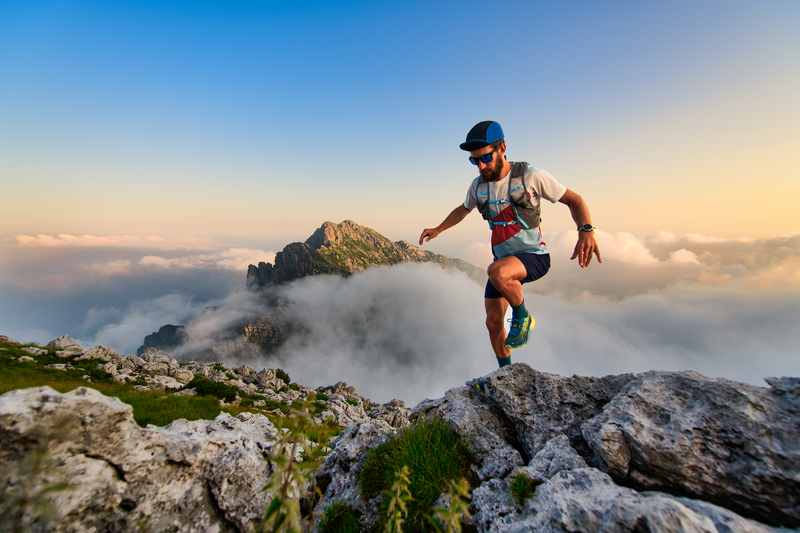
94% of researchers rate our articles as excellent or good
Learn more about the work of our research integrity team to safeguard the quality of each article we publish.
Find out more
REVIEW article
Front. Med. , 14 October 2022
Sec. Nuclear Medicine
Volume 9 - 2022 | https://doi.org/10.3389/fmed.2022.1026083
This article is part of the Research Topic ImmunoPET Imaging in Disease Diagnosis and Therapy Assessment View all 6 articles
Radioimmunoconjugates have been used for over 30 years in nuclear medicine applications. In the last few years, advances in cancer biology knowledge have led to the identification of new molecular targets specific to certain patient subgroups. The use of these targets in targeted therapies approaches has allowed the developments of specifically tailored therapeutics for patients. As consequence of the PET-imaging progresses, nuclear medicine has developed powerful imaging tools, based on monoclonal antibodies, to in vivo characterization of these tumor biomarkers. This imaging modality known as immuno-positron emission tomography (immuno-PET) is currently in fastest-growing and its medical value lies in its ability to give a non-invasive method to assess the in vivo target expression and distribution and provide key-information on the tumor targeting. Currently, immuno-PET presents promising probes for different nuclear medicine topics as staging/stratification tool, theranostic approaches or predictive/prognostic biomarkers. To develop a radiopharmaceutical drug that can be used in immuno-PET approach, it is necessary to find the best compromise between the isotope choice and the immunologic structure (full monoclonal antibody or derivatives). Through some clinical applications, this paper review aims to discuss the most important aspects of the isotope choice and the usable proteic structure that can be used to meet the clinical needs.
The original idea of personalized and tailored drugs, highly specific to a pharmacological target, appeared at the beginning of the XXth century via the “magic bullet” concept (1) brought by Paul Ehrlich (Nobel laureate in 1908). In oncologic field, this postulate aims to optimize the risk-benefit ratio for the patients care. Yet, it was not until the late 1970's that the discovery of hybridoma technology by Georges Kohler and Cesar Milstein (Nobel laureates in 1984) made it possible to apply this theory in clinical practice. The hybridoma technology allowed biochemists and immunologists to produce monoclonal antibodies (mAbs) which specifically recognize antigens on pathologic cells, thereby providing the proof-of-concept for specific immunotherapeutic approaches to treat cancer (2).
With the concomitant progress in nuclear medicine, the radiolabeled monoclonal antibody (mAb) quickly emerged to specifically target antigens of abnormal cells, most often cancerous. The discovery of overexpressed antigens in many hematologic and solid tumors paved the way for the use of gamma emitters such as iodine-131 (131I) radiolabeled mAbs for tumor detection in nuclear medicine practice (3, 4). In parallel, iodine-131 being also a beta minus emitter, the use of 131I-mAbs has quickly found its place in therapeutic approaches, mainly in hematologic neoplasms with real efficacy in B-cell lymphoma (5). For almost three decades, mAbs have been labeled with gamma emitters (such 131I or 111In) in planar or Single Photon Emission Computed Tomography (SPECT) imaging procedures. Despite the reliable and confident clinical information, this modality suffers from several drawbacks including poor sensitivity, poor spatial resolution and complex scatter correction due to the collimator.
