- 1Wal-yan Respiratory Research Centre, Telethon Kids Institute, The University of Western Australia, Nedlands, WA, Australia
- 2Department of Respiratory and Sleep Medicine, Perth Children’s Hospital, Nedlands, WA, Australia
- 3Centre for Cell Therapy and Regenerative Medicine, School of Medicine and Pharmacology, The University of Western Australia and Harry Perkins Institute of Medical Research, Nedlands, WA, Australia
- 4School of Population Health, Curtin University, Bentley, WA, Australia
Persistent respiratory bacterial infections are a clinical burden in several chronic inflammatory airway diseases and are often associated with neutrophil infiltration into the lungs. Following recruitment, dysregulated neutrophil effector functions such as increased granule release and formation of neutrophil extracellular traps (NETs) result in damage to airway tissue, contributing to the progression of lung disease. Bacterial pathogens are a major driver of airway neutrophilic inflammation, but traditional management of infections with antibiotic therapy is becoming less effective as rates of antimicrobial resistance rise. Bacteriophages (phages) are now frequently identified as antimicrobial alternatives for antimicrobial resistant (AMR) airway infections. Despite growing recognition of their bactericidal function, less is known about how phages influence activity of neutrophils recruited to sites of bacterial infection in the lungs. In this review, we summarize current in vitro and in vivo findings on the effects of phage therapy on neutrophils and their inflammatory mediators, as well as mechanisms of phage-neutrophil interactions. Understanding these effects provides further validation of their safe use in humans, but also identifies phages as a targeted neutrophil-modulating therapeutic for inflammatory airway conditions.
Introduction
Chronic airway diseases are major causes of human mortality (1, 2), represented by conditions such as cystic fibrosis (CF), chronic obstructive pulmonary disease (COPD), bronchiectasis, and asthma. Key features of these diseases are recurrent lower respiratory bacterial infections, which over repeated courses of antibiotic therapy can become antimicrobial resistant (AMR). Across these lung conditions, neutrophilic inflammation sustained by frequent infections can be a major driver of airway damage (3), yet effective anti-inflammatory and neutrophil-targeted therapies are not available.
An emerging tool against AMR infections is the therapeutic use of bacteriophages or phages, viruses that infect bacteria, ubiquitously and abundantly present in the environment (4). First identified in the early twentieth century, phages were initially investigated for use as antimicrobials in humans following observations of bacterial killing in vitro (5). Upon the discovery of penicillin and other antimicrobial compounds in the 1940s, interest in phages waned as academia and industry focused on development of these drugs; however, therapeutic phage centers have remained active in certain countries (5). With the emergence of antimicrobial resistance, and four decades since the last antibiotic drug was discovered, there is resurging interest in phages as potential alternatives. Phage therapy works by exploiting the life cycle of lytic phages, which in the process of replication lyse and kill their bacterial host (5). Phages have several characteristics that support their clinical use. They do not infect human cells (5), appear to be safe and well tolerated (6–8), may require less doses compared to conventional antibiotics due to their self-replicating nature (9, 10), and are highly specific to their target bacterial species, meaning unlike antibiotics they do not have broad bactericidal activity against the host microbiome (7, 11). The use of phages as a standard clinical therapeutic is hampered by a still incomplete understanding of phage biology (12), as well as a lack of regulatory manufacturing guidelines for phage products (13, 14) and standardized large-scale clinical trials (15); however, science, medicine, and industry are progressively working to surmount these challenges. In the context of chronic respiratory diseases, phage therapy is now being explored as a treatment for pulmonary infections (16, 17). Intriguingly, emerging evidence suggests that administration of phages may also have significant therapeutic benefits for managing neutrophilic inflammation in the lungs (18–21).
The role of bacterial infections in chronically diseased airways
The link between bacterial airway infections and chronic inflammatory lung diseases is well established. One significant bacterium in this regard is the opportunistic pathogen Pseudomonas aeruginosa, a species which is notably associated with severe and negative health outcomes across multiple chronic airway conditions (22). Perhaps most striking is the early childhood acquisition in the autosomal recessive disorder CF, where P. aeruginosa contributes to reduced lung function (23–26), increased airway inflammation (27, 28), permanent airway remodeling (29, 30), and increased mortality in individuals with the disease (31, 32). Treatment strategies initiating eradication therapy in children with CF have reduced P. aeruginosa colonization rates from 80 to 50% (33, 34), but acquisition of this bacterium remains a key determinant of long-term CF clinical outcomes (31, 35). Among individuals with COPD, up to 40% will have positive sputum cultures for P. aeruginosa (36–39), with over 10% meeting criteria for colonization (39–41). In addition, up to a third of participants in cohorts of non-CF bronchiectasis can be colonized by this pathogen (42–44). The degree to which P. aeruginosa colonization in non-CF airway diseases contributes to lung function decline is still not clear (45), but multiple studies in both COPD and bronchiectasis link P. aeruginosa to more frequent exacerbations and/or hospitalization (36, 38, 42, 44, 46–48), increased mortality (36, 44, 46, 47, 49, 50), and greater annual lung function decline (40).
The role of bacterial infections in the pathogenesis of asthma is not as well understood as that of respiratory viruses, which are associated with childhood wheezing, compromised epithelial barrier function, asthma development, and exacerbations (51, 52). However, studies have still noted associations between bacterial pathogens and asthma pathologies. For example, in a cohort of 56 asthmatic patients from Royal Brompton Hospital, London, sputum bacterial culture positivity with P. aeruginosa, Haemophilus influenzae, and Staphylococcus aureus was significantly associated with increased asthma duration and frequency of exacerbations in the previous year (53). Other factors including pneumonia, pathogen isolation, as well as sputum production and purulence have also been identified and associated with the development of bronchiectasis in asthma cohorts (54, 55).
A primary concern with treating frequent lung infections in the context of these diseases is the acquisition of antimicrobial resistance, with some pathogens becoming multi-drug resistant (MDR). This makes eradication of these bacterial infections challenging and increases the treatment burden of patients with chronic lung conditions. In 2019, lower respiratory infections globally accounted for over 1.5 million out of 4.95 million estimated deaths associated with antimicrobial resistance, more than any other infectious syndrome (56). Among individuals with chronic lung diseases, acquisition of AMR/MDR pathogens is associated with increased disease severity (57–59), exacerbations (57, 59, 60), and mortality (31, 61). With prevalence of chronic airway conditions increasing by nearly 40% since 1990 (62, 63), novel therapeutics to treat AMR lung infections are desperately needed.
Neutrophils, drivers of lung damage
As one of the first immune cell types recruited to sites of infection, neutrophils have an important role in the innate immune response to respiratory bacterial infections (64). Historically perceived as functionally rigid and transcriptionally fixed, neutrophils are increasingly described as plastic cells whose function is shaped by their environment (65–67). In the context of chronic lung diseases, studies assessing neutrophils recruited to diseased airways have observed changes in neutrophil antimicrobial functions that result in airway damage and contribute to lung disease progression.
One of the major mechanisms by which neutrophils can damage the airways is through release of neutrophil elastase (NE), a serine protease normally stored within intracellular primary granules. Airway NE is an important marker of inflammation in CF, significantly correlating with severity of lung disease in both children and adults (68, 69). Infection with P. aeruginosa is associated with increased sputum NE activity in adults with CF (70), as well as prolonged NE activity in pediatric CF airways (28). Release of NE by neutrophils in CF airways was originally thought to be a consequence of neutrophil death, but studies within the last decade have described how this process occurs from granule exocytosis by viable neutrophil populations in CF lungs (71–73). Work by our group using in vitro modeling of the airway infection environment created by P. aeruginosa has demonstrated that infection induces neutrophil degranulation (74). We found that neutrophils recruited to infection microenvironments primed by P. aeruginosa had significantly increased staining of CD63 and CD66b, neutrophil markers of primary and secondary granule exocytosis, respectively (74). In COPD, airway NE is elevated during exacerbations and can be predictive of bacterially induced exacerbations (75). Studies are also identifying airway NE as a potential biomarker of disease severity in non-CF bronchiectasis. For example, in a cohort of 433 adult patients, Chalmers and colleagues found significant associations between high sputum NE levels and increased dyspnea scores, lung function decline, exacerbation frequency, and radiological scoring of bronchiectasis severity (76). Sputum NE was elevated during exacerbations and reduced in response to antibiotic therapy targeting organisms such as P. aeruginosa and H. influenzae, highlighting a relationship between release of NE and bacterial respiratory infection (76). Another recent pediatric cross-sectional study of both CF and non-CF related bronchiectasis also found that sputum NE significantly correlated with exacerbation severity and frequency, as well as number of hospitalizations (77). For CF bronchiectasis it was specifically observed that NE correlated with risk of disease progression and increased lung function decline, while for non-CF bronchiectasis, sputum NE positively correlated with airway neutrophil counts and severity of lung disease (77). Asthma is a chronic lung disease with a much broader range of inflammatory phenotypes, and while eosinophil activity is critical to some cohorts, some of the more severe forms of asthma are primarily a result of airway neutrophilic inflammation (78). Past studies of asthma have associated increased airway NE (79–81) and myeloperoxidase (82), another factor released from primary granules, with more severe disease. Studies on allergic asthmatic responses in animal models have also shown reduced airway inflammation (83, 84) and bronchoconstriction (85) following treatment with NE inhibitors.
