/3/034113
2. Silver A. Five innovative ways to use 3D printing in the laboratory. Nature. (2019) 565:123–4. doi: 10.1038/d41586-018-07853-5
3. Kim GB, Lee S, Kim H, Yang DH, Kim Y, Kyung YS, et al. Three-dimensional printing: basic principles and applications in medicine and radiology. Korean J Radiol. (2016) 17:182–97. doi: 10.3348/kjr.2016.17.2.182
4. Mitsouras D, Liacouras P, Imanzadeh A, Giannopoulos A, Cai T, Kumamaru KK, et al. Medical 3D printing for the radiologist. Radiographics. (2015) 35:1965–88. doi: 10.1148/rg.2015140320
5. Wake N, Rude T, Kang SK, Stifelman MD, Borin JF, Sodickson DK, et al. 3D printed renal cancer models derived from MRI data: application in pre-surgical planning. Abdom Radiol. (2017) 42:1501–9. doi: 10.1007/s00261-016-1022-2
6. Jacquet JR, Ossant F, Levassort F, Gregoire JM. 3-D-printed phantom fabricated by photopolymer jetting technology for high-frequency ultrasound imaging. IEEE Trans Ultrason Ferroelectr Freq Control. (2018) 65:1048–55. doi: 10.1109/TUFFC.2018.2823545
7. Fullerton JN, Frodsham GC, Day RM. 3D printing for the many, not the few. Nat Biotechnol. (2014) 32:1086–7. doi: 10.1038/nbt.3056
8. Ventola CL. Medical applications for 3D printing: current and projected uses. P T. (2014) 39:704–11.
9. Sheth U, Theodoropoulos J, Abouali J. Use of 3-dimensional printing for preoperative planning in the treatment of recurrent anterior shoulder instability. Arthrosc Tech. (2015) 4:e311–6. doi: 10.1016/j.eats.2015.03.003
10. Vehmeijer M, van Eijnatten M, Liberton N, Wolff J. A novel method of orbital floor reconstruction using virtual planning, 3-dimensional printing, and autologous bone. J Oral Maxillofac Surg. (2016) 74:1608–12. doi: 10.1016/j.joms.2016.03.044
11. Chae MP, Rozen WM, McMenamin PG, Findlay MW, Spychal RT, Hunter-Smith DJ. Emerging applications of bedside 3D printing in plastic surgery. Front Surg. (2015) 2:25. doi: 10.3389/fsurg.2015.00025
12. Giannopoulos AA, Steigner ML, George E, Barile M, Hunsaker AR, Rybicki FJ, et al. Cardiothoracic applications of 3-dimensional printing. J Thorac Imaging. (2016) 31:253–72. doi: 10.1097/RTI.0000000000000217
13. Al Jabbari O, Abu Saleh WK, Patel AP, Igo SR, Reardon MJ. Use of three-dimensional models to assist in the resection of malignant cardiac tumors. J Card Surg. (2016) 31:581–3. doi: 10.1111/jocs.12812
14. Rengier F, Mehndiratta A, von Tengg-Kobligk H, Zechmann CM, Unterhinninghofen R, Kauczor H-U, et al. 3D printing based on imaging data: review of medical applications. Int J Comput Assist Radiol Surg. (2010) 5:335–41. doi: 10.1007/s11548-010-0476-x
15. Schubert C, van Langeveld MC, Donoso LA. Innovations in 3D printing: a 3D overview from optics to organs. Br J Ophthalmol. (2014) 98:159–61. doi: 10.1136/bjophthalmol-2013-304446
16. Perica E, Sun Z. Patient-specific three-dimensional printing for pre-surgical planning in hepatocellular carcinoma treatment. Quant Imaging Med Surg. (2017) 7:668–77. doi: 10.21037/qims.2017.11.02
17. Maddox MM, Feibus A, Liu J, Wang J, Thomas R, Silberstein JL. 3D-printed soft-tissue physical models of renal malignancies for individualized surgical simulation: a feasibility study. J Robot Surg. (2018) 12:27–33. doi: 10.1007/s11701-017-0680-6
18. Zhou Z, Cunningham E, Lennon A, McCarthy HO, Buchanan F, Dunne N. Development of three-dimensional printing polymer-ceramic scaffolds with enhanced compressive properties and tuneable resorption. Mater Sci Eng C Mater Biol Appl. (2018) 93:975–86. doi: 10.1016/j.msec.2018.08.048
19. Mobbs RJ, Choy WJ, Wilson P, McEvoy A, Phan K, Parr WCH. L5 En-Bloc vertebrectomy with customized reconstructive implant: comparison of patient-specific versus off-the-shelf implant. World Neurosurg. (2018) 112:94–100. doi: 10.1016/j.wneu.2018.01.078