While Positron Emission Tomography (PET) was revolutionizing diagnostic applications of nuclear medicine with small organic molecules such as fluorodeoxyglucose (FDG) radiolabeled with fluorine-18 (18F), PET approach seemed unusable with long pharmacokinetic half-life mAbs. The availability in the early 2000s (6–8) of longer half-life isotopes such as iodine-124, copper-64 or zirconium-89 allowed the fruitful association between the high specificity of mAbs with the high resolution of PET leading to the Immuno-PET approach. Comparatively to classical SPECT modality, using of immuno-PET imaging combined several technical advantages such as precise scatter correction, exact correction of attenuation, accurate quantitative information, improved spatial resolution with a better delineation of tumors and organs, and, last but not least, higher sensitivity associated with the capacity to perform a true whole-body imaging in a reasonable time. The recent technological evolution in new PET detectors and reconstruction algorithms (9–11) appeared to be also a key factor for the immuno-PET clinical performance in terms of both spatial resolution and signal-to-noise ratio.
Today, the overall performance of the immuno-PET methodology is carried by different biological or imaging parameters such target accessibility or imaging protocol (time between injection and image acquisition, number of acquisitions). The aim of this paper review is more focused on the pharmacological choices to find the best matching between the radionuclide parameters (physical, chemical, logistic) and the mAb used (specificity, affinity, dose, mAb derivative, pharmacokinetic…). Immuno-PET is a fast-growing approach (12) in many cancer pathologies (13). Currently, from an imaging point of view, the management of patients suffering from a tumor pathology is mainly driven on an initial diagnosis made by conventional techniques combining CT/MRI and 18F-FDG PET. In this care management, immuno-PET approach finds a particularly effective place to provide some specific information on the tumor phenotype, intra- and inter-tumor heterogeneity and as prognostic/predictive indicator for targeted therapies. It should be noted that in the specific case, where the immuno-PET can be used to select only patients in whom the treatment is likely to provide a benefit, the immuno-PET falls into the scope of companion diagnostic. Some preclinical proof-of-concept will be discussed in mAb derivatives section and a clinical part will illustrate, through some typical examples of results obtained with compounds (mAb and immunological derivatives) allowing antigen targeting in solid tumors, hematological and tumor microenvironment.
One of the immuno-PET key success is based on an appropriate matching between the biological half-life of the immunoprotein and the physical half-life of the isotope to achieve the best contrast in pathologic tissues (14–16). Despite the great variety of radionuclides which are positron emitters, only few of them could be used in immuno-PET. For nuclear medicine applications, the choice of the radionuclide is basically based on three main criteria: physical characteristics, chemical characteristics, and production/logistic. The list of isotopes used (or considered) is summarized in Table 1. Indeed, intact mAbs have a pharmacokinetic half-life of several days with a long circulation time and required a long radionuclide half-life to increase the tumor-to-background ratio such as iodine-124 or zirconium-89. Copper-64 with its intermediate half-life can be used for labeling a large size range of molecules like native mAbs or their smaller derivatives [F(ab')2, F(ab), minibody, ScFv, nanobody or affibody structures]. Fluorine-18 and gallium-68 with their shorter half-life may be used to label small molecular weight molecules such as mAb derivates or small synthetic molecules for pretargeting approach (15).
Several additional considerations must also be taken to rationalize the choice of a positron emitter. Positron energy range is a key factor for intrinsic resolution of PET modality because it has a direct and significant impact on the positron travel distance before annihilation. As a consequence, a high-energy positron will result in an intrinsic resolution loss. In addition, existence of concomitant γ and/or β− emissions will have a major impact on the staff and/or patient radiation dose. It should be noted, to our knowledge, they are currently no allowed maximal recommended activity limitation per isotopes. Dosimetric studies are on progress and first results obtained with trastuzumab showed an effective dose equivalent to the whole body of 45 and 10.8 mSv, respectively for 89Zr and 64Cu (17, 18). Of course, these preliminary results are only indicative because, the dosimetric data are also under the dependence of the vector pharmacokinetic profile, As consequences, the dosimetry for smaller mAb derivatives like nanobodies is lower (shorter in vivo stay, due to a fast renal clearance). For example, the dosimetry induced by 185 MBq 68Ga-2Rs15d nanobody is close to the dosimetry due to the classical 370 MBq 18F-FDG injection with, respectively, 4 and 7 mSv (19).