Neutrophil degranulation and NE release seem to coincide with a reduction in phagocytic ability that, certainly in CF, may contribute to further disease (71–74, 86). There are few reports describing decreased phagocytosis by neutrophils in COPD (87–89), bronchiectasis (90, 91), and asthma (92), and further investigation is required to definitively conclude whether this is a feature of non-CF lung diseases. Impairment of this crucial neutrophil function may contribute to prolonged infection, pathogen colonization, and associated negative health outcomes in chronic airway diseases, and may explain in part why neutrophils in these conditions resort to alternative antimicrobial strategies detrimental to host airways.
In the last 20 years, a novel neutrophil antimicrobial function was discovered and linked to the production of neutrophil extracellular traps (NETs) (93). This was termed NETosis, a process in which neutrophils eject extracellular networks of DNA containing primary granules, NE and other antimicrobial factors, which can trap and neutralize invading pathogens. Also thought to be an event resulting in neutrophil death (94), different NETosis pathways have been described that utilize mitochondrial DNA release rather than nuclear DNA (95), or preserve neutrophil viability after NET formation (96, 97). The degree to which NETs significantly contribute to pathogen clearance is debated (98, 99). The toxic antimicrobial factors harbored within NET complexes may instead contribute to airway damage. Interest in NETs has increased since studies identified them as major sputum components in chronically disease airways (100–102). In CF, NETs influence airway mucus viscosity (100, 103) and are associated with increased airway obstruction (104). CF neutrophils are also inherently predisposed to increased NET formation, delayed apoptosis and increased lifespan as a result of CFTR dysfunction (105). NET formation in COPD sputum has been found to significantly correlate with disease severity, lung function decline, and exacerbation frequency (101, 106). In severe asthma, high extracellular DNA indicative of increased NETosis has been associated with increased corticosteroid use, neutrophilic inflammation, and inflammasome activation (107). An international observational study by Keir et al. performed proteomic analysis of sputum from bronchiectasis patients, finding that NET proteins were abundantly present and strongly associated with increased disease severity, hospital admissions, and mortality (102). A separate study within this report further showed that low doses of antibiotics over a 12-month period was associated with NET reduction in sputum from individuals with bronchiectasis or asthma (102), underscoring the connection between NETosis and airway bacteria.
Limiting neutrophil migration to the lungs would appear to be a simple solution for preventing damage by aberrant functions of recruited neutrophils. However, reducing airway neutrophil influx can have negative consequences, as was the case in a phase two clinical trial of the leukotriene B4 (LTB4) receptor antagonist BIIL 284 BS (108). This trial was prematurely terminated upon discovering a significant increase in serious adverse events among CF patients receiving the drug (108). A follow up study assessing participant samples and BIIL 284 treatment in P. aeruginosa infected mice determined that the drug significantly reduced airway neutrophil counts, leading to increased P. aeruginosa in the lung, bacteremia, and increased lung inflammation (109). This suggests that outright reduction of neutrophil numbers in infected lungs is not therapeutically beneficial; a therapy that instead amends neutrophil pathological activity, preserves phagocytic function, and aids in bacterial clearance, may be more effective. In chronic lung diseases, the interplay between bacterial respiratory infections, neutrophilic inflammation, and airway damage highlights an important need for therapies that can treat infection, and the inflammatory processes and neutrophil functions that result in damage to the lungs (Figure 1).
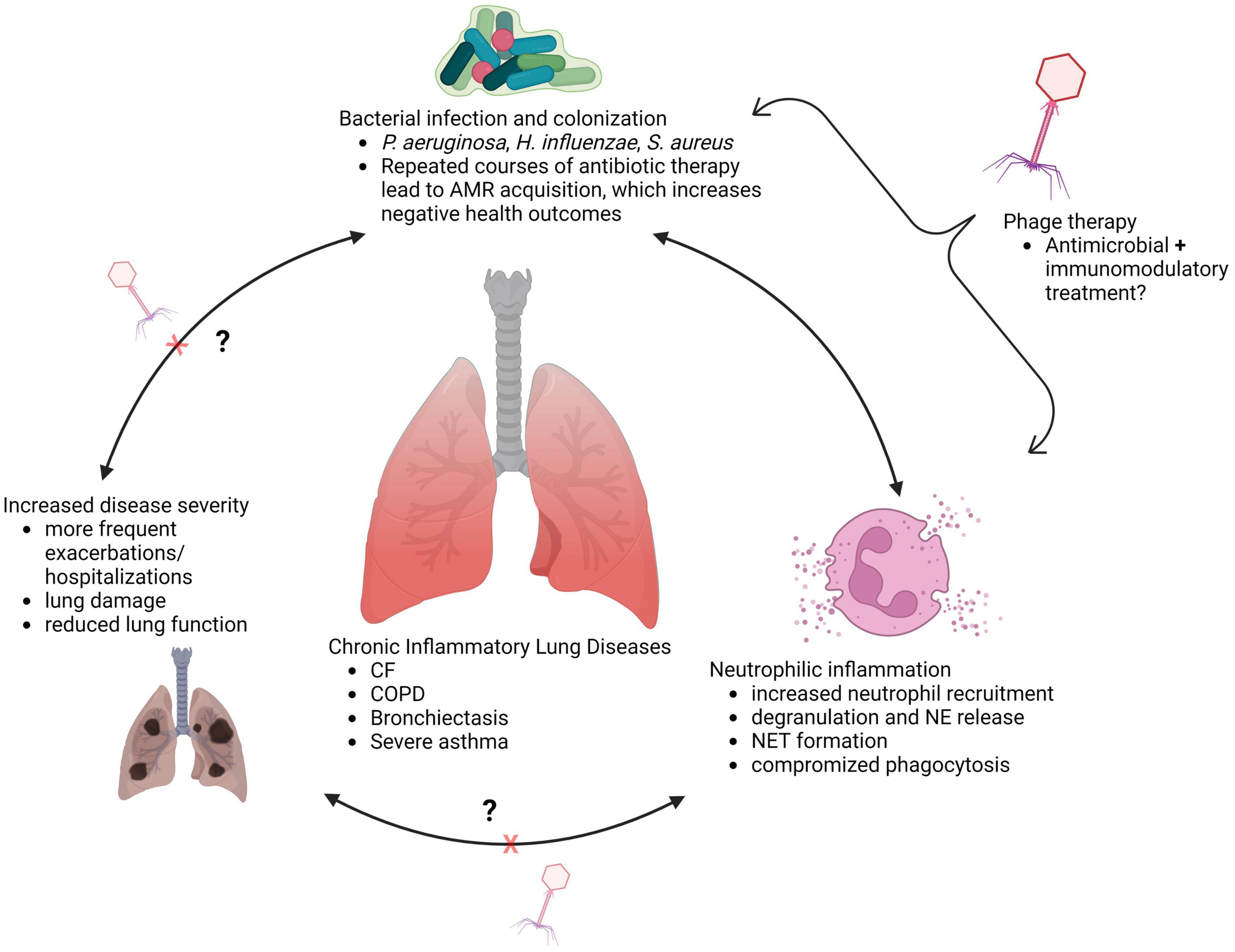
Figure 1. Interplay between infection and inflammation in chronically diseased lungs (created with BioRender.com).
Phage therapy and airway inflammation
Trials of phage therapy for respiratory infections in humans have occurred in a limited number of instances for compassionate use, particularly in cases with MDR pathogens where the traditional spectrum of antibiotics failed to clear infection. Many of the resulting case reports have described positive outcomes, with no adverse effects and infections successfully cleared in treated patients (110–115). However, despite increasing validation for use as antimicrobials in humans, little is known about the innate immune response to respiratory phage therapy, and how airway neutrophils recruited during bacterial infection may respond to treatment. A handful of studies in small animal models have provided some data on inflammation following therapeutic phage administration during experimental airway infection (20, 116–119).
Airway neutrophil recruitment is initiated by detection of chemotactic signals such as interleukin (IL)-8 and LTB4 (120). As neutrophils exit circulation and migrate through tissue, they can encounter additional inflammatory cytokines such as IL-1, IL-6, IL-18, TNFα, and become primed, further increasing their responsiveness and propensity for pathological activation (67, 121). Thus, host cytokines have an important role in modulating neutrophil responses during infection. A study by Pabary et al. assessed the effects of an intranasally administered mixture of individual phages or phage cocktail, before, during, and after P. aeruginosa inoculation in mice, measuring inflammatory markers and neutrophil counts in bronchoalveolar lavage fluid (BALF) (116). In experimental infections with P. aeruginosa reference strain PAO1, phage treatment at all timepoints significantly reduced viable bacterial numbers, but only the prophylactic administration of phages significantly reduced BALF neutrophil counts compared to untreated animals (116). Simultaneous inoculation with phages and bacteria significantly reduced IL-10 and IL-1β compared to animals infected with bacteria alone, while both delayed and prophylactic administration of phages significantly reduced the neutrophil chemokine keratinocyte chemoattractant (KC) (116). A CF clinical isolate of P. aeruginosa was also tested, inoculated simultaneously with lytic phages. Curiously, bacterial clearance of this isolate was not enhanced with phage treatment compared to untreated animals; however, the authors acknowledged differences in bacterial doses and BALF sampling times in experiments with the clinical isolate vs. PAO1 that may account for this (116). Nevertheless, phage treatment in this experiment significantly reduced both neutrophil counts and pro-inflammatory mediators IL-6, IL-10, IL-12p70, KC, and TNFα in BALF of treated animals compared to untreated controls (116). A different study using bioluminescent P. aeruginosa was able to image phage-mediated clearance in the lungs of infected mice, with treatment with phages reducing bacterial luminescent signal from the lungs, increasing animal survival, and reducing IL-6 and TNFα in BALF (117). It was also determined that prophylactic phage administration twenty-fours prior to bacterial inoculation had a protective effect against infection (117). Importantly, a study of Escherichia coli pneumonia in mice showed that bacterial lysis induced by phage therapy induces similar levels of cytokine release as lysis induced by antibiotics, with phages primarily reducing release of most inflammatory signals (118). This suggests phage-induced bacterial lysis is unlikely to result in more severe inflammation compared to activity of conventional antibiotics, but additional research is needed to verify this.
