- 1Department of Intensive Care, Cerrahpaşa Faculty of Medicine, Istanbul University-Cerrahpaşa, Istanbul, Turkey
- 2School of Medicine and Surgery, University of Milano-Bicocca, Monza, Italy
- 3Department of Emergency and Intensive Care, ECMO Center, ASST Monza, San Gerardo University Teaching Hospital, Monza, Italy
Background: Prone position (PP) is a recommended intervention in severe classical acute respiratory distress syndrome (ARDS). Changes in lung resting volume, respiratory mechanics and gas exchange during a 16-h cycle of PP in COVID-19 ARDS has not been yet elucidated.
Methods: Patients with severe COVID-19 ARDS were enrolled between May and September 2021 in a prospective cohort study in a University Teaching Hospital. Lung resting volume was quantitatively assessed by multiple breath nitrogen wash-in/wash-out technique to measure the end-expiratory lung volume (EELV). Timepoints included the following: Baseline, Supine Position (S1); start of PP (P0), and every 4-h (P4; P8; P12) until the end of PP (P16); and Supine Position (S2). Respiratory mechanics and gas exchange were assessed at each timepoint.
Measurements and main results: 40 mechanically ventilated patients were included. EELV/predicted body weight (PBW) increased significantly over time. The highest increase was observed at P4. The highest absolute EELV/PBW values were observed at the end of the PP (P16 vs S1; median 33.5 ml/kg [InterQuartileRange, 28.2–38.7] vs 23.4 ml/kg [18.5–26.4], p < 0.001). Strain decreased immediately after PP and remained stable between P4 and P16. PaO2/FiO2 increased during PP reaching the highest level at P12 (P12 vs S1; 163 [138–217] vs 81 [65–97], p < 0.001). EELV/PBW, strain and PaO2/FiO2 decreased at S2 although EELV/PBW and PaO2/FiO2 were still significantly higher as compared to S1. Both absolute values over time and changes of strain and PaO2/FiO2 at P16 and S2 versus S1 were strongly associated with EELV/PBW levels.
Conclusion: In severe COVID-19 ARDS, EELV steadily increased over a 16-h cycle of PP peaking at P16. Strain gradually decreased, and oxygenation improved over time. Changes in strain and oxygenation at the end of PP and back to SP were strongly associated with changes in EELV/PBW. Whether the change in EELV and oxygenation during PP may play a role on outcomes in COVID-ARDS deserves further investigation.
Clinical trial registration: [www.ClinicalTrials.gov], identifier [NCT 04818164].
Introduction
Lung protective ventilation with low tidal volumes and driving pressures (1), higher levels of positive end-expiratory pressure (PEEP) (2, 3) and prone positioning (PP) (4) are recommended treatments in classical ARDS (5, 6).
Prone positioning improves ventilation-perfusion matching and oxygenation in classical ARDS (7–9). A single PP session for at least 16 h (10, 11) or longer (12) is recommended to achieve a clinical benefit (13). These recommendations on the duration of PP sessions were mainly hinged on the PROSEVA trial (4) and a subsequent meta-analysis (14) that showed an improved survival in patients with ARDS.
Recently, Protti et al. reported that PP improved alveolar collapse, decreased hyperinflation and homogenized lung aeration also in Coronavirus disease 2019 (COVID-19) ARDS. This provides a strong rationale for its use in this scenario (15). Furthermore, Zarantonello and co-workers observed that PP induced an improvement of ventilation-perfusion matching and dorsal ventilation within the first 90 min (16). Another study established the positive role of PP on outcomes, in which shorter time to PP was associated with a decrease in mortality (17). Accordingly, COVID-19 guidelines also recommend PP in mechanically ventilated severe ARDS patients (18).
Despite the abundance of literature demonstrating the effects of PP immediately or after a short follow-up period in classical ARDS (12, 19, 20), its effect on lung volumes [i.e., end-expiratory lung volume (EELV)], respiratory mechanics and gas exchange over the recommended period, i.e., 16 h, was not previously elucidated in COVID-19 ARDS (21). Therefore, there is limited information on the optimal duration of PP in this population (22).
EELV is the Functional Residual Capacity measured in the presence of PEEP. It is dramatically reduced in ARDS (20, 23) because of loss of aeration which leads to severe hypoxemia (24). Quantitative EELV measurement can be performed by multiple breath nitrogen wash-in/wash out (NWI-WO) technique at the bedside without interrupting the mechanical ventilation. Additionally, it can accurately monitor PEEP or PP induced changes in the ARDS lung resting volume (25–27).
In this study we aimed at prospectively assessing how lung volumes, respiratory mechanics and gas exchange change before, during and after a 16-h cycle of PP in patients with COVID-19 ARDS. Furthermore, we explored whether changes in respiratory mechanics and gas exchange were correlated with changes in EELV during and after PP in this patient population.
Materials and methods
This prospective cohort, physiological study was conducted in a single-center University Teaching Hospital in a 11-bed intensive care unit (ICU). The local ethical committee (Board Name: Ministry of Health University, Istanbul Research Hospital, Clinical Studies Ethical Committee. Reference no:18, Title: Evaluating the change in the end-expiratory lung volume after prone positioning in ARDS patients, date: 14.01.2021) reviewed and approved the study. Informed consent was waived based on the observational nature of the study. Study procedures were followed in accordance with the ethical standards on human experimentation according to the Helsinki Declaration of 1975. The study was registered at www.clinicaltrials.gov as NCT 04818164. The study was reported according to the recommendations of strengthening the reporting of observational studies in epidemiology (STROBE) (28).
Patients
Inclusion criteria were as follows:
1. COVID-19 confirmed by severe acute respiratory syndrome coronavirus 2 (SARS-CoV-2) Polymerase Chain Reaction (PCR) molecular test;
2. Moderate-severe ARDS (ratio of arterial oxygen partial pressure to fractional inspired oxygen [PaO2/FiO2] < 200);
3. Intubation and mechanical ventilation.
All patients in the ICU that receive supplemental oxygen were reviewed daily by two investigators (i.e., OD, YD) whether they met the inclusion criteria.
Exclusion criteria were as follows:
1. Age < 18 years;
2. Invasive mechanical ventilation duration >12 h before study enrolment;
3. History of chronic obstructive pulmonary disease, or lung malignancies or resection;
4. Suspected or confirmed pulmonary embolism;
5. Pneumothorax or pneumomediastinum;
6. Chest drainage tubes;
7. Extra corporeal membrane oxygenation;
8. Hemodynamic instability (i.e., a cardiovascular Sequential Organ Failure Assessment [SOFA] score > 2);
9. Pregnancy.
As no previous studies evaluated the change on EELV in COVID ARDS, we assumed a rise in the PaO2/FiO2 for at least 16 mmHg after PP versus S1 to detect a two-sided significance of 0.05 and a power of 80% (29). With an effect size of 0.5 [(mean of group 1 - mean of group 2)/standard deviation of the control group], mean difference of 16 mmHg and a pooled standard deviation of 32, estimated sample size was calculated as 34 patients. Therefore, we decided to enrol a priori at least 40 patients in the study to account for possible missing data.