20. Wu Z, Zhao J, Wu W, Wang P, Wang B, Li G, et al. Radial compressive property and the proof-of-concept study for realizing self-expansion of 3D printing polylactic acid vascular stents with negative poisson’s ratio structure. Materials. (2018) 11:1357. doi: 10.3390/ma11081357
21. Graham AD, Olof SN, Burke MJ, Armstrong J, Mikhailova EA, Nicholson JG, et al. High-resolution patterned cellular constructs by droplet-based 3D printing. Sci Rep. (2017) 7:7004. doi: 10.1038/s41598-017-06358-x
22. Tan Y, Richards DJ, Trusk TC, Visconti RP, Yost MJ, Kindy MS, et al. 3D printing facilitated scaffold-free tissue unit fabrication. Biofabrication. (2014) 6:024111. doi: 10.1088/1758-5082/6/2/024111
23. Bernhard JC, Isotani S, Matsugasumi T, Duddalwar V, Hung AJ, Suer E, et al. Personalized 3D printed model of kidney and tumor anatomy: a useful tool for patient education. World J Urol. (2016) 34:337–45. doi: 10.1007/s00345-015-1632-2
24. Zhang Y, Ge HW, Li NC, Yu CF, Guo HF, Jin SH, et al. Evaluation of three-dimensional printing for laparoscopic partial nephrectomy of renal tumors: a preliminary report. World J Urol. (2016) 34:533–7. doi: 10.1007/s00345-015-1530-7
25. Knoedler M, Feibus AH, Lange A, Maddox MM, Ledet E, Thomas R, et al. Individualized physical 3-dimensional kidney tumor models constructed from 3-dimensional printers result in improved trainee anatomic understanding. Urology. (2015) 85:1257–61. doi: 10.1016/j.urology.2015.02.053
26. George M, Aroom KR, Hawes HG, Gill BS, Love J. 3D printed surgical instruments: the design and fabrication process. World J Surg. (2017) 41:314–9. doi: 10.1007/s00268-016-3814-5
27. Del Junco M, Yoon R, Okhunov Z, Abedi G, Hwang C, Dolan B, et al. Comparison of flow characteristics of novel three-dimensional printed ureteral stents versus standard ureteral stents in a porcine model. J Endourol. (2015) 29:1065–9. doi: 10.1089/end.2014.0716
28. Park CJ, Kim HW, Jeong S, Seo S, Park Y, Moon HS, et al. Anti-reflux ureteral stent with polymeric flap valve using three-dimensional printing: an in vitro study. J Endourol. (2015) 29:933–8. doi: 10.1089/end.2015.0154
29. Komai Y, Sugimoto M, Gotohda N, Matsubara N, Kobayashi T, Sakai Y, et al. Patient-specific 3-dimensional printed kidney designed for “4D” surgical navigation: a novel aid to facilitate minimally invasive off-clamp partial nephrectomy in complex tumor cases. Urology. (2016) 91:226–33. doi: 10.1016/j.urology.2015.11.060
30. Golab A, Smektala T, Krolikowski M, Slojewski M. Percutaneous nephrolithotomy using an individual 3-dimensionally printed surgical guide. Urol Int. (2018) 100:485–7. doi: 10.1159/000446291
31. Kang HW, Lee SJ, Ko IK, Kengla C, Yoo JJ, Atala A. A 3D bioprinting system to produce human-scale tissue constructs with structural integrity. Nat Biotechnol. (2016) 34:312–9. doi: 10.1038/nbt.3413
32. Orecchia L, Manfrin D, Germani S, Fabbro DD, Asimakopoulos AD, Agrò EF, et al. Introducing 3D printed models of the upper urinary tract for high-fidelity simulation of retrograde intrarenal surgery. 3D Print Med. (2021) 7:15. doi: 10.1186/s41205-021-00105-9
33. Xu Y, Yuan Y, Cai Y, Li X, Wan S, Xu G. Use 3D printing technology to enhance stone free rate in single tract percutaneous nephrolithotomy for the treatment of staghorn stones. Urolithiasis. (2020) 48:509–16. doi: 10.1007/s00240-019-01164-8
34. Denizet G, Calame P, Lihoreau T, Kleinclauss F, Aubry S. 3D multi-tissue printing for kidney transplantation. Quant Imaging Med Surg. (2019) 9:101–6. doi: 10.21037/qims.2018.10.16