While by design not a respiratory model, a CFTR loss-of-function zebrafish model has been used by Cafora et al. to describe the immunomodulatory potential of phage therapy in CF across two studies. In the first, a phage cocktail administered to zebrafish embryos infected with PAO1 was observed to significantly reduce bacterial load, lethality, and gene expression of IL-1β and TNFα (119). Of note, reduced cytokine gene expression was also observed in embryos exposed to phages alone in the absence of bacteria, suggesting phage anti-inflammatory mechanisms independent of bactericidal activity (119). This was a major focus of the second study, which identified that embryo toll-like receptor (TLR) recognition of phage capsid proteins, and not phage DNA, was necessary to elicit an anti-inflammatory effect (20). The injection of a phage cocktail at the site of experimental tail amputation was further observed to reduce neutrophil recruitment to wound sites, further demonstrating phage capacity to influence localized inflammation (20).
As a direct barrier to infection, the airway epithelium is a major source of neutrophil chemotactic signals and inflammatory mediators (122, 123). In perhaps one of the only studies assessing effects of phage therapy on primary airway epithelial cells, Trend and colleagues performed exposures of undifferentiated primary airway epithelial cell cultures to the virulent P. aeruginosa phage E79 (124). They observed that E79 did not increase release of pro-inflammatory cytokines IL-1β, IL-6, IL-8, or induce apoptosis, in airway cultures derived from children with and without CF (124), indicating that phages alone are not highly immunostimulatory to human airway cells. Altogether, studies suggest that phage therapy can effectively reduce cytokine signals involved in neutrophil recruitment and activation. This effect is not always a consequence of overt antimicrobial activity, with induction of anti-inflammatory mediators (125), reduced production of reactive oxygen species (126), and LPS binding (127) identified as possible mechanisms. This would make phages an attractive multipurpose therapeutic for managing both airway inflammation and infection in chronic lung diseases. However, further investigation is necessary to understand the specific mechanisms of phage anti-inflammatory activity.
Phage-neutrophil interactions
The interactions between phages and human phagocytes have been of interest to researchers since the 1920s, when a number of early studies noted increased phagocytosis of bacteria by leukocytes in the presence of phages (128–130). More recent studies in neutropenic mouse models have noted a synergism between phages and neutrophils that is required for successful clearance of bacteria. An investigation by Tiwari et al. found that immunocompetent mice inoculated intranasally with a lethal dose of PAO1 could clear lung infection and maintain an 80–100% survival rate when receiving different doses of lytic phages; however, neutropenic animals failed to clear infection with phage administration alone (131). Roach and colleagues took this approach a step further, using in silico modeling to identify host innate responses as a necessary feature to overcome emerging phage resistant mutants during respiratory P. aeruginosa infection, and neutrophil activity as a key component of successful phage therapy (21). Whether this synergy implies phage-mediated enhancement of neutrophil bacterial killing capacity is an important question for future research. Some studies have linked phages to increased intracellular killing of pathogens within human phagocytes such as Klebsiella pneumoniae (132), Mycobacterium avium and tuberculosis (133), and methicillin-resistant S. aureus (134, 135). There are contrasting reports, however, where phages did not significantly influence intracellular killing of pathogenic bacteria (136, 137).
Whether phages contribute to activation of pathological neutrophil functions is critical to ascertain for safe use of this therapy. A study of neutrophil exposure to lytic phages observed little to no respiratory burst activity induced by T4 E. coli phage and A3/R S. aureus phage preparations, compared to heat inactivated S. aureus cells, suggesting that phages alone should not induce oxidative stress when administered in humans (19). Importantly, it has also been shown that A3/R phage and S. aureus phage lysate do not elicit neutrophil degranulation, as indicated by low neutrophil expression of CD63 and CD66b (18). This implies that both phages and phage-mediated lysis of bacteria are not likely to activate neutrophil degranulation and consequent NE release during treatment in vivo. However, availability of data in this regard is inconsistent across bacterial pathogens and neutrophil activation states, so there remains much work to be done for a definitive understanding. Further exploration of whether phage therapy can restore neutrophil phagocytosis of evasive organisms and ameliorate aberrant functions such as degranulation is warranted, particularly in the context of inflammatory airway diseases.
Modeling phage therapy and neutrophilic inflammation in the laboratory
Altogether, studies to date suggest potential anti-inflammatory and neutrophil-modulating benefits of phage therapy for respiratory infections. Further research on this topic is justified, as findings could point to novel therapeutic benefits with capacity to improve treatment of multiple chronic inflammatory lung conditions. However, several factors must be taken into account for relevant modeling of human airway immune responses during phage therapy. For the purposes of understanding airway cell and neutrophil responses to pathogen associated molecular patterns during infection, a major limitation of murine models is differential TLR expression. Mice contain a pseudogene for TLR10, an anti-inflammatory TLR shown to detect bacterial and viral ligands, which is normally expressed by human cells (138). Furthermore, mice and rats express three TLRs that are not express in humans, TLRs 11, 12, and 13, which detect flagellin, fungal profilin, and bacterial ribosomal sequences, respectively (139). In addition, mouse neutrophils may not be activated by certain microbial factors that normally affect human neutrophils, as has been reported with staphylococcal superantigen-like protein 13 (SSL13) (140). These limitations can be overcome by using large animal models of chronic airway disease including pigs and ferrets (Figure 2) (141, 142), whose airway physiology and TLR expression more closely resemble that of human airways (143).
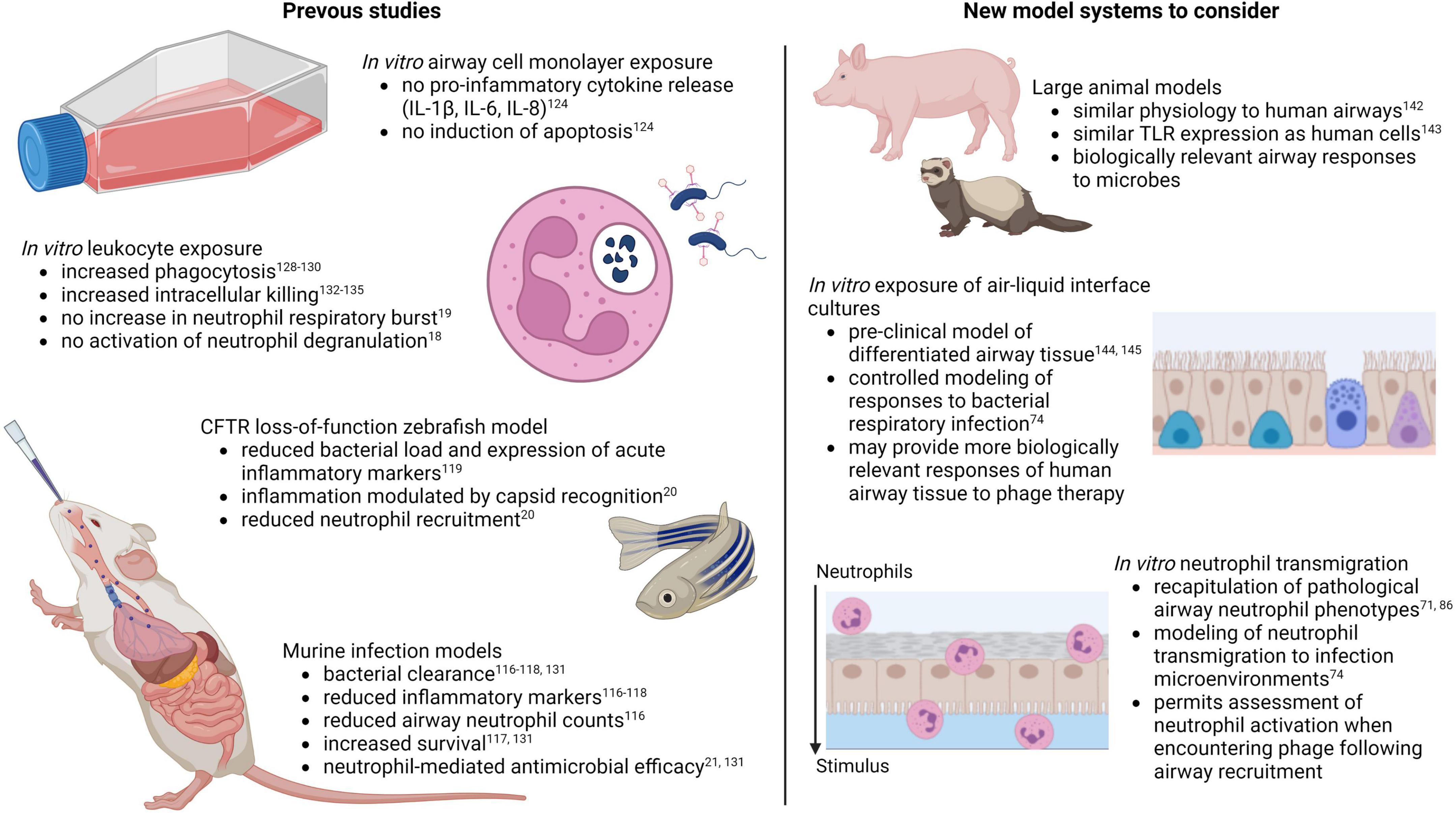
Figure 2. Models for assessing innate immune responses to pulmonary phage therapy (created with BioRender.com).