Enrolled patients spent 16 h in PP and were positioned back to SP as soon as they completed the cycle of PP. Measurements were performed at 7 time points: Supine (S) 1 (15 min after mechanical ventilation adjustments, immediately before turning to PP), Prone (P) 0 (15 min after turning to PP to allow stabilization), P4 (at 4 h of PP), P8 (at 8 h of PP), P12 (at 12 h of PP), P16 (at 16 h of PP) and finally S2 (15 min after turning to SP to allow stabilization; Supplementary Figure 1). Demographic data, laboratory data on the day of intubation (i.e., C-reactive protein, D-Dimer and ferritin), SOFA score, days since positive PCR confirmation of SARS-CoV-2, and variables about lung volumes, respiratory mechanics, ventilation, gas exchange and hemodynamics at all study timepoints were recorded. All patients included in the study were evaluated during their first prone position session.
Study aims
Primary aim: to prospectively assess how lung volumes, respiratory mechanics and gas exchange change before, during and after a 16-h cycle of PP in patients with COVID-19 ARDS.
Secondary aims: to explore whether changes in respiratory mechanics and gas exchange were correlated with changes in EELV during and after PP in this patient population; to evaluate whether changes in lung volumes, ventilatory variables and gas exchanges could differ based on the response in respiratory mechanics (Crs) after PP in COVID-19 ARDS patients.
In order to assess changes in lung volumes, ventilatory variables and gas exchanges based on the change in the Crs after PP we classified patients as responders versus non-responders to PP as follows:
1. As first, in each patient, arithmetic mean of Crs during PP was calculated [i.e., (sum of 5 values obtained during PP)/5).] Subsequently, the mean of Crs during PP was subtracted from the baseline Crs value (S1). If the Crs difference was greater than 0; patients were defined as responders. If the Crs difference was lower than 0; then the patients were defined as non-responders.
2. Responders were patients with an average Crs during 16 h of PP that was higher as compared with Crs at baseline (i.e., supine position at the study start, S1);
3. Non-responders were patients with an average Crs during 16 h of PP that was unchanged or lower as compared with Crs at baseline (i.e., supine position at the study start, S1).
Mechanical ventilation
Continuous infusion of endovenous anesthetic agents and rocuronium bromide was administered to set controlled mechanical ventilation. Pressure Control Ventilation with a ventilator capable to measure EELV via the NWI-WO technique (Carescape R860, General Electric, Madison, WI, USA) was used. The oxygenation goal was to keep a peripheral oxygen saturation (SpO2) 92% with a maximum of 80% FiO2. Detailed information about PEEP titration is reported in the Supplementary material. Briefly, at baseline, a range of PEEP values was applied for a pre-set duration in an incremental fashion (Supplementary Table 1). After each increment of PEEP, an automated EELV measurement was performed (30). PEEP with the highest EELV without decreasing the respiratory system compliance (Crs) was selected (i.e., OD, GYD) and applied throughout the entire study. If the PEEP level was changed for any reason after this time point, the patient was excluded from the analysis. No recruitment manoeuvres were performed between consequent PEEP levels and at the end of the PEEP setting procedure. The physician in charge of the patient was free to change the pressure control in order to match the starting tidal volume at S1.
Study procedures and measurements
After changes in the body position (from S1 to PP, or from PP to S2), patients were maintained for 15 min in the new body position. This was confirmed by reaching a flat Carbon Dioxide Output (VCO2) trend. At baseline, central venous and arterial blood samples were drawn and blood gas analyses were then immediately performed. An expiratory hold manoeuvre was performed at each timepoint before EELV measurement in order to exclude the presence of dynamic hyperinflation. Afterward, ventilation and hemodynamic parameters were collected.
The following parameters were also collected from the mechanical ventilator at each timepoint. Tidal volume (Vt), minute ventilation, inspiratory pressure above PEEP level (Pcontrol), mean airway pressure (Pmean), Plateau airway pressure (Pplat, measured after a two-second inspiratory hold), Static compliance of the respiratory system (Crs), FiO2, respiratory rate. Data acquired from the mechanical ventilator were averaged over 3 consecutive values and recorded real-time afterward. Tidal volume and minute ventilation were normalized by their respective predicted body weight (see below).
Lung Strain (31) was calculated as:
where Vt is tidal volume and EELV is end-expiratory lung volume.
Alveolar ventilation and dead space were calculated according to the formula (32, 33):
is expired minute ventilation, is carbon dioxide production per minute, PaCO2 is arterial carbon dioxide pressure (in mmHg) and is the ratio of dead space volume/tidal volume and 863 is the constant.
Ventilatory Ratio (VR) was computed from the equation:
Predicted body weight (PBW) (kg) was calculated by using patient height measured when the patient was in flat position after intubation and with the following formula (34):
Statistical analysis
Data were expressed as median [Inter Quartile Range] and mean ± Standard Error of the mean as appropriate. Mixed-effect models with Benjamini–Krieger and Yekutielli correction for repeated measures were used to explore differences over time during PP and S2 as compared to S1. A non-linear regression curve of the percentage increase in the EELV/PBW, strain and PaO2/FiO2 was fitted to visualize the cumulative increase of each parameter as compared to S1. Mean values of the replicates were fitted with the least squares regression method and no weighting. No constraints were used to fit the curve. 95% Prediction Band of this curve was reported. Additionally, non-truncated violin plots of the percentage change in the EELV, strain and PaO2/FiO2 at P16 and S2 were superimposed with individual changes to reflect the data distribution. Pearson correlation coefficient was assessed to determine the relationship of a) strain and b) PaO2/FiO2 versus EELV/PBW. As for each patient multiple time-points were explored, robust clustering was performed by using each patient as cluster variable to take into account for within patient correlation. A linear regression analysis was performed to predict the change at P16 and at S2 as compared to S1 in strain and PaO2/FiO2 based on the change in EELV/PBW. Change in the lung volume, respiratory variables and gas exchanges stratified by responders versus non-responders in Crs after PP were assessed using a 2-way ANOVA for repeated measurements. Difference in time, group and group be time interaction were reported. A two-tailed p-value < 0.05 was considered statistically significant. GraphPad Prism version 9.0 (Graphpad Software, San Diego, CA, USA) was used for the statistical analysis.
Results
A total of 43 COVID-19 ARDS patients were enrolled in the study. Three patients were excluded from the analysis for the following reasons: 1 patient had a pneumothorax after the P12 time point and was treated with a chest tube. Extra Corporeal Membrane Oxygenation was initiated in another patient after P0. In 1 patient, EELV could not be accurately estimated despite repeated attempts (i.e., difference between measurements >20%). Forty patients were included in the final analysis. Patients were included at a median of 4 h [Inter Quartile Range, 2–5 h]. Patient characteristics are shown in Table 1.
Effects of prone positioning on end-expiratory lung volume, strain and oxygenation
Absolute data of EELV, strain and oxygenation during the study period was reported in Table 2.
EELV/PBW and PaO2/FiO2 increased immediately after PP and decreased when PP was reversed (EELV/PBW P0 vs S1: 25.3 ml/kg [23.2–32.9] vs 23.4 ml/kg [18.5–26.4], p < 0.001; PaO2/FiO2 P0 vs S1: 117 [87–167] vs 81 [65–97], p = 0.002). However, both variables were higher at S2 as compared to S1 (EELV/PBW S2 vs S1: 25.9 ml/kg [20.1–31.6] vs 23.4 ml/kg [18.5–26.4], p = 0.006; PaO2/FiO2 S2 vs S1: 106 [79–138] vs 81 [65–97], p = 0.024). Strain declined rapidly after the onset of PP until P4 (P4 vs S1: 0.22 [0.19–0.27] vs 0.31 [0.26–0.39], p < 0.001). Subsequently, strain was stable until the end of PP (Table 2 and Figures 1A–C).