The chemical nature of the isotope presents a direct impact on the immunoprotein radiolabeling (20). Classically in radiopharmaceutical practices, radionuclides may belong to the radiohalogen or radiometal family, Radiohalogens like iodine-131 could be directly radiolabeled to the amino-acid chain on an aromatic residue (such histidine or more usually on a tyrosine) after gentle oxidation of iodide (21). This effective method presents some limits (mAb sensitive to oxidizing environment, low stability of radioactive mAb,…) and indirect radiolabeling could be envisaged. The indirect radiohalogen labeling is based on the use of prosthetic group intermediate (5, 22–25) like Bolton-Hunter reagent, organostanyl compound or iodonium salts (26–29).
Radiometals form very stable coordination complexes with a great variety of ligands, including linear diethylenetriaminepentaacetic acid (DTPA) derivatives, macrocyclic [1,4,7,10-tetraazacyclododecane-1,4,7,10-tetraacetic acid (DOTA) polyaminocarboxylic or desferrioxamine (DFO) derivatives] (30–32). These ligands are transformed in bifunctional chelating agent (BCA) capable of reacting with proteins to form a stable covalent bond with lysine residue, cysteine residue or synthetic bioorthogonal [click-chemistry approach (33)].
Finally, the production way of the isotope has consequences on the cost and in the availability of the radionuclide. The great majority of positron emitters are produced by cyclotron accelerator with a relatively high usual production cost. The half-life of the radionuclide presents a direct impact on the logistic because of decay during the transport. To circumvent this constraint a cyclotron network was built close to nuclear medicine departments for the short half-life isotopes (fluor-18; scandium-44; copper-64). These particular supply chain leads to a particular production schedule without daily availability of all isotopes. To solve this problem, some isotopes like Gallium-68 can be put in generator form (in this case, industrial supplier used germanium-68 with a longer half-life to produce in nuclear medicine department the desired gallium-68 by filiation).
The discovery of hybridoma technology by Köhler et al. (34) originally allowed the production of antigen-directed specific mAbs. This discovery paved the way for immuno-PET development but has appeared to be limited by the immunogenicity due to the murine origin of the amino-acid sequence (35). To circumvent this first hurdle, chimeric then humanized mAbs were designed by molecular biology and enabled less immunogenic approach with a better tolerance profile for the patients (36). The murine, chimeric and humanized mAbs are entire (intact) immunoglobulin G (IgG) with a long pharmacokinetic half-life and high weight (150 kDa) which reduces their capacity to diffuse inside the tumor mass.
To adapt these properties (pharmacokinetic and distribution), biochemists and immunochemists have developed some immunoconjugate derivatives exhibit reduced half-lives and improved tumoral penetration. The overall characteristics of these protein structures are summarized on Table 2.
The first improvement was the use of mAbs fragments like F(ab')2 and F(ab). These smaller protein fragments consist in the reduction/digestion of initial mAbs by enzymes (pepsin and papain). The F(ab')2 and F(ab) fragments are smaller (110 and 50 kDa, respectively) than the native mAbs but preserve the affinity and the specificity for the tumor antigen. F(ab')2 fragments of mAbs were radiolabeled with 64Cu in preclinical studies in breast cancer (37) or in lymphoma (38) animal models. Results showed a rapid and sustained uptake in tumor with promising and encouraging perspectives for the clinical evaluation of malignant pathologies while delivering a lower total body radiation dose compared to the entire mAbs. F(ab) fragments radiolabeled with 68Ga (39) or 64Cu (40) showed similar results with a shorter pharmacological half-life. In terms of biodistribution, F(ab) fragments present the characteristic of having a mass weight lower than the renal size cut-off (30–50 kDa) and consequently of being eliminated by the urinary tract (41). As consequence, F(ab) fragments cause high and persistent localization of the radioactivity in the kidney which could be reduced by a metabolizable linker between F(ab) fragment and isotope part of the construct (42).