A major drawback of large animal models is the high cost, labor, and resources required. This is where cell-based laboratory models have an advantage. Few studies to date have assessed phage safety and efficacy on cultures of differentiated primary airway epithelial cells known as air-liquid interface cultures (Figure 2) (144, 145), the gold-standard model for pre-clinical studies in human airways. Further research in this model may provide valuable and biologically relevant insights on innate responses to phage therapy in human lungs. Regarding in vitro studies of phage-neutrophil interactions, one of the major caveats of previous studies is the restricted exposure of phages to peripheral blood neutrophils. This may provide insights into how neutrophils in circulation interact with phages delivered by intravenous injection, but it fails to account for the fact that the site of infection and the extravasation process itself contribute to pathological neutrophil activation (66, 67). Granule releasing neutrophils in CF, as an example, are only evident in the airway lumen, as peripheral blood neutrophils in individuals with CF are phenotypically similar to neutrophils from non-CF individuals (71, 73). Furthermore, recapitulation of this neutrophil phenotype in the laboratory can only be achieved following in vitro transmigration (71, 86). Existing laboratory models of neutrophil recruitment to the lungs may provide more relevant examples of neutrophil behavior during respiratory phage therapy, as neutrophil responses to phages can be observed following transmigration to the airway infection environment (Figure 2).
Conclusion
In summary, neutrophils are important and necessary for the clearance of bacterial respiratory pathogens. In chronic and inflammatory airway diseases, persistent bacterial infections sustain neutrophil influx into the lungs, wherein exaggerated neutrophil antimicrobial functions can result in host tissue damage. Phage therapy is emerging as novel therapeutic for AMR lung infections resulting from prolonged antibiotic use. A growing body of evidence suggests phage therapy may have important immunomodulatory benefits. Whether this is primarily a consequence of reduced bacterial burden or direct interaction between phages and neutrophils merits further investigation. Various laboratory model systems are available to assess airway innate responses to phage therapy; researchers must ensure that models are representative of these dynamics in human airways.
Author contributions
DL, LG, and AK conceived the review, conduced the literature search, and wrote the manuscript. SS critically reviewed and edited the manuscript. All authors approved the final version.
Funding
This work was supported in part by an NHMRC Synergy Grant (1183640). SS holds an NHMRC Investigator Grant (2007725) and AK is a Rothwell Family Fellow.
Conflict of interest
The authors declare that the research was conducted in the absence of any commercial or financial relationships that could be construed as a potential conflict of interest.
Publisher’s note
All claims expressed in this article are solely those of the authors and do not necessarily represent those of their affiliated organizations, or those of the publisher, the editors and the reviewers. Any product that may be evaluated in this article, or claim that may be made by its manufacturer, is not guaranteed or endorsed by the publisher.
References
1. Murphy SLKK, Xu JQ, Arias E. Mortality in the United States, 2020. Hyattsville, MD: National Center for Health Statistics (2021).
3. Jasper AE, McIver WJ, Sapey E, Walton GM. Understanding the role of neutrophils in chronic inflammatory airway disease. F1000Res. (2019) 8:F1000FacultyRev–557. doi: 10.12688/f1000research.18411.1
4. Jurczak-Kurek A, Gasior T, Nejman-Falenczyk B, Bloch S, Dydecka A, Topka G, et al. Biodiversity of bacteriophages: morphological and biological properties of a large group of phages isolated from urban sewage. Sci Rep. (2016) 6:34338. doi: 10.1038/srep34338
5. Wittebole X, De Roock S, Opal SM. A historical overview of bacteriophage therapy as an alternative to antibiotics for the treatment of bacterial pathogens. Virulence. (2014) 5:226–35. doi: 10.4161/viru.25991
6. Speck P, Smithyman A. Safety and efficacy of phage therapy via the intravenous route. FEMS Microbiol Lett. (2016) 363:fnv242. doi: 10.1093/femsle/fnv242
7. Sarker SA, McCallin S, Barretto C, Berger B, Pittet AC, Sultana S, et al. Oral T4-like phage cocktail application to healthy adult volunteers from Bangladesh. Virology. (2012) 434:222–32. doi: 10.1016/j.virol.2012.09.002
8. Sarker SA, Sultana S, Reuteler G, Moine D, Descombes P, Charton F, et al. Oral phage therapy of acute bacterial diarrhea with two Coliphage preparations: a randomized trial in children from Bangladesh. EBioMedicine. (2016) 4:124–37. doi: 10.1016/j.ebiom.2015.12.023
9. Loc-Carrillo C, Abedon ST. Pros and cons of phage therapy. Bacteriophage. (2011) 1:111–4. doi: 10.4161/bact.1.2.14590
10. Principi N, Silvestri E, Esposito S. Advantages and limitations of bacteriophages for the treatment of bacterial infections. Front Pharmacol. (2019) 10:513. doi: 10.3389/fphar.2019.00513
11. Chibani-Chennoufi S, Sidoti J, Bruttin A, Kutter E, Sarker S, Brussow H. In vitro and in vivo bacteriolytic activities of Escherichia coli phages: implications for phage therapy. Antimicrob Agents Chemother. (2004) 48:2558–69. doi: 10.1128/AAC.48.7.2558-2569.2004
12. Venturini C, Petrovic Fabijan A, Fajardo Lubian A, Barbirz S, Iredell J. Biological foundations of successful bacteriophage therapy. EMBO Mol Med. (2022) 14:e12435. doi: 10.15252/emmm.202012435
13. Pirnay JP, Ferry T, Resch G. Recent progress toward the implementation of phage therapy in Western medicine. FEMS Microbiol Rev. (2022) 46:fuab040. doi: 10.1093/femsre/fuab040
14. Pires DP, Costa AR, Pinto G, Meneses L, Azeredo J. Current challenges and future opportunities of phage therapy. FEMS Microbiol Rev. (2020) 44:684–700. doi: 10.1093/femsre/fuaa017
15. Uyttebroek S, Chen B, Onsea J, Ruythooren F, Debaveye Y, Devolder D, et al. Safety and efficacy of phage therapy in difficult-to-treat infections: a systematic review. Lancet Infect Dis. (2022) 22:e208–20. doi: 10.1016/S1473-3099(21)00612-5
16. Chang RYK, Wallin M, Lin Y, Leung SSY, Wang H, Morales S, et al. Phage therapy for respiratory infections. Adv Drug Deliv Rev. (2018) 133:76–86. doi: 10.1016/j.addr.2018.08.001
17. Ng RN, Tai AS, Chang BJ, Stick SM, Kicic A. Overcoming challenges to make bacteriophage therapy standard clinical treatment practice for cystic fibrosis. Front Microbiol. (2020) 11:593988. doi: 10.3389/fmicb.2020.593988
18. Borysowski J, Miedzybrodzki R, Wierzbicki P, Klosowska D, Korczak-Kowalska G, Weber-Dabrowska B, et al. A3R phage and Staphylococcus aureus lysate do not induce neutrophil degranulation. Viruses. (2017) 9:36. doi: 10.3390/v9020036
19. Borysowski J, Wierzbicki P, Klosowska D, Korczak-Kowalska G, Weber-Dabrowska B, Gorski A. The effects of T4 and A3/R phage preparations on whole-blood monocyte and neutrophil respiratory burst. Viral Immunol. (2010) 23:541–4. doi: 10.1089/vim.2010.0001
20. Cafora M, Brix A, Forti F, Loberto N, Aureli M, Briani F, et al. Phages as immunomodulators and their promising use as anti-inflammatory agents in a cftr loss-of-function zebrafish model. J Cyst Fibros. (2021) 20:1046–52. doi: 10.1016/j.jcf.2020.11.017