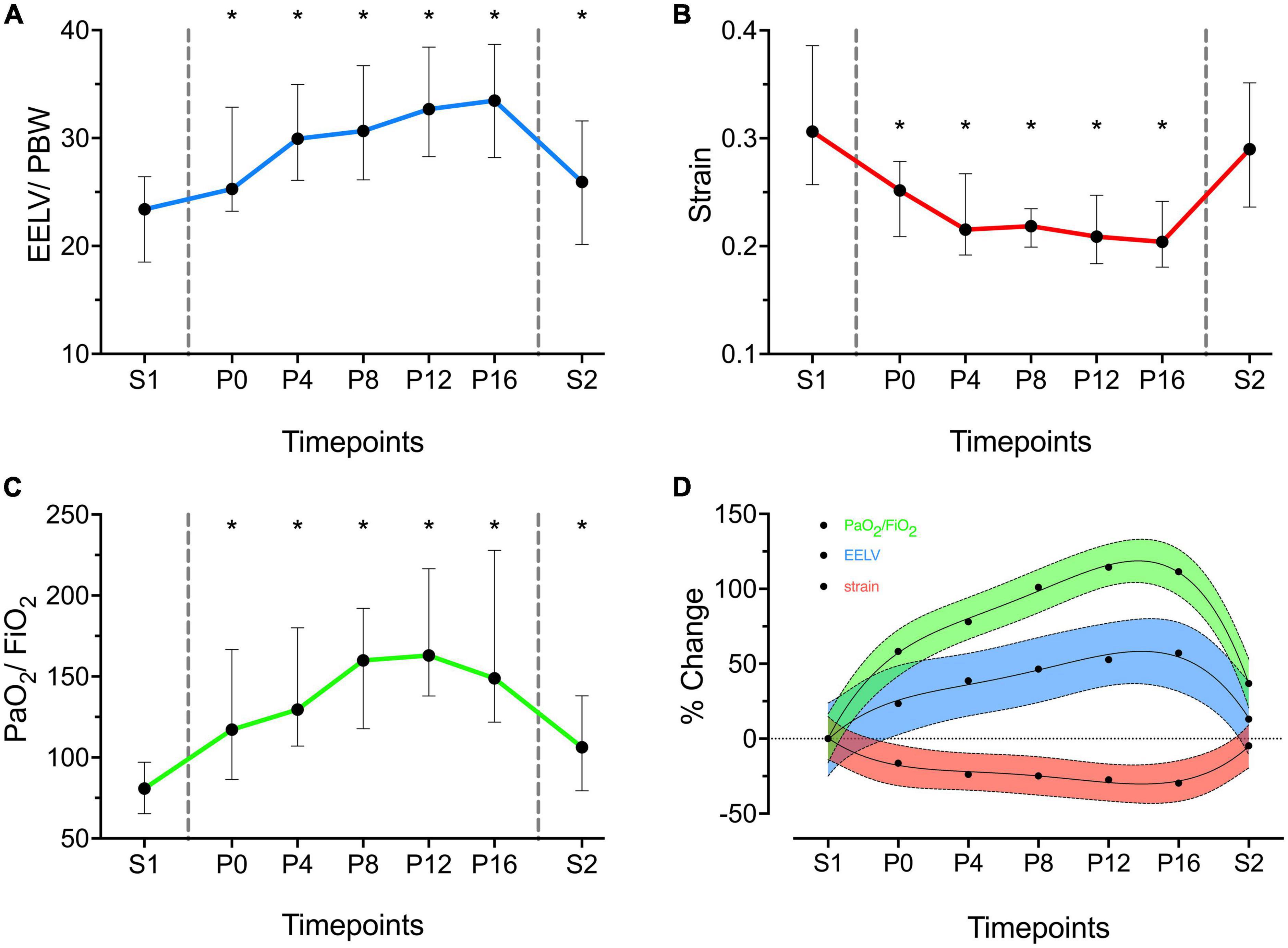
Figure 1. End-expiratory lung volume/predicted body weight (EELV/PBW – ml/kg) (A), Strain (B) and PaO2/FiO2 (C) over time. X axis represents timepoints. Y axis represents absolute values of the variables. Data are presented as median and Inter Quartile Range. Areas within vertical dashed lines indicate prone position. *Statistically significant versus S1. (D) Cumulative percentage Increase in EELV/PBW (blue), Strain (red) and PaO2/FiO2 (green) as compared to S1 (S1 = 0%). Data are presented as mean and connecting regression line. Color shaded area indicates 95% Prediction Band of the regression line.
The absolute changes over time in EELV/PBW, strain and PaO2/FiO2 were reported in Figure 1. The cumulative and relative changes over time in EELV/PBW, strain and PaO2/FiO2 were reported in Supplementary Tables 2, 3, respectively.
The cumulative percentage change in the EELV/PBW was highest at P16 (+38.6% [22.2–76.6], p < 0.001; Supplementary Table 2). The highest relative EELV/PBW increase between consecutive steps was immediately after PP (i.e., between P0 and S1) when compared to other time, intervals (P0 vs S1: +15.8% [10.2–29.3], p < 0.001). Afterward change in the EELV/PBW declined progressively with a nadir between P12 and P16 (P16 vs P12: +3.7% [-0.2 to 9], p = 0.013; Supplementary Table 3).
Similarly, the highest cumulative percentage change in PaO2/FiO2 was at P12 (+92.8 [46.5–171.6], p < 0.001; Supplementary Table 2). Its greatest relative change was immediately after PP (P0 vs S1: +23.7% [14.1–49.8], p < 0.001). After P12, PaO2/FiO2 slightly decreased (P16 vs P12: -4.7% [-15.8–8.8], p = 0.5). (Supplementary Table 3).
Association between end-expiratory lung volume versus strain and oxygenation
EELV/PBW was significantly correlated with strain and oxygenation, either in supine (2 timepoints) or in prone (5 timepoints) position (Figures 2A,B).
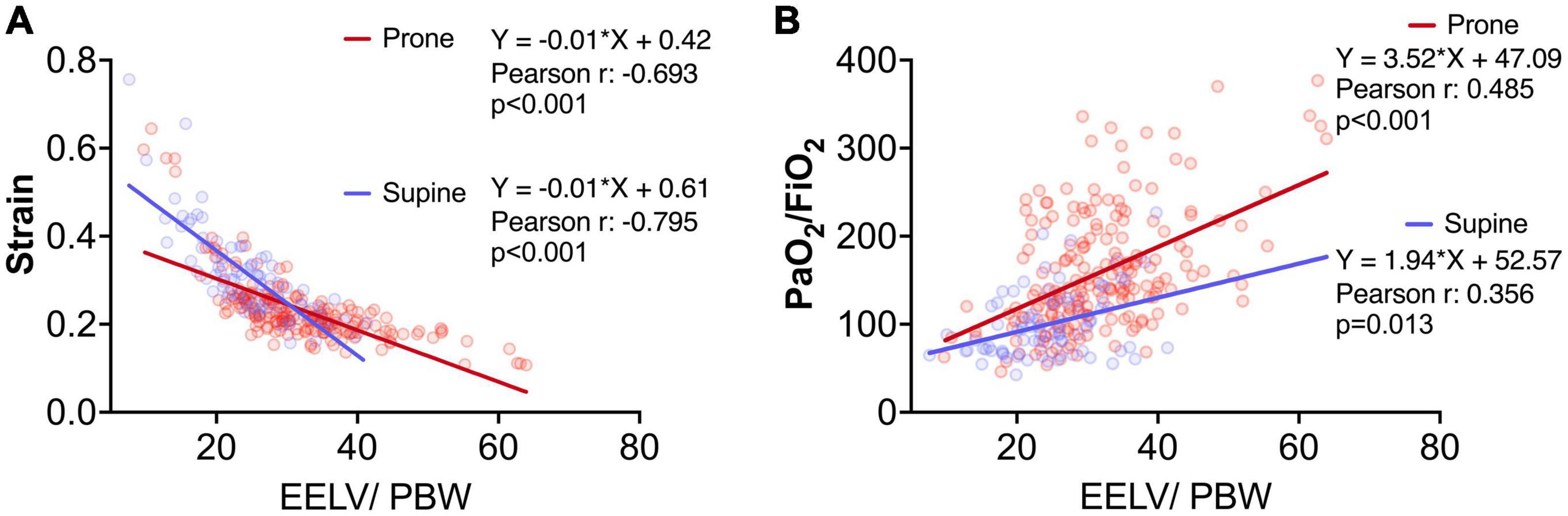
Figure 2. Association between end-expiratory lung volume/Predicted body weight (EELV/PBW – ml/kg) versus strain (A) and PaO2/FiO2 (B) (n = 280). Blue and red circles represent measurements during supine and prone position, respectively. Equations, Pearson correlation coefficient and p-value were reported. Measurements were adjusted by robust clustering for each study patient.