Building on these successes, immunochemical engineers have turned to small synthetic proteins which contains only the antigen recognition domain of the initial mAb such minibodies, fusion protein-like single chain variable fragments (ScFv) or nanobodies. Minibodies consist of constant heavy chain (CH) and variable light (VL) and variable heavy (VH) domains, ScFv constructs are made of solely VL/VH domains and nanobodies has only the VH domains. 124I and 89Zr radiolabeled minibodies validated the feasibility proof-of-concept of immuno-PET in prostate cancer animal model (43). In breast cancer animal model, ScFv fragments radiolabeled with 68Ga (44) showed in vivo promising results. On their sides, nanobodies are subjects of intense preclinical research (45–47). These engineered antibody-based proteins demonstrate their capacity to be used in nuclear medicine field with β+ emitters for immuno-PET applications (48, 49). To date, the proof-of-concept was validated at a preclinical level and exploratory work in human is started (50).
More recently, synthetic proteins (three-helix scaffold) generated from a phage display library were produced with a high affinity against an antigenic structure. These proteins, named affibodies, have been prepared against different cancer targets for PET applications and were studied in vitro with promising results (51–53).
To optimize the tumor-to-background ratio, recent advances of nuclear medicine demonstrate the great interest of pretargeting approach (54). This technic involves a preliminary injection of a non-radioactive bispecific antibody followed by an injection of a radiolabeled bivalent hapten (peptide or small organic molecule). This system allows to bypass the slow clearance of bispecific mAbs. In this particular case, a bispecific mAb recognizes the tumor antigen with a high specificity by one of its two recognition domains. The second recognition domain is used to bind the radioactive hapten which is injected after the bispecific mAb within an optimal delay. The unbound hapten is rapidly cleared from the blood circulation via the renal system and allows, de facto, an increase of the immuno-PET image contrast. The hapten approach is not the only one feasible for pretargeting and bio-orthogonal reactions based on click-chemistry has recently been considered with successful results in preclinical studies (55, 56). The mAb (entire IgG or derivative) is in vitro prefunctionalized with a chemical clickable function. The intra-venous injection of this compound is followed by the injection of a radioactive compound with clickable complementary function and a specific covalent bond between these two components is in vivo formed. The relatively short pharmacokinetic half-life of the radioactive clickable moiety allows the use of shorter half-life isotopes and a renewed interest for fluorine-18 in immunoPET applications (57).
One of the first clinical proof of immuno-PET interest has been reported by Divgi et al. (58) with a chimeric mAb (cG250, girentuximab) directed toward the carbonic anhydrase IX (CAIX) cell-surface antigen particularly overexpressed in clear cell renal cell carcinoma (ccRCC). In this phase I clinical study, the mAb was 124I-radiolabeled and shows a very good sensitivity and specificity (respectively 94 and 100%) for ccRCC. Consolidated results obtained during the REDECT trial (Divgi JCO 2013) showed more robust results with sensitivity and specificity (respectively 86.2 and 85.9%). As consequences, immuno-PET can accurately and non-invasively assess the initial diagnostic of cancer without the inherent risk of biopsies. Despite this promising results, iodine-124 has physics drawbacks and zirconium-89 has been preferred in many subsequent clinical trials with girentuximab (59, 60). CAIX is also upregulated in various solid tumors and many clinical trials are currently underway for different tumor sites such as urothelial/bladder cancer (61) or triple negative breast cancer (OPALESCENCE Study Clinicaltrials.gov identifier NCT 04758780).