21. Roach DR, Leung CY, Henry M, Morello E, Singh D, Di Santo JP, et al. Synergy between the host immune system and bacteriophage is essential for successful phage therapy against an acute respiratory pathogen. Cell Host Microbe. (2017) 22:38–47.e4. doi: 10.1016/j.chom.2017.06.0
22. Garcia-Clemente M, de la Rosa D, Maiz L, Giron R, Blanco M, Olveira C, et al. Impact of Pseudomonas aeruginosa infection on patients with chronic inflammatory airway diseases. J Clin Med. (2020) 9:3800. doi: 10.3390/jcm9123800
23. Pillarisetti N, Williamson E, Linnane B, Skoric B, Robertson CF, Robinson P, et al. Infection, inflammation, and lung function decline in infants with cystic fibrosis. Am J Respir Crit Care Med. (2011) 184:75–81. doi: 10.1164/rccm.201011-1892OC
24. Ramsey KA, Ranganathan S, Park J, Skoric B, Adams AM, Simpson SJ, et al. Early respiratory infection is associated with reduced spirometry in children with cystic fibrosis. Am J Respir Crit Care Med. (2014) 190:1111–6. doi: 10.1164/rccm.201407-1277OC
25. John A, Gozdzik-Spychalska J, Durda-Masny M, Czainski W, Pawlowska N, Wlizlo J, et al. Pseudomonas aeruginosa, the type of mutation, lung function, and nutritional status in adults with cystic fibrosis. Nutrition. (2021) 89:111221. doi: 10.1016/j.nut.2021.111221
26. Kerem E, Viviani L, Zolin A, MacNeill S, Hatziagorou E, Ellemunter H, et al. Factors associated with FEV1 decline in cystic fibrosis: analysis of the ECFS patient registry. Eur Respir J. (2014) 43:125–33. doi: 10.1183/09031936.00166412
27. Gangell C, Gard S, Douglas T, Park J, de Klerk N, Keil T, et al. Inflammatory responses to individual microorganisms in the lungs of children with cystic fibrosis. Clin Infect Dis. (2011) 53:425–32. doi: 10.1093/cid/cir399
28. Garratt LW, Breuer O, Schofield CJ, McLean SA, Laucirica DR, Tirouvanziam R, et al. Changes in airway inflammation with Pseudomonas eradication in early cystic fibrosis. J Cyst Fibros. (2021) 20:941–8. doi: 10.1016/j.jcf.2020.12.015
29. Sly PD, Gangell CL, Chen L, Ware RS, Ranganathan S, Mott LS, et al. Risk factors for bronchiectasis in children with cystic fibrosis. N Engl J Med. (2013) 368:1963–70. doi: 10.1056/NEJMoa1301725
30. Stick SM, Brennan S, Murray C, Douglas T, von Ungern-Sternberg BS, Garratt LW, et al. Bronchiectasis in infants and preschool children diagnosed with cystic fibrosis after newborn screening. J Pediatr. (2009) 155:623–8.e1. doi: 10.1016/j.jpeds.2009.05.005
31. Durda-Masny M, Gozdzik-Spychalska J, John A, Czainski W, Strozewska W, Pawlowska N, et al. The determinants of survival among adults with cystic fibrosis-a cohort study. J Physiol Anthropol. (2021) 40:19. doi: 10.1186/s40101-021-00269-7
32. McColley SA, Schechter MS, Morgan WJ, Pasta DJ, Craib ML, Konstan MW. Risk factors for mortality before age 18 years in cystic fibrosis. Pediatr Pulmonol. (2017) 52:909–15. doi: 10.1002/ppul.23715
33. Douglas TA, Brennan S, Gard S, Berry L, Gangell C, Stick SM, et al. Acquisition and eradication of P. aeruginosa in young children with cystic fibrosis. Eur Respir J. (2009) 33:305–11. doi: 10.1183/09031936.00043108
34. Annual Data Report 2021. Cystic Fibrosis Foundation Patient Registry 2021 Annual Data Report. Maryland: Bethesda (2022).
35. Pittman JE, Calloway EH, Kiser M, Yeatts J, Davis SD, Drumm ML, et al. Age of Pseudomonas aeruginosa acquisition and subsequent severity of cystic fibrosis lung disease. Pediatr Pulmonol. (2011) 46:497–504. doi: 10.1002/ppul.21397
36. Jacobs DM, Ochs-Balcom HM, Noyes K, Zhao J, Leung WY, Pu CY, et al. Impact of Pseudomonas aeruginosa isolation on mortality and outcomes in an outpatient chronic obstructive pulmonary disease cohort. Open Forum Infect Dis. (2020) 7:ofz546. doi: 10.1093/ofid/ofz546
37. Domenech A, Puig C, Marti S, Santos S, Fernandez A, Calatayud L, et al. Infectious etiology of acute exacerbations in severe COPD patients. J Infect. (2013) 67:516–23. doi: 10.1016/j.jinf.2013.09.003
38. Rodrigo-Troyano A, Melo V, Marcos PJ, Laserna E, Peiro M, Suarez-Cuartin G, et al. Pseudomonas aeruginosa in chronic obstructive pulmonary disease patients with frequent hospitalized exacerbations: a prospective multicentre study. Respiration. (2018) 96:417–24. doi: 10.1159/000490190
39. Gallego M, Pomares X, Espasa M, Castaner E, Sole M, Suarez D, et al. Pseudomonas aeruginosa isolates in severe chronic obstructive pulmonary disease: characterization and risk factors. BMC Pulm Med. (2014) 14:103. doi: 10.1186/1471-2466-14-103
40. Martinez-Garcia MA, Oscullo G, Posadas T, Zaldivar E, Villa C, Dobarganes Y, et al. Pseudomonas aeruginosa and lung function decline in patients with bronchiectasis. Clin Microbiol Infect. (2021) 27:428–34. doi: 10.1016/j.cmi.2020.04.007
41. Millares L, Ferrari R, Gallego M, Garcia-Nunez M, Perez-Brocal V, Espasa M, et al. Bronchial microbiome of severe COPD patients colonised by Pseudomonas aeruginosa. Eur J Clin Microbiol Infect Dis. (2014) 33:1101–11. doi: 10.1007/s10096-013-2044-0
42. McDonnell MJ, Jary HR, Perry A, MacFarlane JG, Hester KL, Small T, et al. Non cystic fibrosis bronchiectasis: a longitudinal retrospective observational cohort study of Pseudomonas persistence and resistance. Respir Med. (2015) 109:716–26. doi: 10.1016/j.rmed.2014.07.021
43. Chalmers JD, Goeminne P, Aliberti S, McDonnell MJ, Lonni S, Davidson J, et al. The bronchiectasis severity index. An international derivation and validation study. Am J Respir Crit Care Med. (2014) 189:576–85. doi: 10.1164/rccm.201309-1575OC
44. Araujo D, Shteinberg M, Aliberti S, Goeminne PC, Hill AT, Fardon TC, et al. The independent contribution of Pseudomonas aeruginosa infection to long-term clinical outcomes in bronchiectasis. Eur Respir J. (2018) 51:1701953. doi: 10.1183/13993003.01953-2017
45. Finch S, McDonnell MJ, Abo-Leyah H, Aliberti S, Chalmers JD. A comprehensive analysis of the impact of Pseudomonas aeruginosa colonization on prognosis in adult bronchiectasis. Ann Am Thorac Soc. (2015) 12:1602–11. doi: 10.1513/AnnalsATS.201506-333OC
46. Eklof J, Sorensen R, Ingebrigtsen TS, Sivapalan P, Achir I, Boel JB, et al. Pseudomonas aeruginosa and risk of death and exacerbations in patients with chronic obstructive pulmonary disease: an observational cohort study of 22 053 patients. Clin Microbiol Infect. (2020) 26:227–34. doi: 10.1016/j.cmi.2019.06.011
47. Wang H, Ji XB, Mao B, Li CW, Lu HW, Xu JF. Pseudomonas aeruginosa isolation in patients with non-cystic fibrosis bronchiectasis: a retrospective study. BMJ Open. (2018) 8:e014613. doi: 10.1136/bmjopen-2016-014613
48. Kwok WC, Ho JCM, Tam TCC, Ip MSM, Lam DCL. Risk factors for Pseudomonas aeruginosa colonization in non-cystic fibrosis bronchiectasis and clinical implications. Respir Res. (2021) 22:132. doi: 10.1186/s12931-021-01729-5
49. Goeminne PC, Nawrot TS, Ruttens D, Seys S, Dupont LJ. Mortality in non-cystic fibrosis bronchiectasis: a prospective cohort analysis. Respir Med. (2014) 108:287–96. doi: 10.1016/j.rmed.2013.12.015
50. Rodrigo-Troyano A, Suarez-Cuartin G, Peiro M, Barril S, Castillo D, Sanchez-Reus F, et al. Pseudomonas aeruginosa resistance patterns and clinical outcomes in hospitalized exacerbations of COPD. Respirology. (2016) 21:1235–42. doi: 10.1111/resp.12825
51. Busse WW, Lemanske RF Jr., Gern JE. Role of viral respiratory infections in asthma and asthma exacerbations. Lancet. (2010) 376:826–34. doi: 10.1016/S0140-6736(10)61380-3
52. Watkinson RL, Looi K, Laing IA, Cianferoni A, Kicic A. Viral induced effects on a vulnerable epithelium; lessons learned from paediatric asthma and eosinophilic oesophagitis. Front Immunol. (2021) 12:773600.