The changes in strain and oxygenation at the end of pronation (P16 versus S1; Figure 3A) and when patients were turned back to supine position (S2 versus S1; Figure 3B) were significantly associated with changes in EELV/PBW at the same timepoints (Figures 3C,D).
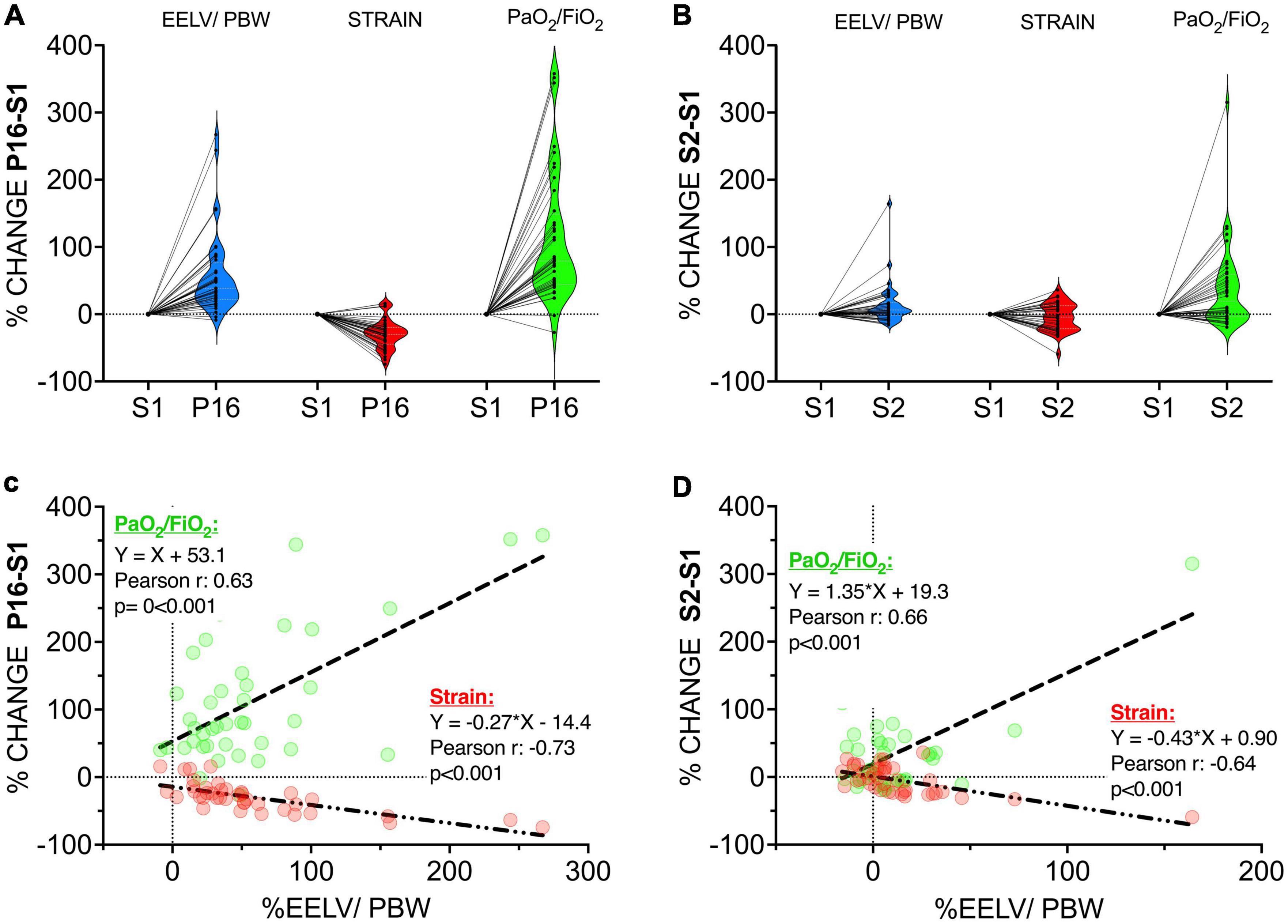
Figure 3. Upper Panel: Before-after plot of the percentage change from S1 (=%0) to P16 (end of prone position) (A) and from S1 (=%0) to S2 (B) (after turning back to supine position) in end-expiratory lung volume/Predicted Body Weight (EELV/PBW – ml/kg) (blue), strain (red), and PaO2/FiO2 (green). Dots represent individual values. Non-truncated violin plots are stacked to plot the distribution of each parameter. Lower Panel: Correlation coefficients and linear regression lines of the percentage change from S1 (=%0) to P16 (end of prone position) (C) and from S1 (=%0) to S2 (after turning back to supine position) (D) in EELV/PBW versus PaO2/FiO2 (green) and strain (red). Dashed lines represent PaO2/FiO2 and dash-dotted lines represent strain.
Changes of respiratory mechanics, gas exchange and ventilatory parameters
Crs decreased after PP (P0 vs S1: 29.5 ml/cmH2O [24.3–41.8] vs 32 ml/cmH2O [27.3–42.5], p < 0.001). Crs increased only when the PP was reversed (S2 vs S1: 35 ml/cmH2O [28.3–45] vs 32 ml/cmH2O [27.3–42.5], p = 0.84). Pplateau was increased after PP and decreased when PP was reversed (P0 vs S1: 23.5 cmH2O [21–26] vs 22.5 cmH2O [21–25.8], p = 0.068; S2 vs S1: 23.5 cmH2O [21.3- 25] vs 22.5 cmH2O [21–25.8], p = 0.67). Pcontrol was slightly increased during PP in order to maintain Vt stable. PaCO2, dead space percentage and ventilatory ratio were constant throughout the study period in the presence of a higher minute ventilation that was consequent to a higher set respiratory rate. (Table 3).
The median PEEP level during the study was 10 [8–12] cmH2O.
Metabolic parameters are presented in the Supplementary Table 4.
Lung volume, ventilatory variables and gas exchange stratified by responders versus non-responders in Crs after prone position
Nine patients out of 40 (23%) were considered responders in terms of Crs after a 16-h cycle of PP.
Mean Crs of the overall patient population during S1 was 36.7 ml/cmH20 and mean Crs of the entire PP session was 33 ml/cmH2O.