One of the first studied antigen for nuclear medicine purpose is the carcinoembryonic antigen (CEA). Different anti-CEA mAbs have been radiolabeled for therapeutic applications with 131I or for SPECT imaging with 111In. It is quite natural that it has been used in various immuno-PET studies. For example, AMG211 mAb was a bispecific antibody directed toward CEA and CD3 (62). AMG211 has been radiolabeled with 89Zr to evaluate the tumor uptake in relapsed/refractory gastrointestinal adenocarcinoma patients (Clinicaltrials.gov identifier NCT 02760199). M5A mAb is also an anti-CEA mAb and was radiolabeled with 124I for the detection of CEA positive colorectal cancer that has spread to the liver (Clinicaltrials.gov identifier NCT 03993327) or with 64Cu for the diagnosis of CEA positive rectal cancer (Clinicaltrials.gov identifier NCT 05245786) or in gastrointestinal, lung, medullary thyroid and breast cancers (Clinicaltrials.gov identifier NCT 02293954). CEA can also be targeted by a bispecific mAb for a pretargeting approach. TF2 is an engineered bispecific mAb of 157 kDa formed by the Dock-and-Lock® procedure from the anti-hapten Fab fragment recognizing the histamine-succinyl-glycine (HSG) motif and two humanized anti-CEA fragments. During the procedure (iTEP-CMT; Clinicaltrials.gov identifier NCT 02293954), non-radioactive TF2 mAb was injected in medullary thyroid carcinoma patients and 24 h later, 68Ga-di-HSG hapten was injected. The preliminary results demonstrated a promising sensitive and specific imaging method (63, 64) with a requirement in terms of optimization, both for the dosing (TF2 and hapten) and administration schedules (64). Similar and encouraging results were obtained in other CEA overexpressed solid tumors such colon (65) or breast cancer (66) with this pretargeted immuno-PET approach.
Finally, as another example to illustrate the large and recent evolution of immuno-PET capacities, cancer antigen 6 (CA6) can be targeted with a radiolabeled Fab (67). CA6 is a neuraminidase-sensitive and periodic acid-sensitive sialic acid glycoconjugate often overexpressed in various carcinoma such pancreas, ovary, breast and bladder (68). 64Cu-anti-CA6-Fab was clinically used in ovarian and breast cancer (Clinicaltrials.gov identifier NCT 02708511).
One of the most widely used mAbs in clinical practice is trastuzumab. Trastuzumab is directed against Human Epidermal growth factor Receptor 2 (HER2) which is particularly overexpressed in breast cancer. In 2010, Dijkers et al. (69) reported a feasibility study of 89Zr-trastuzumab in HER2-positive metastatic breast cancer and has shown the immuno-PET capacity to detect most of the known lesions and some that had remained unnoticed with other diagnostic modalities (CT, MRI or bone scans). Based on this first encouraging results, many clinical trials (70, 71) have been conducted to evaluate the tumor uptake of 89Zr-trastuzumab in breast tumor and in their metastatic lesions. The results have shown medium sensitivity of 75.8% (57.7–88.9%) and specificity of 61.5% (31.6–86.1%). These scattering of preliminary results seems to be explained by the high liver uptake which is strongly dependent of the non-radiaocative mAb injected. Indeed, when the authors remove the liver metastases from their interpretation, the SUVmax appears to be significantly higher in HER2 positive compared to HER2 negative patients. In this study, immuno-PET approach demonstrated its ability to identify the intrapatient heterogeneity of 89Zr-trastuzumab uptake in 20% of patient with multiple lesions. This intrapatient heterogeneity could be explained by sampling error during biopsy, heterogeneous intratumoral distribution of HER2 antigen or expression change of HER2 status since the last biopsy (72). Nevertheless, 89Zr-trastuzumab has the potential to characterize the whole body HER2 status of all the tumor and metastatic sites thus obviating repeated tissue biopsies to assess the intrapatient heterogeneity. Another advantage of the immuno-PET in these clinical circumstances is that it allows a quantitative evaluation of the target expression to optimize the future therapeutic dose and allows a better evaluation of the in-vivo penetration (ability to cross the physiologic barrier) of mAbs in the tumor mass comparatively to the ex-vivo glass-slide immunohistochemistry (IHC) provided by cytology (73). A possible future for this dose finding may be clinically translated to predict and to monitor the HER2-targeted therapeutics treatments (74). The tumor uptake of 89Zr-trastuzumab is related to the target concentration but there is still a pitfall due to the small range for distinguishing IHC classes 1 to 3 with relatively constant SUV. Currently, the whole-body quantitative imaging objective with HER2 immuno-PET has not been reached and requires a normalization of the non-radioactive trastuzumab mass dose injected to minimize the accumulation in health tissue and to maximize the contrast with cancer lesions (69, 73). Nevertheless, this quantitative approach of the in vivo status of HER2 in patients with metastatic breast cancer is proving to be an interesting tool in predictive of respond and benefit for women (ZEPHIR clinical trial) which receiving antibody drug conjugate such trastuzumab emtansine (T-DM1) as second line treatment of HER2+ metastatic breast cancer (75, 76). The ZEPHIR clinical trial categorized patients into 4 subgroups in function of the tracer uptake (18F-FDG and 89Zr-trastuzumab) patterns and allows a very good prognostic in response evaluation. To optimize the radiation safety and patient radiation dose, copper-64 was used by Mortimer et al. (77–79). 64Cu-trastuzumab using in HER2 positive breast cancer patients showed a rapid tumor and metastasis uptake of the radiolabeled mAb in a similar way than 89Zr (80). A promising clinical trial (Clinicaltrials.gov identifier NCT 05376878) is currently in progress to determine the ability of 64Cu-trastuzumab immuno-PET to detect positive brain metastases of breast cancer. HER2 antigen is also overexpressed in ~20% of esophagogastric adenocarcinoma and 89Zr-trastuzumab showed promising results (safe and high-quality images) in patients with HER2 positive tumors (81). Same promising results were obtained in gastric cancer with 64Cu-trastuzumab (Clinicaltrials.gov identifier NCT 01939275). In regards of the nanobodies applications, a phase I study confirmed the capacity of 68Ga-HER2-nanobody to provide safe and informative uptake in breast carcinoma (82).