53. Zhang Q, Illing R, Hui CK, Downey K, Carr D, Stearn M, et al. Bacteria in sputum of stable severe asthma and increased airway wall thickness. Respir Res. (2012) 13:35. doi: 10.1186/1465-9921-13-35
54. Padilla-Galo A, Olveira C, Fernandez de Rota-Garcia L, Marco-Galve I, Plata AJ, Alvarez A, et al. Factors associated with bronchiectasis in patients with uncontrolled asthma; the NOPES score: a study in 398 patients. Respir Res. (2018) 19:43. doi: 10.1186/s12931-018-0746-7
55. Dimakou K, Gousiou A, Toumbis M, Kaponi M, Chrysikos S, Thanos L, et al. Investigation of bronchiectasis in severe uncontrolled asthma. Clin Respir J. (2018) 12:1212–8. doi: 10.1111/crj.12653
56. GARDP Foundation. Global burden of bacterial antimicrobial resistance in 2019: a systematic analysis. Lancet. (2022) 399:629–55. doi: 10.1016/S0140-6736(21)02724-0
57. Fainardi V, Neglia C, Muscara M, Spaggiari C, Tornesello M, Grandinetti R, et al. Multidrug-resistant bacteria in children and adolescents with cystic fibrosis. Children. (2022) 9:1330. doi: 10.3390/children9091330
58. Hahn A, Burrell A, Fanous H, Chaney H, Sami I, Perez GF, et al. Antibiotic multidrug resistance in the cystic fibrosis airway microbiome is associated with decreased diversity. Heliyon. (2018) 4:e00795. doi: 10.1016/j.heliyon.2018.e00795
59. Gao YH, Guan WJ, Zhu YN, Chen RC, Zhang GJ. Antibiotic-resistant Pseudomonas aeruginosa infection in patients with bronchiectasis: prevalence, risk factors and prognostic implications. Int J Chron Obstruct Pulmon Dis. (2018) 13:237–46. doi: 10.2147/COPD.S150250
60. Menendez R, Mendez R, Polverino E, Rosales-Mayor E, Amara-Elori I, Reyes S, et al. Risk factors for multidrug-resistant pathogens in bronchiectasis exacerbations. BMC Infect Dis. (2017) 17:659. doi: 10.1186/s12879-017-2754-5
61. Montero M, Dominguez M, Orozco-Levi M, Salvado M, Knobel H. Mortality of COPD patients infected with multi-resistant Pseudomonas aeruginosa: a case and control study. Infection. (2009) 37:16–9. doi: 10.1007/s15010-008-8125-9
62. GBD Chronic Respiratory Disease Collaborators. Prevalence and attributable health burden of chronic respiratory diseases, 1990-2017: a systematic analysis for the Global Burden of Disease Study 2017. Lancet Respir Med. (2020) 8:585–96. doi: 10.1016/S2213-2600(20)30105-3
63. Xie M, Liu X, Cao X, Guo M, Li X. Trends in prevalence and incidence of chronic respiratory diseases from 1990 to 2017. Respir Res. (2020) 21:49. doi: 10.1186/s12931-020-1291-8
64. Craig A, Mai J, Cai S, Jeyaseelan S. Neutrophil recruitment to the lungs during bacterial pneumonia. Infect Immun. (2009) 77:568–75. doi: 10.1128/IAI.00832-08
65. Garratt LW. Current understanding of the neutrophil transcriptome in health and disease. Cells. (2021) 10:2406. doi: 10.3390/cells10092406
66. Giacalone VD, Margaroli C, Mall MA, Tirouvanziam R. Neutrophil adaptations upon recruitment to the lung: new concepts and implications for homeostasis and disease. Int J Mol Sci. (2020) 21:851. doi: 10.3390/ijms21030851
67. Margaroli C, Tirouvanziam R. Neutrophil plasticity enables the development of pathological microenvironments: implications for cystic fibrosis airway disease. Mol Cell Pediatr. (2016) 3:38. doi: 10.1186/s40348-016-0066-2
68. Rosenow T, Mok LC, Turkovic L, Berry LJ, Sly PD, Ranganathan S, et al. The cumulative effect of inflammation and infection on structural lung disease in early cystic fibrosis. Eur Respir J. (2019) 54:1801771. doi: 10.1183/13993003.01771-2018
69. Dittrich AS, Kuhbandner I, Gehrig S, Rickert-Zacharias V, Twigg M, Wege S, et al. Elastase activity on sputum neutrophils correlates with severity of lung disease in cystic fibrosis. Eur Respir J. (2018) 51:1701910. doi: 10.1183/13993003.01910-2017
70. Oriano M, Gramegna A, Terranova L, Sotgiu G, Sulaiman I, Ruggiero L, et al. Sputum neutrophil elastase associates with microbiota and Pseudomonas aeruginosa in bronchiectasis. Eur Respir J. (2020) 56:2000769. doi: 10.1183/13993003.00769-2020
71. Forrest OA, Ingersoll SA, Preininger MK, Laval J, Limoli DH, Brown MR, et al. Frontline Science: pathological conditioning of human neutrophils recruited to the airway milieu in cystic fibrosis. J Leukoc Biol. (2018) 104:665–75. doi: 10.1002/JLB.5HI1117-454RR
72. Margaroli C, Garratt LW, Horati H, Dittrich AS, Rosenow T, Montgomery ST, et al. Elastase exocytosis by airway neutrophils is associated with early lung damage in children with cystic fibrosis. Am J Respir Crit Care Med. (2019) 199:873–81. doi: 10.1164/rccm.201803-0442OC
73. Tirouvanziam R, Gernez Y, Conrad CK, Moss RB, Schrijver I, Dunn CE, et al. Profound functional and signaling changes in viable inflammatory neutrophils homing to cystic fibrosis airways. Proc Natl Acad Sci USA. (2008) 105:4335–9. doi: 10.1073/pnas.0712386105
74. Laucirica DR, Schofield CJ, McLean SA, Margaroli C, Agudelo-Romero P, Stick SM, et al. Pseudomonas aeruginosa modulates neutrophil granule exocytosis in an in vitro model of airway infection. Immunol Cell Biol. (2022) 100:352–70. doi: 10.1111/imcb.12547
75. Thulborn SJ, Mistry V, Brightling CE, Moffitt KL, Ribeiro D, Bafadhel M. Neutrophil elastase as a biomarker for bacterial infection in COPD. Respir Res. (2019) 20:170. doi: 10.1186/s12931-019-1145-4
76. Chalmers JD, Moffitt KL, Suarez-Cuartin G, Sibila O, Finch S, Furrie E, et al. Neutrophil elastase activity is associated with exacerbations and lung function decline in bronchiectasis. Am J Respir Crit Care Med. (2017) 195:1384–93. doi: 10.1164/rccm.201605-1027OC
77. Ali HA, Fouda EM, Salem MA, Abdelwahad MA, Radwan HH. Sputum neutrophil elastase and its relation to pediatric bronchiectasis severity: a cross-sectional study. Health Sci Rep. (2022) 5:e581. doi: 10.1002/hsr2.581
78. Ray A, Kolls JK. Neutrophilic inflammation in asthma and association with disease severity. Trends Immunol. (2017) 38:942–54. doi: 10.1016/j.it.2017.07.003
79. Fahy JV, Kim KW, Liu J, Boushey HA. Prominent neutrophilic inflammation in sputum from subjects with asthma exacerbation. J Allergy Clin Immunol. (1995) 95:843–52. doi: 10.1016/s0091-6749(95)70128-1
80. Vignola AM, Bonanno A, Mirabella A, Riccobono L, Mirabella F, Profita M, et al. Increased levels of elastase and alpha1-antitrypsin in sputum of asthmatic patients. Am J Respir Crit Care Med. (1998) 157:505–11. doi: 10.1164/ajrccm.157.2.9703070
81. Wark PA, Johnston SL, Moric I, Simpson JL, Hensley MJ, Gibson PG. Neutrophil degranulation and cell lysis is associated with clinical severity in virus-induced asthma. Eur Respir J. (2002) 19:68–75. doi: 10.1183/09031936.02.00226302
82. Jatakanon A, Uasuf C, Maziak W, Lim S, Chung KF, Barnes PJ. Neutrophilic inflammation in severe persistent asthma. Am J Respir Crit Care Med. (1999) 160, (5 Pt 1):1532–9. doi: 10.1164/ajrccm.160.5.9806170
83. Koga H, Miyahara N, Fuchimoto Y, Ikeda G, Waseda K, Ono K, et al. Inhibition of neutrophil elastase attenuates airway hyperresponsiveness and inflammation in a mouse model of secondary allergen challenge: neutrophil elastase inhibition attenuates allergic airway responses. Respir Res. (2013) 14:8. doi: 10.1186/1465-9921-14-8
84. Weng Q, Zhu C, Zheng K, Wu Y, Dong L, Wu Y, et al. Early recruited neutrophils promote asthmatic inflammation exacerbation by release of neutrophil elastase. Cell Immunol. (2020) 352:104101. doi: 10.1016/j.cellimm.2020.104101
85. Fujimoto K, Kubo K, Shinozaki S, Okada K, Matsuzawa Y, Kobayashi T, et al. Neutrophil elastase inhibitor reduces asthmatic responses in allergic sheep. Respir Physiol. (1995) 100:91–100. doi: 10.1016/0034-5687(94)00123-h
86. Margaroli C, Moncada-Giraldo D, Gulick DA, Dobosh B, Giacalone VD, Forrest OA, et al. Transcriptional firing represses bactericidal activity in cystic fibrosis airway neutrophils. Cell Rep Med. (2021) 2:100239. doi: 10.1016/j.xcrm.2021.100239
87. Shanmugam L, Ravinder SS, Johnson P, Padmavathi R, Rajagopalan B, Kindo AJ. Assessment of phagocytic activity of neutrophils in chronic obstructive pulmonary disease. Lung India. (2015) 32:437–40. doi: 10.4103/0970-2113.164159
88. Thomas C, Barnes P, Donnelly L. Reduced phagocytosis of pathogenic bacteria by neutrophils from COPD patients. Eur Respir J. (2012) 40, (Suppl 56):387.