Responders had a lower driving pressure over time and a higher Vt during PP as compared with non-responders (Figures 4A–C). EELV/PBW was higher and increased more over time as compared with non-responders – and so was oxygenation; strain did not differ between the groups (Figures 4D–F).
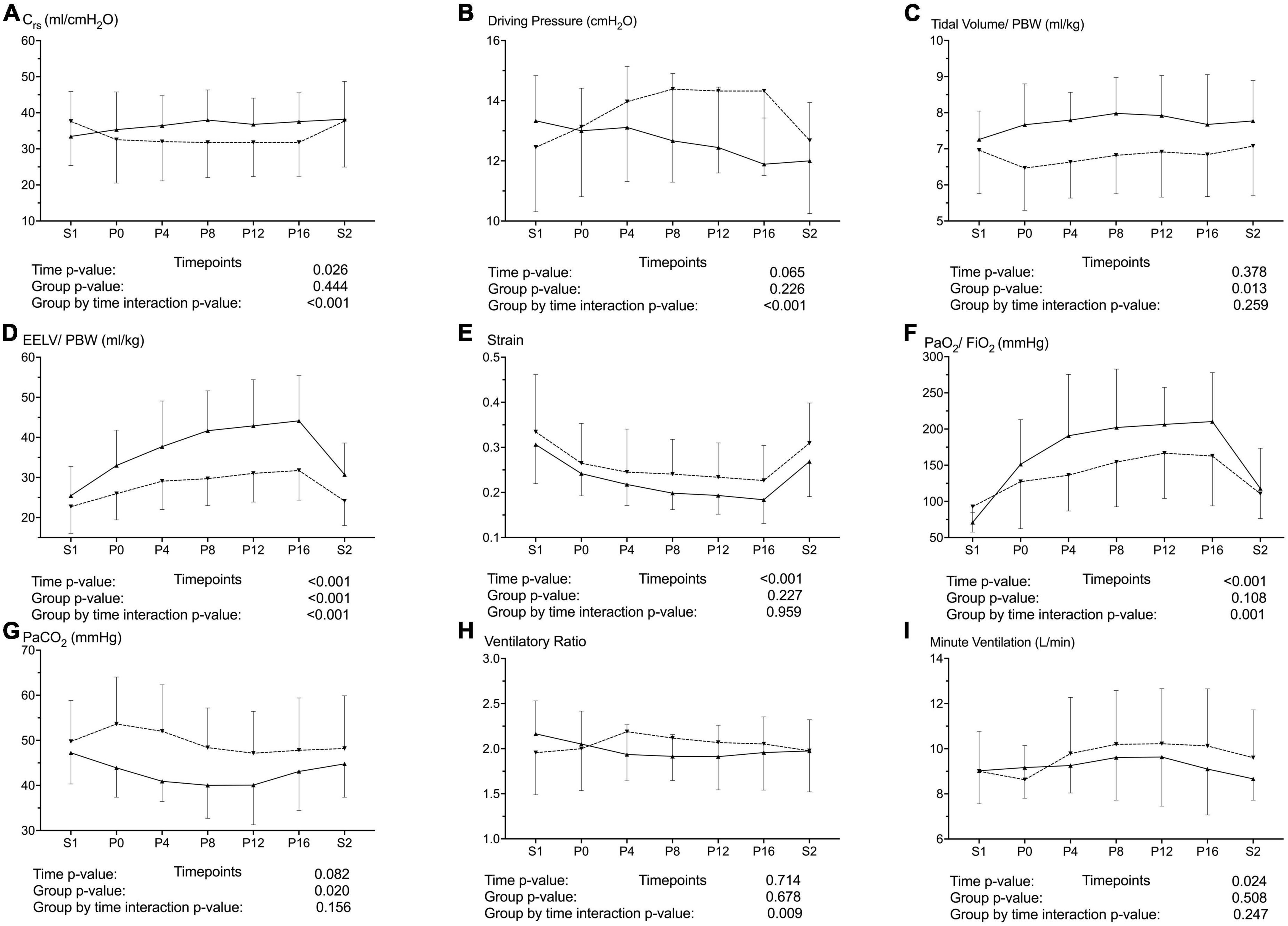
Figure 4. Changes in the resting lung volume, respiratory mechanics and gas exchange during the study time line and after stratification by the change in the respiratory system compliance (Crs) during prone position (PP) in COVID-19 ARDS patients. Upward triangles with solid lines represent increased Crs during PP (responders, n = 9) and downward triangles with dashed lines represent Decreased or Equal Crs during PP (non-responders, n = 31). P-values from the 2-way ANOVA for repeated measurements are provided below each variable. Data are presented as mean and unidirectional error bar. S1: supine position at baseline, 15 min after mechanical ventilation adjustments; P0, P4, P8, P12, P16: 15 min, 4, 8, 12 and 16 h of prone positioning; S2: 15 min after turning back to the supine position following a 16-h PP cycle. Upper Panel: Respiratory system compliance (A), Driving Pressure (B), tidal volume/Predicted body weight (PBW) (C). Mid Panel: end-expiratory lung volume/Predicted body weight (EELV/PBW) (D), Strain (E), PaO2/FiO2 (F). Lower Panel: Partial pressure of arterial carbon dioxide (PaCO2) (G), Ventilatory Ratio (H), minute ventilation (I).
Responders had a better CO2 clearance at the price of a similar minute ventilation. Consequently, ventilatory ratio decreased over time in responders as compared with non-responders (Figures 4G–I).
Discussion
In this prospective cohort study in mechanically ventilated COVID-19 ARDS patients, we report the following main findings:
1. 16-h cycle of PP increases the end-expiratory lung volume, reduces the strain and increases the oxygenation. These changes occur early and rapidly after PP;
2. the changes in EELV/PBW, strain and oxygenation quickly decreased after returning to SP, although EELV/PBW and oxygenation remained different as compared to baseline SP;
3. both changes of strain and oxygenation at the end of PP and back to SP were significantly correlated with changes in EELV/PBW;
4. COVID-19 ARDS patients with an increase in Crs after a 16h cycle of PP (i.e., responders) have a higher increase in EELV, a better oxygenation and CO2 clearance in the presence of a similar minute ventilation as compared with non-responders.
Prone position has an established survival benefit in moderate-severe ARDS. Several events, such as better distribution of ventilation-perfusion, increased gas-to-tissue ratio and increased chest wall elastance occur after switching from SP to PP (35). These transitions are crucial in reducing the stress and the strain delivered by the ventilator to the lung, which may contribute to mortality (36, 37). Cycles of ≥ 16 h of PP are recommended to minimize the exposure to potentially injurious ventilation during SP (12, 38). Previous studies evaluated the lung volume response immediately after PP or at the end of the PP duration in COVID-19 ARDS and the climax of the duration of lung volume change is unknown (15, 37, 39). We showed that EELV starts to increase rapidly after PP and peaks at the end of the 16-h cycle. Our findings support the use of PP cycle of at least 16 h as it is recommended in mechanically ventilated ARDS patients (40).
Increased lung strain and ventilation-perfusion uncoupling are directly associated with mortality (31, 41). As previously demonstrated, PP reduces non-aerated and over-aerated lung regions immediately and the inflated gas is more evenly distributed (31, 37). Furthermore, as recently reported by Zarantonello and co-workers, the ventilation-perfusion matching is improved early after PP (16). Consequently, the strain on the alveoli is reduced during the entire PP period as confirmed in our study. Therefore, PP should be used also to deliver protective mechanical ventilation.