Despite the very large number of clinical trials using small molecules, the Prostate-Specific Membrane Antigen (PSMA) can also be targeted by mAbs. PSMA is particularly overexpressed in prostate adenocarcinoma and J591 mAb was firstly radiolabeled by 124I then by 89Zr (83). The first-in-human use of 89Zr-J591 (84) showed a correlation between the uptake of 89Zr-J591 and the tumor aggressiveness. These first results were confirmed by a phase I/II clinical trial and demonstrated a superior targeting of bone metastases lesions compared to conventional imaging modalities (85). In prostate cancer, 64Cu radiolabeled anti-PSMA engineered humanized mAb (HuX592r) is currently under clinical trial (CUPID Study, Clinicaltrials.gov identifier NCT 04726033) to determine the safety, pharmacokinetic, whole body distribution and radiation dosimetry of this possible new immuno-PET modality.
Encouraging results were observed with the epidermal growth factor receptor (EGFR) where 89Zr-cetuximab (86) has provided additional information on advanced head and neck cancer (ARTFORCE study, Clinicaltrials.gov identifier NCT 01504815) for an enlightened choice of the most effective treatment between cisplatin or cetuximab (87, 88).
Blood cells and hematopoietic stem cells located in bone marrow are well-known to be radiosensitive. As a consequence, hematological diseases such as B-cell non-Hodgkin lymphoma or multiple myeloma are highly studied malignancies in nuclear medicine. Twenty years ago, overexpressed B-lymphocyte antigen CD20 provided an interesting target for β− radioimmunotherapy (RIT) applications with 90Y or 131I radiolabeled mAbs. At the same period, 86Y has gained interest as an interest surrogate for immuno-PET complement of 90Y-ibritumomab-tiuxetan RIT (89). Due to the 86Y physical drawbacks, 89Zr-ibritumomab-tiuxetan was preferred for immuno-PET clinical trial. Rizvi et al. (90) conducted a prospective clinical study to analyze the biodistribution and the radiation dosimetry of 89Zr-ibritumomab-tiuxetan prior and after 90Y-ibritumomab-tiuxetan RIT. This study has confirmed the potential value of immuno-PET to predict and to optimize RIT as individualized treatment. CD20 antigen is overexpressed in diffuse large B-cell lymphoma, commercial rituximab mAb has an indication on this pathology and can be functionalized with desferrioxamine derivatives for 89Zr radiolabeling (91). 89Zr-rituximab clinical trial showed a strong correlation between the tumor uptake and CD20 expression (92) with a promising expectation to predict the benefit of “cold” rituximab therapy and to guide individualized treatment. To date, a clinical trial with 64Cu-rituximab (Clinicaltrials.gov identifier NCT 01598558) was scheduled but withdrawn without explications in non-Hodgkin lymphoma and could, in a near future, provide new information for immuno-PET development. Daratumumab is a mAb that targets CD38, an antigen overexpressed in nearly all myeloma cells. 89Zr-daratumumab was synthesized and showed in a first-in-human study (Clinicaltrials.gov identifier NCT 03665155) a highly sensitive detection of multiple myeloma. These preliminary results allowed a good bone localization before therapy, the quantification of the disease burden before therapy, the best responders for “cold” daratumumab therapy and a detection of minimal residual disease after therapy (93). Daratumumab was also 64Cu radiolabeled by Krishnan et al. and has allowed whole body images of the multiple myeloma expansion. This 64Cu-daratumumab clinical study (Clinicaltrials.gov identifier NCT 03311828) showed a better concordance (94) between biopsy results and 64Cu-daratumumab than classical 18F-FDG (uptake due to inflammatory region). A future phase II clinical trial is scheduled to determine the sensitivity and the specificity of 64Cu-daratumumab in multiple myeloma.