89. Lavinskiene S, Vaitkus M, Bieksiene K, Jeroch J, Sakalauskas R. Neutrophil phagocytic activity in AECOPD. Eur Respir J. (2011) 38, (Suppl 55):738.
90. Bedi P, Davidson DJ, McHugh BJ, Rossi AG, Hill AT. Blood neutrophils are reprogrammed in bronchiectasis. Am J Respir Crit Care Med. (2018) 198:880–90. doi: 10.1164/rccm.201712-2423OC
91. Chalmers JD, Ma A, Turnbull K, Doherty C, Govan JR, Hill A. Impaired neutrophil phagocytosis and receptor expression in non-CF bronchiectasis. Eur Respir J. (2013) 42, (Suppl 57):2065. doi: 10.3390/biom11081065
92. da Silva-Martins CL, Couto SC, Muniz-Junqueira MI. Inhaled corticosteroid treatment for 6 months was not sufficient to normalize phagocytosis in asthmatic children. Clin Transl Allergy. (2013) 3:28. doi: 10.1186/2045-7022-3-28
93. Brinkmann V, Reichard U, Goosmann C, Fauler B, Uhlemann Y, Weiss DS, et al. Neutrophil extracellular traps kill bacteria. Science. (2004) 303:1532–5. doi: 10.1126/science.1092385
94. Fuchs TA, Abed U, Goosmann C, Hurwitz R, Schulze I, Wahn V, et al. Novel cell death program leads to neutrophil extracellular traps. J Cell Biol. (2007) 176:231–41. doi: 10.1083/jcb.200606027
95. Yousefi S, Mihalache C, Kozlowski E, Schmid I, Simon HU. Viable neutrophils release mitochondrial DNA to form neutrophil extracellular traps. Cell Death Differ. (2009) 16:1438–44. doi: 10.1038/cdd.2009.96
96. Pilsczek FH, Salina D, Poon KK, Fahey C, Yipp BG, Sibley CD, et al. A novel mechanism of rapid nuclear neutrophil extracellular trap formation in response to Staphylococcus aureus. J Immunol. (2010) 185:7413–25. doi: 10.4049/jimmunol.1000675
97. Yipp BG, Petri B, Salina D, Jenne CN, Scott BN, Zbytnuik LD, et al. Infection-induced NETosis is a dynamic process involving neutrophil multitasking in vivo. Nat Med. (2012) 18:1386–93. doi: 10.1038/nm.2847
98. Kaplan MJ, Radic M. Neutrophil extracellular traps: double-edged swords of innate immunity. J Immunol. (2012) 189:2689–95. doi: 10.4049/jimmunol.1201719
99. Delgado-Rizo V, Martinez-Guzman MA, Iniguez-Gutierrez L, Garcia-Orozco A, Alvarado-Navarro A, Fafutis-Morris M. Neutrophil extracellular traps and its implications in inflammation: an overview. Front Immunol. (2017) 8:81. doi: 10.3389/fimmu.2017.00081
100. Manzenreiter R, Kienberger F, Marcos V, Schilcher K, Krautgartner WD, Obermayer A, et al. Ultrastructural characterization of cystic fibrosis sputum using atomic force and scanning electron microscopy. J Cyst Fibros. (2012) 11:84–92. doi: 10.1016/j.jcf.2011.09.008
101. Grabcanovic-Musija F, Obermayer A, Stoiber W, Krautgartner WD, Steinbacher P, Winterberg N, et al. Neutrophil extracellular trap (NET) formation characterises stable and exacerbated COPD and correlates with airflow limitation. Respir Res. (2015) 16:59. doi: 10.1186/s12931-015-0221-7
102. Keir HR, Shoemark A, Dicker AJ, Perea L, Pollock J, Giam YH, et al. Neutrophil extracellular traps, disease severity, and antibiotic response in bronchiectasis: an international, observational, multicohort study. Lancet Respir Med. (2021) 9:873–84. doi: 10.1016/S2213-2600(20)30504-X
103. Linssen RS, Chai G, Ma J, Kummarapurugu AB, van Woensel JBM, Bem RA, et al. Neutrophil extracellular traps increase airway mucus viscoelasticity and slow mucus particle transit. Am J Respir Cell Mol Biol. (2021) 64:69–78. doi: 10.1165/rcmb.2020-0168OC
104. Marcos V, Zhou-Suckow Z, Onder Yildirim A, Bohla A, Hector A, Vitkov L, et al. Free DNA in cystic fibrosis airway fluids correlates with airflow obstruction. Mediators Inflamm. (2015) 2015:408935. doi: 10.1155/2015/408935
105. Gray RD, Hardisty G, Regan KH, Smith M, Robb CT, Duffin R, et al. Delayed neutrophil apoptosis enhances NET formation in cystic fibrosis. Thorax. (2018) 73:134–44. doi: 10.1136/thoraxjnl-2017-210134
106. Dicker AJ, Crichton ML, Pumphrey EG, Cassidy AJ, Suarez-Cuartin G, Sibila O, et al. Neutrophil extracellular traps are associated with disease severity and microbiota diversity in patients with chronic obstructive pulmonary disease. J Allergy Clin Immunol. (2018) 141:117–27. doi: 10.1016/j.jaci.2017.04.022
107. Lachowicz-Scroggins ME, Dunican EM, Charbit AR, Raymond W, Looney MR, Peters MC, et al. Extracellular DNA, neutrophil extracellular traps, and inflammasome activation in severe asthma. Am J Respir Crit Care Med. (2019) 199:1076–85. doi: 10.1164/rccm.201810-1869OC
108. Konstan MW, Doring G, Heltshe SL, Lands LC, Hilliard KA, Koker P, et al. A randomized double blind, placebo controlled phase 2 trial of BIIL 284 BS (an LTB4 receptor antagonist) for the treatment of lung disease in children and adults with cystic fibrosis. J Cyst Fibros. (2014) 13:148–55. doi: 10.1016/j.jcf.2013.12.009
109. Doring G, Bragonzi A, Paroni M, Akturk FF, Cigana C, Schmidt A, et al. BIIL 284 reduces neutrophil numbers but increases P. aeruginosa bacteremia and inflammation in mouse lungs. J Cyst Fibros. (2014) 13:156–63. doi: 10.1016/j.jcf.2013.10.007
110. Hoyle N, Zhvaniya P, Balarjishvili N, Bolkvadze D, Nadareishvili L, Nizharadze D, et al. Phage therapy against Achromobacter xylosoxidans lung infection in a patient with cystic fibrosis: a case report. Res Microbiol. (2018) 169:540–2. doi: 10.1016/j.resmic.2018.05.001
111. Lebeaux D, Merabishvili M, Caudron E, Lannoy D, Van Simaey L, Duyvejonck H, et al. A case of phage therapy against pandrug-resistant Achromobacter xylosoxidans in a 12-year-old lung-transplanted cystic fibrosis patient. Viruses. (2021) 13:60. doi: 10.3390/v13010060
112. Maddocks S, Fabijan AP, Ho J, Lin RCY, Ben Zakour NL, Dugan C, et al. Bacteriophage therapy of ventilator-associated pneumonia and empyema caused by Pseudomonas aeruginosa. Am J Respir Crit Care Med. (2019) 200:1179–81. doi: 10.1164/rccm.201904-0839LE
113. Dedrick RM, Smith BE, Cristinziano M, Freeman KG, Jacobs-Sera D, Belessis Y, et al. Phage therapy of Mycobacterium infections: compassionate-use of phages in twenty patients with drug-resistant Mycobacterial disease. Clin Infect Dis. (2022) [Online ahead of print]. doi: 10.1093/cid/ciac453.