Despite the sound evidence and rationale behind PP, it remains largely underutilized and reserved as a last resort against severe hypoxemia (42). Furthermore, the beneficial role of PP has been recently suggested in patients undergoing veno-venous ECMO (43) and in a time-dependent manner (44, 45). We observed a solid response to PP in terms of oxygenation. Although the time to peak in the PaO2/FiO2 was shorter as compared to the peak of the EELV, this response was preserved throughout the PP. The increase of oxygenation and decrease of strain at the end of PP or back to SP is consistent with the increase in the lung resting volume and this is clearly demonstrated by the robust correlation between the variables. Nevertheless, improved oxygenation after PP is weakly correlated with the decrease in the non-aerated lung regions (39, 41). In a recent study Protti et al. reported that despite an increase in oxygenation observed immediately after PP, the total gas volume of the lung was reduced in 73% of patients with COVID-19 ARDS (15). Additionally, better oxygenation response is not always associated with improved survival (4, 35). Therefore, the correlation between these two parameters may not necessarily indicate a causal relationship, at least immediately after PP.
Lung recruitment and derecruitment occur constantly during PP as a result of ongoing changes in the chest wall and lung elastance. Mainly, dorsal regions that contribute to hypoxemia are recruited while the ventral regions collapse due to the sponge like structure of the lungs and hydrostatic and gravitational forces (41, 46, 47). The net effect may differ significantly between patients and within a patient between consecutive PP sessions (12). Despite EELV improved after PP, Crs was reduced in our study. Therefore, we cannot exclude that the mild decrease in the Crs was due to alveolar overinflation despite median driving pressure was kept below 15 cmH2O through the study period (48, 49). However, the partitioned effects of PP on the chest wall and lung Compliance were not evaluated as an esophageal balloon was not available. Nonetheless, we can speculate that, although clinically negligible, decrease of the Crs may be due to the worsening of the chest wall compliance. Indeed, decreased chest wall compliance during PP as compared to supine is well described (50). However, after stratifying patients based on the change in their Crs during PP, we observed that approximately 1 out of 4 patients had an increase of Crs over time during a 16h cycle of PP as compared with baseline (S1). Moreover, patients with improved Crs had a higher EELV, a higher PaO2/FiO2 and a better CO2 clearance and compared with non-responders. Interestingly, in our study, for a similar change in minute ventilation, CO2 elimination more effective in responders. However, the definition of “Crs responders versus non-responders” is arbitrary and we cannot exclude that some patients in the non-responder group may have had an increase in lung compliance despite a more significant decrease in the chest-wall compliance resulting in an overall decrease of Crs (i.e., non-responders).
We acknowledge that our study has some limitations. We only focused our attention on the first PP session. Additionally, it may be argued that the time spent in PP was limited and extended durations may further contribute to lung recruitment. However, we believe that this phenomenon would be minimal as considered that after 12 h, the gain in EELV was negligible and the strain and oxygenation were stable. While it is possible that extended PP may further improve the EELV when the patient is back to SP after a prolonged PP session, its clinical relevance is questionable and its beneficial role is debated (51). Thirdly, the dead space estimation was burdened by the use of Heat and Moisture Exchange filters as active humidifiers were not available in our unit during the COVID pandemic. Fourth, partitioned respiratory mechanics could not be assessed as the esophageal balloon was not available. As last, advanced experimental techniques of lung imaging such as electrical impedance tomography (52) were not available, therefore the redistribution of the tidal ventilation due to the increase in the resting lung volume during PP versus SP could not be assessed.
- In conclusion, early PP rapidly increases the end-expiratory lung volume, decreases the lung strain and leads to a higher oxygenation in COVID-19 ARDS. Changes in the strain and PaO2/FiO2 are robustly correlated with the change in the EELV. A higher respiratory system compliance during PP may suggest an increased EELV and better gas exchange. Future studies should evaluate whether the change in lung volume may be maintained over a longer period of time and whether the increase in EELV during PP may predict a favorable outcome in patients with COVID-19 ARDS.
Data availability statement
The original contributions presented in this study are included in the article/Supplementary material, further inquiries can be directed to the corresponding author.
Ethics statement
The studies involving human participants were reviewed and approved by Ministry of Health University, Istanbul Research Hospital, Clinical Studies Ethical Committee. Reference no: 18, Title: Evaluating the change in the end-expiratory lung volume after prone positioning in ARDS patients, date: 14.01.2021. The ethics committee waived the requirement of written informed consent for participation.
Author contributions
ODi designed the work and drafted the first manuscript. ER revised the first draft, analysed, and interpreted the data. ODi, ODe, GY, SÜ, and YD were the major contributor to acquire and interpret the data. All authors contributed to the writing of the manuscript, read, and approved the final version of the manuscript.
Funding
This research did not receive any specific grant from funding agencies in the public, commercial, or not-for-profit sectors. ER was supported by the Bicocca Starting grant 2020 from the University of Milano-Bicocca with the project titled: “Functional Residual Capacity Assessment using a Wash-In/Wash-Out technique based on a fast main-stream O2 Sensor with nanofluorescenT geometry for severe lung injury (FAST) – COVID and beyond”.
Conflict of interest
The authors declare that the research was conducted in the absence of any commercial or financial relationships that could be construed as a potential conflict of interest.
Publisher’s note
All claims expressed in this article are solely those of the authors and do not necessarily represent those of their affiliated organizations, or those of the publisher, the editors and the reviewers. Any product that may be evaluated in this article, or claim that may be made by its manufacturer, is not guaranteed or endorsed by the publisher.
Supplementary material
The Supplementary Material for this article can be found online at: https://www.frontiersin.org/articles/10.3389/fmed.2022.1056766/full#supplementary-material
Abbreviations
ARDS, acute respiratory distress syndrome; COVID-19, Coronavirus disease 2019; Crs, respiratory system compliance; EELV, end-expiratory lung volume; MV, minute ventilation; NWI-WO, nitrogen wash-in/wash-out; PaCO2, arterial carbon dioxide pressure; PaO2/FiO2, ratio of arterial oxygenation partial pressure to fractional inspired oxygen; PBW, predicted body weight; PCR, polymerase chain reaction; PEEP, positive end-expiratory pressure; PP, prone position; SARS-CoV-2, severe acute respiratory distress syndrome coronavirus 2; SOFA, sequential organ failure assessment; SP, supine position; VCO2, carbon dioxide output; , carbon dioxide output per minute; VD/VT, ratio of dead space volume/tidal volume; , expired minute ventilation volume; VR, ventilatory ratio; Vt, tidal volume.