35. Chandak P, Byrne N, Coleman A, Karunanithy N, Carmichael J, Marks SD, et al. Patient-specific 3D printing: a novel technique for complex pediatric renal transplantation. Ann Surg. (2019) 269:e18–23. doi: 10.1097/SLA.0000000000003016
36. Xu K, Han Y, Huang Y, Wei P, Yin J, Jiang J. The application of 3D bioprinting in urological diseases. Mater Today Bio. (2022) 16:100388. doi: 10.1016/j.mtbio.2022.100388
37. Vijayavenkataraman S, Yan WC, Lu WF, Wang CH, Fuh JYH. 3D bioprinting of tissues and organs for regenerative medicine. Adv Drug Deliv Rev. (2018) 132:296–332. doi: 10.1016/j.addr.2018.07.004
38. Gungor-Ozkerim PS, Inci I, Zhang YS, Khademhosseini A, Dokmeci MR. Bioinks for 3D bioprinting: an overview. Biomater Sci. (2018) 6:915–46. doi: 10.1039/c7bm00765e
39. Nguyen DG, Pentoney SL Jr. Bioprinted three dimensional human tissues for toxicology and disease modeling. Drug Discov Today Technol. (2017) 23:37–44. doi: 10.1016/j.ddtec.2017.03.001
40. Sochol RD, Gupta NR, Bonventre JVA. Role for 3D printing in kidney-on-a-chip platforms. Curr Transplant Rep. (2016) 3:82–92. doi: 10.1007/s40472-016-0085-x
41. King SM, Higgins JW, Nino CR, Smith TR, Paffenroth EH, Fairbairn CE, et al. 3D proximal tubule tissues recapitulate key aspects of renal physiology to enable nephrotoxicity testing. Front Physiol. (2017) 8:123. doi: 10.3389/fphys.2017.00123
42. Rodríguez-Dévora JI, Zhang B, Reyna D, Shi ZD, Xu T. High throughput miniature drug-screening platform using bioprinting technology. Biofabrication. (2012) 4:035001. doi: 10.1088/1758-5082/4/3/035001
43. Lin YQ, Wang LR, Pan LL, Wang H, Zhu GQ, Liu WY, et al. Kidney bioengineering in regenerative medicine: an emerging therapy for kidney disease. Cytotherapy. (2016) 18:186–97. doi: 10.1016/j.jcyt.2015.10.004
44. Yan WC, Davoodi P, Vijayavenkataraman S, Tian Y, Ng WC, Fuh J, et al. 3D bioprinting of skin tissue: from pre-processing to final product evaluation. Adv Drug Deliv Rev. (2018) 132:270–95. doi: 10.1016/j.addr.2018.07.016
45. Byambaa B, Annabi N, Yue K, Santiago G, Alvarez MM, Jia W, et al. Bioprinted osteogenic and vasculogenic patterns for engineering 3D bone tissue. Adv Healthc Mater. (2017) 6:1700015. doi: 10.1002/adhm.201700015
46. Kucukgul C, Ozler SB, Inci I, Karakas E, Irmak S, Gozuacik D, et al. 3D bioprinting of biomimetic aortic vascular constructs with self-supporting cells. Biotechnol Bioeng. (2015) 112:811–21. doi: 10.1002/bit.25493
47. Bae SW, Lee KW, Park JH, Lee J, Jung CR, Yu J, et al. 3D bioprinted artificial trachea with epithelial cells and chondrogenic-differentiated bone marrow-derived mesenchymal stem cells. Int J Mol Sci. (2018) 19:1624. doi: 10.3390/ijms19061624
48. Bejleri D, Streeter BW, Nachlas ALY, Brown ME, Gaetani R, Christman KL, et al. A bioprinted cardiac patch composed of cardiac-specific extracellular matrix and progenitor cells for heart repair. Adv Healthc Mater. (2018) 7:e1800672. doi: 10.1002/adhm.201800672
49. Apelgren P, Amoroso M, Säljö K, Lindahl A, Brantsing C, Orrhult LS, et al. Skin grafting on 3D bioprinted cartilage constructs in vivo. Plast Reconstr Surg Glob Open. (2018) 6:e1930. doi: 10.1097/GOX.0000000000001930
50. Reint G, Rak-Raszewska A, Vainio SJ. Kidney development and perspectives for organ engineering. Cell Tissue Res. (2017) 369:171–83. doi: 10.1007/s00441-017-2616-x
51. Monda SM, Weese JR, Anderson BG, Vetter JM, Venkatesh R, Du K, et al. Development and validity of a silicone renal tumor model for robotic partial nephrectomy training. Urology. (2018) 114:114–20. doi: 10.1016/j.urology.2018.01.030