Recent advances in oncology have revealed the greatest interest of the tumor microenvironment in the disease proliferation process. Tumor microenvironment is an important site of immunologic response and tumor-infiltrating T-cell can be targeted by an anti-CD8 minibody radiolabeled with 89Zr (89Zr-DfIABM2C). Phase I clinical trial showed promising results in 15 patients to predict early response to immunotherapy (95). The tumor response is under the regulation of immune check point and recent advances in this immunologic field showed the great importance of PD1/PDL1 system (96). Research on these immune check points has identified the program cell death protein (PD1) and the program cell death ligand (PDL1) to have a key role in this process. Overexpression of PD1/PDL1 axis is associated with a poorly patient prognostic in a large range of cancers (97, 98). The potential interest of non-invasive PET imaging of tumor PD1/PDL1 expression is therefore of prime importance for the patient care (99). One of the most used mAb specifically for PD1 is pembrolizumab which could be radiolabeled with 89Zr. 89Zr-penbrolizumab remained stable in blood circulation with a classical accumulation in liver and spleen tissues (100). Some clinical trials (Clinicaltrials.gov identifiers NCT 02760225; NCT 03065764) were done (or in progress) with 89Zr-penbrolizumab and has shown uptake in tumor lesions correlated with treatment response (101). This study was performed in a small patient cohort (14 patients enrolled) and show promised results but need to be confirmed by a larger clinical trial. Similar results were obtained with 89Zr-atezolizumab (directed against PDL1) in lobular breast cancer during the ImaGelato clinical study (Clinicaltrials.gov identifier NCT 04222426) (102) or with 89Zr-durvalumab in non-small cell lung cancer (103).
To provide increase oxygen and nutrients supply for the growing tumor, tumor cells induct neoangiogenesis by vascular endothelial growth factor (VEGF) secretion. VEGF receptors located at the surface of the endothelial cells can be targeted by mAbs such bevacizumab. 89Zr-labeled bevacizumab was prepared and administered in patients with non-small cell lung cancer and showed a correlation between tumor-uptake and progression-free survival and overall survival after treatment (104).
Today, the immuno-PET field is rapidly progressing and allows to provide essential information for the care management of each patient. To meet the need of personalized medicine era where each patient is unique, immuno-PET provides repeatable, non-invasive whole-body information of biomarkers mapping. Many preclinical developments and clinical proof-of-concepts have been done or are ongoing. The recent clinical developments of immuno-PET have confirmed the potential of mAbs (and their derivatives) as companion diagnostic to determine, whether or not, a patient will respond to a targeted therapy. Nevertheless, the majority of proof-of-concept clinical studies have been performed on small patient samples and require larger studies to confirm their potential as predictive imaging biomarkers. Currently, the main limitation for these clinical studies with great patient numbers are often limited by the costs and the availability limitation of isotopes.
The original phenotypic information provided by immuno-PET currently allows in vivo access of several pieces of information on the tumor aggressivity associated to a patient outcome prognostic. The power of this molecular imaging modality seems to be able to show very informative data on the intra-tumor and intra-patient variability on molecular biomarkers expression and consecutively in phenotypic heterogeneity of the entire disease burden. Moreover, in comparison with “gold-standard” biopsies, immuno-PET allows to acquire in-vivo knowledge of the intra-tumoral penetration/biodistribution of the mAb without inherent-risk of tumor seeding during needle biopsy (105).