References
1. Amato MBP, Meade MO, Slutsky AS, Brochard L, Costa ELV, Schoenfeld DA, et al. Driving pressure and survival in the acute respiratory distress syndrome. N Engl J Med. (2015) 372:747–55. doi: 10.1056/NEJMsa1410639
2. Rezoagli E, Bellani G. How I set up positive end-expiratory pressure: evidence – and physiology-based! Crit Care. (2019) 23:412. doi: 10.1186/s13054-019-2695-z
3. Maddali MV, Churpek M, Pham T, Rezoagli E, Zhuo H, Zhao W, et al. Validation and utility of ARDS subphenotypes identified by machine-learning models using clinical data: an observational, multicohort, retrospective analysis. Lancet Respir Med. (2022) 10:367–77. doi: 10.1016/S2213-2600(21)00461-6
4. Guérin C, Reignier J, Richard JC, Beuret P, Gacouin A, Boulain T, et al. Prone positioning in severe acute respiratory distress syndrome. N Engl J Med. (2013) 368:2159–68. doi: 10.1056/NEJMoa1214103
5. Scholten EL, Beitler JR, Prisk GK, Malhotra A. Treatment of ARDS With Prone Positioning. Chest. (2017) 151:215–24. doi: 10.1016/j.chest.2016.06.032
6. Del Sorbo L, Goligher EC, McAuley DF, Rubenfeld GD, Brochard LJ, Gattinoni L, et al. Mechanical ventilation in adults with acute respiratory distress syndrome. summary of the experimental evidence for the clinical practice guideline. Ann Am Thorac Soc. (2017) 14(Suppl_4):S261–70. doi: 10.1513/AnnalsATS.201704-345OT
7. Pelosi P, Tubiolo D, Mascheroni D, Vicardi P, Crotti S, Valenza F, et al. Effects of the prone position on respiratory mechanics and gas exchange during acute lung injury. Am J Respir Crit Care Med. (1998) 157:387–93. doi: 10.1164/ajrccm.157.2.97-04023
8. Papazian L, Munshi L, Guérin C. Prone position in mechanically ventilated patients. Intensive Care Med. (2022) 48:1062–5. doi: 10.1007/s00134-022-06731-z
9. Gattinoni L, Taccone P, Carlesso E, Marini JJ. Prone position in acute respiratory distress syndrome. rationale, indications, and limits. Am J Respir Crit Care Med. (2013) 188:1286–93. doi: 10.1164/rccm.201308-1532CI
10. Abroug F, Ouanes-Besbes L, Elatrous S, Brochard L. The effect of prone positioning in acute respiratory distress syndrome or acute lung injury: a meta-analysis. Areas of uncertainty and recommendations for research. Intensive Care Med. (2008) 34:1002. doi: 10.1007/s00134-008-1062-3
11. Papazian L, Aubron C, Brochard L, Chiche JD, Combes A, Dreyfuss D, et al. Formal guidelines: management of acute respiratory distress syndrome. Ann Intensive Care. (2019) 9:69. doi: 10.1186/s13613-019-0540-9
12. Jochmans S, Mazerand S, Chelly J, Pourcine F, Sy O, Thieulot-Rolin N, et al. Duration of prone position sessions: a prospective cohort study. Ann Intensive Care. (2020) 10:66. doi: 10.1186/s13613-020-00683-7
13. Sud S, Friedrich JO, Taccone P, Polli F, Adhikari NKJ, Latini R, et al. Prone ventilation reduces mortality in patients with acute respiratory failure and severe hypoxemia: systematic review and meta-analysis. Intensive Care Med. (2010) 36:585–99. doi: 10.1007/s00134-009-1748-1
14. Munshi L, Del Sorbo L, Adhikari NKJ, Hodgson CL, Wunsch H, Meade MO, et al. Prone position for acute respiratory distress syndrome. a systematic review and meta-analysis. Ann Am Thorac Soc. (2017) 14(Suppl_4):S280–8. doi: 10.1513/AnnalsATS.201704-343OT
15. Protti A, Santini A, Pennati F, Chiurazzi C, Ferrari M, Iapichino GE, et al. Lung response to prone positioning in mechanically-ventilated patients with COVID-19. Crit Care. (2022) 26:127. doi: 10.1186/s13054-022-03996-0
16. Zarantonello F, Sella N, Pettenuzzo T, Andreatta G, Calore A, Dotto D, et al. Early physiologic effects of prone positioning in COVID-19 acute respiratory distress syndrome. Anesthesiology. (2022) 137:327–39. doi: 10.1097/ALN.0000000000004296
17. Camporota L, Sanderson B, Chiumello D, Terzi N, Argaud L, Rimmelé T, et al. Prone Position in COVID-19 and -COVID-19 acute respiratory distress syndrome: an international multicenter observational comparative study. Crit Care Med. (2022) 50:633–43. doi: 10.1097/CCM.0000000000005354
18. Fan E, Beitler JR, Brochard L, Calfee CS, Ferguson ND, Slutsky AS, et al. COVID-19-associated acute respiratory distress syndrome: is a different approach to management warranted? Lancet Respir Med. (2020) 8:816–21. doi: 10.1016/S2213-2600(20)30304-0
19. Reutershan J, Schmitt A, Dietz K, Unertl K, Fretschner R. Alveolar recruitment during prone position: time matters. Clin Sci. (2006) 110:655–63. doi: 10.1042/CS20050337
20. Aguirre-Bermeo H, Turella M, Bitondo M, Grandjean J, Italiano S, Festa O, et al. Lung volumes and lung volume recruitment in ARDS: a comparison between supine and prone position. Ann Intensive Care. (2018) 8:25. doi: 10.1186/s13613-018-0371-0
21. Rezoagli E, Magliocca A, Bellani G, Pesenti A, Grasselli G. Development of a critical care response – experiences from italy during the coronavirus disease 2019 pandemic. Anesthesiol Clin. (2021) 39:265–84. doi: 10.1016/j.anclin.2021.02.003
22. Rezoagli E, Mariani I, Rona R, Foti G, Bellani G. Difference between prolonged versus standard duration of prone position in COVID-19 patients: a retrospective study. Minerva Anestesiol. (2021) 87:1383–5. doi: 10.23736/S0375-9393.21.15864-X
23. Rezoagli E, Laffey JG, Bellani G. Monitoring lung injury severity and ventilation intensity during mechanical ventilation. Semin Respir Crit Care Med. (2022) 43:346–68. doi: 10.1055/s-0042-1748917
24. Rezoagli E, Fumagalli R, Bellani G. Definition and epidemiology of acute respiratory distress syndrome. Ann Transl Med. (2017) 5:282. doi: 10.21037/atm.2017.06.62
25. Gommers D. Functional residual capacity and absolute lung volume. Curr Opin Crit Care. (2014) 20:347–51. doi: 10.1097/MCC.0000000000000099
26. Dellamonica J, Lerolle N, Sargentini C, Beduneau G, Di Marco F, Mercat A, et al. PEEP-induced changes in lung volume in acute respiratory distress syndrome. Two methods to estimate alveolar recruitment. Intensive Care Med. (2011) 37:1595. doi: 10.1007/s00134-011-2333-y
27. Dellamonica J, Lerolle N, Sargentini C, Beduneau G, Di Marco F, Mercat A, et al. Accuracy and precision of end-expiratory lung-volume measurements by automated nitrogen washout/washin technique in patients with acute respiratory distress syndrome. Crit Care. (2011) 15:R294. doi: 10.1186/cc10587
28. von Elm E, Altman DG, Egger M, Pocock SJ, Gøtzsche PC, Vandenbroucke JP, et al. The Strengthening the Reporting of Observational Studies in Epidemiology (STROBE) statement: guidelines for reporting observational studies. J Clin Epidemiol. (2008) 61:344–9.
29. Coppo A, Bellani G, Winterton D, Di Pierro M, Soria A, Faverio P, et al. Feasibility and physiological effects of prone positioning in non-intubated patients with acute respiratory failure due to COVID-19 (PRON-COVID): a prospective cohort study. Lancet Respir Med. (2020) 8:765–74. doi: 10.1016/S2213-2600(20)30268-X
30. Olegård C, Söndergaard S, Houltz E, Lundin S, Stenqvist O. Estimation of functional residual capacity at the bedside using standard monitoring equipment: a modified nitrogen washout/washin technique requiring a small change of the inspired oxygen fraction. Anesth Analg. (2005) 101:206–12. doi: 10.1213/01.ANE.0000165823.90368.55
31. Protti A, Andreis DT, Monti M, Santini A, Sparacino CC, Langer T, et al. Lung stress and strain during mechanical ventilation: any difference between statics and dynamics? Crit Care Med. (2013) 41:1046–55.