52. Lee H, Nguyen NH, Hwang SI, Lee HJ, Hong SK, Byun SS. Personalized 3D kidney model produced by rapid prototyping method and its usefulness in clinical applications. Int Braz J Urol. (2018) 44:952–7. doi: 10.1590/S1677-5538.IBJU.2018.0162
53. Kotta S, Nair A, Alsabeelah N. 3D printing technology in drug delivery: recent progress and application. Curr Pharm Des. (2018) 24:5039–48. doi: 10.2174/1381612825666181206123828
54. Do AV, Worthington KS, Tucker BA, Salem AK. Controlled drug delivery from 3D printed two-photon polymerized poly(ethylene glycol) dimethacrylate devices. Int J Pharm. (2018) 552:217–24. doi: 10.1016/j.ijpharm.2018.09.065
55. Shim IK, Yi HJ, Yi HG, Lee CM, Lee YN, Choi YJ, et al. Locally-applied 5-fluorouracil-loaded slow-release patch prevents pancreatic cancer growth in an orthotopic mouse model. Oncotarget. (2017) 8:40140–51. doi: 10.18632/oncotarget.17370
56. Song TH, Jang J, Choi YJ, Shim JH, Cho DW. 3D-printed drug/cell carrier enabling effective release of cyclosporin a for xenogeneic cell-based therapy. Cell Transplant. (2015) 24:2513–25. doi: 10.3727/096368915X686779
57. Ilyés K, Kovács NK, Balogh A, Borbás E, Farkas B, Casian T, et al. The applicability of pharmaceutical polymeric blends for the fused deposition modelling (FDM) 3D technique: material considerations-printability-process modulation, with consecutive effects on in vitro release, stability and degradation. Eur J Pharm Sci. (2019) 129:110–23. doi: 10.1016/j.ejps.2018.12.019
58. Jang KJ, Mehr AP, Hamilton GA, McPartlin LA, Chung S, Suh KY, et al. Human kidney proximal tubule-on-a-chip for drug transport and nephrotoxicity assessment. Integr Biol. (2013) 5:1119–29. doi: 10.1039/c3ib40049b
59. Sakolish C, Weber EJ, Kelly EJ, Himmelfarb J, Mouneimne R, Grimm FA, et al. Technology transfer of the microphysiological systems: a case study of the human proximal tubule tissue chip. Sci Rep. (2018) 8:14882. doi: 10.1038/s41598-018-33099-2
60. Weber EJ, Chapron A, Chapron BD, Voellinger JL, Lidberg KA, Yeung CK, et al. Development of a microphysiological model of human kidney proximal tubule function. Kidney Int. (2016) 90:627–37. doi: 10.1016/j.kint.2016.06.011
61. Tourovskaia A, Fauver M, Kramer G, Simonson S, Neumann T. Tissue-engineered microenvironment systems for modeling human vasculature. Exp Biol Med. (2014) 239:1264–71. doi: 10.1177/1535370214539228
Keywords: three-dimensional (3D) printing, kidney, preoperative communication, renal carcinoma, renal calculus, renal transplantation, pharmaceutical research
Citation: Dai S, Wang Q, Jiang Z, Liu C, Teng X, Yan S, Xia D, Tuo Z and Bi L (2022) Application of three-dimensional printing technology in renal diseases. Front. Med. 9:1088592. doi: 10.3389/fmed.2022.1088592
Received: 03 November 2022; Accepted: 21 November 2022;
Published: 02 December 2022.
Edited by:
Nithesh Naik, Manipal Academy of Higher Education, IndiaReviewed by:
Sudipto Datta, Indian Institute of Engineering Science and Technology, Shibpur, IndiaGang Fan, Charité – Universitätsmedizin Berlin, Germany
Chengpeng Chen, University of Maryland, Baltimore, United States
Copyright © 2022 Dai, Wang, Jiang, Liu, Teng, Yan, Xia, Tuo and Bi. This is an open-access article distributed under the terms of the Creative Commons Attribution License (CC BY). The use, distribution or reproduction in other forums is permitted, provided the original author(s) and the copyright owner(s) are credited and that the original publication in this journal is cited, in accordance with accepted academic practice. No use, distribution or reproduction is permitted which does not comply with these terms.
*Correspondence: Liangkuan Bi, YmlsaWFuZ2t1YW5AMTI2LmNvbQ==
†These authors have contributed equally to this work