114. Nick JA, Dedrick RM, Gray AL, Vladar EK, Smith BE, Freeman KG, et al. Host and pathogen response to bacteriophage engineered against Mycobacterium abscessus lung infection. Cell. (2022) 185:1860–74.e12. doi: 10.1016/j.cell.2022.04.024
115. Petrovic Fabijan A, Lin RCY, Ho J, Maddocks S, Ben Zakour NL, Iredell JR, et al. Safety of bacteriophage therapy in severe Staphylococcus aureus infection. Nat Microbiol. (2020) 5:465–72. doi: 10.1038/s41564-019-0634-z
116. Pabary R, Singh C, Morales S, Bush A, Alshafi K, Bilton D, et al. Antipseudomonal bacteriophage reduces infective burden and inflammatory response in murine lung. Antimicrob Agents Chemother. (2016) 60:744–51. doi: 10.1128/AAC.01426-15
117. Debarbieux L, Leduc D, Maura D, Morello E, Criscuolo A, Grossi O, et al. Bacteriophages can treat and prevent Pseudomonas aeruginosa lung infections. J Infect Dis. (2010) 201:1096–104. doi: 10.1086/651135
118. Dufour N, Delattre R, Chevallereau A, Ricard JD, Debarbieux L. Phage therapy of pneumonia is not associated with an overstimulation of the inflammatory response compared to antibiotic treatment in mice. Antimicrob Agents Chemother. (2019) 63:e00379-19. doi: 10.1128/AAC.00379-19.
119. Cafora M, Deflorian G, Forti F, Ferrari L, Binelli G, Briani F, et al. Phage therapy against Pseudomonas aeruginosa infections in a cystic fibrosis zebrafish model. Sci Rep. (2019) 9:1527. doi: 10.1038/s41598-018-37636-x
120. Metzemaekers M, Gouwy M, Proost P. Neutrophil chemoattractant receptors in health and disease: double-edged swords. Cell Mol Immunol. (2020) 17:433–50. doi: 10.1038/s41423-020-0412-0
121. Miralda I, Uriarte SM, McLeish KR. Multiple phenotypic changes define neutrophil priming. Front Cell Infect Microbiol. (2017) 7:217. doi: 10.3389/fcimb.2017.00217
122. Yamamoto K, Ahyi AN, Pepper-Cunningham ZA, Ferrari JD, Wilson AA, Jones MR, et al. Roles of lung epithelium in neutrophil recruitment during pneumococcal pneumonia. Am J Respir Cell Mol Biol. (2014) 50:253–62. doi: 10.1165/rcmb.2013-0114OC
123. Cabrini G, Rimessi A, Borgatti M, Lampronti I, Finotti A, Pinton P, et al. Role of cystic fibrosis bronchial epithelium in neutrophil chemotaxis. Front Immunol. (2020) 11:1438. doi: 10.3389/fimmu.2020.01438
124. Trend S, Chang BJ, O’Dea M, Stick SM, Kicic A, Waerp, et al. Use of a primary epithelial cell screening tool to investigate phage therapy in cystic fibrosis. Front Pharmacol. (2018) 9:1330. doi: 10.3389/fphar.2018.01330
125. Van Belleghem JD, Clement F, Merabishvili M, Lavigne R, Vaneechoutte M. Pro- and anti-inflammatory responses of peripheral blood mononuclear cells induced by Staphylococcus aureus and Pseudomonas aeruginosa phages. Sci Rep. (2017) 7:8004. doi: 10.1038/s41598-017-08336-9
126. Przerwa A, Zimecki M, Switala-Jelen K, Dabrowska K, Krawczyk E, Luczak M, et al. Effects of bacteriophages on free radical production and phagocytic functions. Med Microbiol Immunol. (2006) 195:143–50. doi: 10.1007/s00430-006-0011-4
127. Miernikiewicz P, Klopot A, Soluch R, Szkuta P, Keska W, Hodyra-Stefaniak K, et al. T4 phage tail adhesin Gp12 counteracts LPS-induced inflammation in vivo. Front Microbiol. (2016) 7:1112. doi: 10.3389/fmicb.2016.01112
128. Nelson AR. The effect of bacteriophage upon the phenomena of leukocytosis and phagocytosis. J Immunol. (1928) 15:43–64.
129. Smith GH. Bacteriophage and phagocytosis, i. effect on resistant and dead bacteria. J Immunol. (1928) 15:125–40. doi: 10.3390/pharmaceutics14091916
130. d’Herelle F, Smith GH. The Bacteriophage, its Role in Immunity. Philadelphia, PA: Williams & Wilkins (1922).
131. Tiwari BR, Kim S, Rahman M, Kim J. Antibacterial efficacy of lytic Pseudomonas bacteriophage in normal and neutropenic mice models. J Microbiol. (2011) 49:994–9. doi: 10.1007/s12275-011-1512-4
132. Singla S, Harjai K, Katare OP, Chhibber S. Encapsulation of bacteriophage in liposome accentuates its entry in to macrophage and shields it from neutralizing antibodies. PLoS One. (2016) 11:e0153777. doi: 10.1371/journal.pone.0153777
133. Broxmeyer L, Sosnowska D, Miltner E, Chacon O, Wagner D, McGarvey J, et al. Killing of Mycobacterium avium and Mycobacterium tuberculosis by a mycobacteriophage delivered by a nonvirulent mycobacterium: a model for phage therapy of intracellular bacterial pathogens. J Infect Dis. (2002) 186:1155–60. doi: 10.1086/343812
134. Kaur S, Harjai K, Chhibber S. Bacteriophage-aided intracellular killing of engulfed methicillin-resistant Staphylococcus aureus (MRSA) by murine macrophages. Appl Microbiol Biotechnol. (2014) 98:4653–61. doi: 10.1007/s00253-014-5643-5
135. Capparelli R, Parlato M, Borriello G, Salvatore P, Iannelli D. Experimental phage therapy against Staphylococcus aureus in mice. Antimicrob Agents Chemother. (2007) 51:2765–73. doi: 10.1128/AAC.01513-06
136. Jonczyk-Matysiak E, Lusiak-Szelachowska M, Klak M, Bubak B, Miedzybrodzki R, Weber-Dabrowska B, et al. The effect of bacteriophage preparations on intracellular killing of bacteria by phagocytes. J Immunol Res. (2015) 2015:482863. doi: 10.1155/2015/482863
137. Kurzepa-Skaradzinska A, Lusiak-Szelachowska M, Skaradzinski G, Jonczyk-Matysiak E, Weber-Dabrowska B, Zaczek M, et al. Influence of bacteriophage preparations on intracellular killing of bacteria by human phagocytes in vitro. Viral Immunol. (2013) 26:150–62. doi: 10.1089/vim.2012.0071
138. Hasan U, Chaffois C, Gaillard C, Saulnier V, Merck E, Tancredi S, et al. Human TLR10 is a functional receptor, expressed by B cells and plasmacytoid dendritic cells, which activates gene transcription through MyD88. J Immunol. (2005) 174:2942–50. doi: 10.4049/jimmunol.174.5.2942
139. Kawasaki T, Kawai T. Toll-like receptor signaling pathways. Front Immunol. (2014) 5:461. doi: 10.3389/fimmu.2014.00461
140. Zhao Y, van Kessel KPM, de Haas CJC, Rogers MRC, van Strijp JAG, Haas PA. Staphylococcal superantigen-like protein 13 activates neutrophils via formyl peptide receptor 2. Cell Microbiol. (2018) 20:e12941. doi: 10.1111/cmi.12941
141. Laucirica DR, Garratt LW, Kicic A. Progress in model systems of cystic fibrosis mucosal inflammation to understand aberrant neutrophil activity. Front Immunol. (2020) 11:595. doi: 10.3389/fimmu.2020.00595
142. Tanner L, Single AB. Animal models reflecting chronic obstructive pulmonary disease and related respiratory disorders: translating pre-clinical data into clinical relevance. J Innate Immun. (2020) 12:203–25. doi: 10.1159/000502489
143. Shao L, Fischer DD, Kandasamy S, Saif LJ, Vlasova AN. Tissue-specific mRNA expression profiles of porcine Toll-like receptors at different ages in germ-free and conventional pigs. Vet Immunol Immunopathol. (2016) 171:7–16. doi: 10.1016/j.vetimm.2016.01.008
144. Martinovich KM, Iosifidis T, Buckley AG, Looi K, Ling KM, Sutanto EN, et al. Conditionally reprogrammed primary airway epithelial cells maintain morphology, lineage and disease specific functional characteristics. Sci Rep. (2017) 7:17971. doi: 10.1038/s41598-017-17952-4
Keywords: bacteriophage, phage therapy, chronic airway disease, bacterial infection, neutrophilic inflammation
Citation: Laucirica DR, Stick SM, Garratt LW and Kicic A (2022) Bacteriophage: A new therapeutic player to combat neutrophilic inflammation in chronic airway diseases. Front. Med. 9:1069929. doi: 10.3389/fmed.2022.1069929
Received: 14 October 2022; Accepted: 29 November 2022;
Published: 14 December 2022.
Edited by:
Mark Willcox, University of New South Wales, AustraliaReviewed by:
Carola Venturini, The University of Sydney, AustraliaCopyright © 2022 Laucirica, Stick, Garratt and Kicic. This is an open-access article distributed under the terms of the Creative Commons Attribution License (CC BY). The use, distribution or reproduction in other forums is permitted, provided the original author(s) and the copyright owner(s) are credited and that the original publication in this journal is cited, in accordance with accepted academic practice. No use, distribution or reproduction is permitted which does not comply with these terms.
*Correspondence: Anthony Kicic, QW50aG9ueS5LaWNpY0B0ZWxldGhvbmtpZHMub3JnLmF1