32. Stickland MK, Neder JA, Guenette JA, O’Donnell DE, Jensen D. Using cardiopulmonary exercise testing to understand dyspnea and exercise intolerance in respiratory disease. CHEST. (2022) 161:1505–16.
33. Charron C, Repesse X, Bouferrache K, Bodson L, Castro S, Page B, et al. PaCO2 and alveolar dead space are more relevant than PaO2/FiO2 ratio in monitoring the respiratory response to prone position in ARDS patients: a physiological study. Crit Care. (2011) 15:R175. doi: 10.1186/cc10324
34. Acute Respiratory Distress Syndrome Network, Brower RG, Matthay MA, Morris A, Schoenfeld D, Thompson BT, et al. Ventilation with lower tidal volumes as compared with traditional tidal volumes for acute lung injury and the acute respiratory distress syndrome. N Engl J Med. (2000) 342:1301–8.
35. Gattinoni L, Camporota L, Marini JJ. Prone position and COVID-19: mechanisms and effects. Crit Care Med. (2022) 50:873–5.
36. Gattinoni L, Marini JJ, Collino F, Maiolo G, Rapetti F, Tonetti T, et al. The future of mechanical ventilation: lessons from the present and the past. Crit Care. (2017) 21:183.
37. Fossali T, Pavlovsky B, Ottolina D, Colombo R, Basile MC, Castelli A, et al. Effects of prone position on lung recruitment and ventilation-perfusion matching in patients With COVID-19 acute respiratory distress syndrome: a combined CT Scan/Electrical Impedance Tomography Study. Crit Care Med. (2022) 50:723–32. doi: 10.1097/CCM.0000000000005450
38. Guerin C, Baboi L, Richard JC. Mechanisms of the effects of prone positioning in acute respiratory distress syndrome. Intensive Care Med. (2014) 40:1634–42.
39. Rossi S, Palumbo MM, Sverzellati N, Busana M, Malchiodi L, Bresciani P, et al. Mechanisms of oxygenation responses to proning and recruitment in COVID-19 pneumonia. Intensive Care Med. (2022) 48:56–66. doi: 10.1007/s00134-021-06562-4
40. Fan E, Del Sorbo L, Goligher EC, Hodgson CL, Munshi L, Walkey AJ, et al. An official American Thoracic Society/European Society of Intensive Care Medicine/Society of Critical Care Medicine clinical practice guideline: mechanical ventilation in adult patients with acute respiratory distress syndrome. Am J Respir Crit Care Med. (2017) 195:1253–63.
41. Spinelli E, Kircher M, Stender B, Ottaviani I, Basile MC, Marongiu I, et al. Unmatched ventilation and perfusion measured by electrical impedance tomography predicts the outcome of ARDS. Crit Care. (2021) 25:192. doi: 10.1186/s13054-021-03615-4
42. Duggal A, Rezoagli E, Pham T, McNicholas BA, Fan E, Bellani G, et al. Patterns of use of adjunctive therapies in patients with early moderate to severe ARDS: insights from the LUNG SAFE Study. Chest. (2020) 157:1497–505.
43. Giani M, Rezoagli E, Guervilly C, Rilinger J, Duburcq T, Petit M, et al. Prone positioning during venovenous extracorporeal membrane oxygenation for acute respiratory distress syndrome: a pooled individual patient data analysis. Crit Care. (2022) 26:8.
44. Le Terrier C, Sigaud F, Lebbah S, Desmedt L, Hajage D, Guérin C, et al. Early prone positioning in acute respiratory distress syndrome related to COVID-19: a propensity score analysis from the multicentric cohort COVID-ICU network-the ProneCOVID study. Crit Care Lond Engl. (2022) 26:71. doi: 10.1186/s13054-022-03949-7
45. Giani M, Rezoagli E, Guervilly C, Rilinger J, Duburcq T, Petit M, et al. Timing of prone positioning during venovenous extracorporeal membrane oxygenation for acute respiratory distress syndrome. Crit Care Med. (2022) 50:873–5. doi: 10.1097/CCM.0000000000005705
46. Dakin J, Jones AT, Hansell DM, Hoffman EA, Evans TW. Changes in lung composition and regional perfusion and tissue distribution in patients with ARDS. Respirology. (2011) 16:1265–72. doi: 10.1111/j.1440-1843.2011.02048.x
47. Richter T, Bellani G, Harris RS, Melo MFV, Winkler T, Venegas JG, et al. Effect of prone position on regional shunt, aeration, and perfusion in experimental acute lung injury. Am J Respir Crit Care Med. (2005) 172:480–7. doi: 10.1164/rccm.200501-004OC
48. Fengmei G, Jin C, Songqiao L, Congshan Y, Yi Y. Dead space fraction changes during PEEP Titration following lung recruitment in patients with ARDS. Respir Care. (2012) 57:1578–85. doi: 10.4187/respcare.01497
49. Gogniat E, Ducrey M, Dianti J, Madorno M, Roux N, Midley A, et al. Dead space analysis at different levels of positive end-expiratory pressure in acute respiratory distress syndrome patients. J Crit Care. (2018) 45:231–8.
50. Guérin C, Albert RK, Beitler J, Gattinoni L, Jaber S, Marini JJ, et al. Prone position in ARDS patients: why, when, how and for whom. Intensive Care Med. (2020) 46:2385–96. doi: 10.1007/s00134-020-06306-w
51. Page DB, Vijaykumar K, Russell DW, Gandotra S, Chiles JW, Whitson MR, et al. Prolonged Prone Positioning for COVID-19–induced acute respiratory distress syndrome: a randomized pilot clinical trial. Ann Am Thorac Soc. (2022) 19:685–7. doi: 10.1513/AnnalsATS.202104-498RL
Keywords: acute respiratory distress syndrome, COVID-19, prone position, end-expiratory lung volume, oxygenation, nitrogen washin/washout
Citation: Dilken O, Rezoagli E, Yartaş Dumanlı G, Ürkmez S, Demirkıran O and Dikmen Y (2022) Effect of prone positioning on end-expiratory lung volume, strain and oxygenation change over time in COVID-19 acute respiratory distress syndrome: A prospective physiological study. Front. Med. 9:1056766. doi: 10.3389/fmed.2022.1056766
Received: 29 September 2022; Accepted: 21 November 2022;
Published: 02 December 2022.
Edited by:
Jihad Mallat, Cleveland Clinic Abu Dhabi, United Arab EmiratesReviewed by:
Francesca Dalla Corte, Humanitas Research Hospital, ItalyCarmen Silvia Valente Barbas, University of São Paulo, Brazil
Copyright © 2022 Dilken, Rezoagli, Yartaş Dumanlı, Ürkmez, Demirkıran and Dikmen. This is an open-access article distributed under the terms of the Creative Commons Attribution License (CC BY). The use, distribution or reproduction in other forums is permitted, provided the original author(s) and the copyright owner(s) are credited and that the original publication in this journal is cited, in accordance with accepted academic practice. No use, distribution or reproduction is permitted which does not comply with these terms.
*Correspondence: Olcay Dilken, b2xjYXlkaWxrZW5AZ21haWwuY29t
†These authors have contributed equally to this work