Beyond the image information, immuno-PET is attractive to study the in-vivo behavior of antibody-based therapies and for understanding their therapeutic efficacities. Within the scope of theranostic approach, the use of β+/β− pair (respectively for diagnostic then therapy) radiolabeling the same mAb is very promising because the same distribution/pharmacokinetic is expected. Immuno-PET allows in theranostic the determination of the patient dosimetry to optimize the cumulated activity and to predict the therapy response by a quantitative measurement of the tumor-antibody uptake. Molecular imaging by immuno-PET with mAbs or their derivatives will play a pivotal role in the close oncologic future to tailor a customized therapy for each patient.
CB, CR, FK-B, and CB-M are nuclear medicine physicians. They have worked for many years on the development of immuno-PET approach in oncology. AL is an endocrinologist with an expertise in immuno-PET approach for neuroendocrine tumors. MC is pharmacist, head of the nuclear oncology research team, with a broad expertise in immunology. MB is radiopharmacist with a long term wide experience of radiopharmaceutical development, mainly for immuno-PET applications. All authors contributed to the article and approved the submitted version.
The authors thank the French National Agency for Research called Investissements d'Avenir via grants Labex IRON n°ANR-11-LABX-0018-01 for their support.
The authors declare that the research was conducted in the absence of any commercial or financial relationships that could be construed as a potential conflict of interest.
All claims expressed in this article are solely those of the authors and do not necessarily represent those of their affiliated organizations, or those of the publisher, the editors and the reviewers. Any product that may be evaluated in this article, or claim that may be made by its manufacturer, is not guaranteed or endorsed by the publisher.
1. Strebhardt K, Ullrich A, Strebhardt K, Ullrich A. Paul Ehrlich's magic bullet concept: 100 years of progress. Nat Rev Cancer. (2008) 8:473-80. doi: 10.1038/nrc2394
2. Köhler G, Milstein C. Continuous cultures of fused cells secreting antibody of predefined specificity. Nature. (1975) 256:495-7. doi: 10.1038/256495a0
3. Goldenberg DM, DeLand F, Kim E, Bennett S, Primus FJ, van Nagell JR, et al. Use of radiolabeled antibodies to carcinoembryonic antigen for the detection and localization of diverse cancers by external photoscanning. N Engl J Med. (1978) 298:1384-6. doi: 10.1056/NEJM197806222982503
4. Herlyn M, Steplewski Z, Herlyn D, Koprowski H. Colorectal carcinoma-specific antigen: detection by means of monoclonal antibodies. Proc Natl Acad Sci USA. (1979) 76:1438-42. doi: 10.1073/pnas.76.3.1438
5. DeNardo SJ, DeNardo GL, O'Grady LF, Macey DJ, Mills SL, Epstein AL, et al. Treatment of a patient with B cell lymphoma by I-131 LYM-1 monoclonal antibodies. Int J Biol Markers. (1987) 2:49-53. doi: 10.1177/172460088700200107
6. Jayson GC, Zweit J, Jackson A, Mulatero C, Julyan P, Ranson M, et al. Molecular imaging and biological evaluation of HuMV833 anti-VEGF antibody: implications for trial design of antiangiogenic antibodies. J Natl Cancer Inst. (2002) 94:1484-93. doi: 10.1093/jnci/94.19.1484
7. Lövqvist A, Humm JL, Sheikh A, Finn RD, Koziorowski J, Ruan S, et al. PET imaging of 86Y-labeled anti-Lewis Y monoclonal antibodies in a nude mouse model: comparison between 86Y and 111In radiolabels. J Nucl Med. (2001) 42:1281-7.
8. Verel I, Visser GWM, Boellaard R, Stigter-van Walsum M, Snow GB, van Dongen GAMS. 89Zr immuno-PET: comprehensive procedures for the production of 89Zr-labeled monoclonal antibodies. J Nucl Med. (2003) 44:1271-81.
9. Lewellen TK. Recent developments in PET detector technology. Phys Med Biol. (2008) 53:R287–317. doi: 10.1088/0031-9155/53/17/R01
10. Stute S, Benoit D, Martineau A, Rehfeld NS, Buvat I. A method for accurate modelling of the crystal response function at a crystal sub-level applied to PET reconstruction. Phys Med Biol. (2011) 56:793-809. doi: 10.1088/0031-9155/56/3/016
11. Moses WW. Recent advances and future advances in time-of-flight PET. Nucl Instrum Methods Phys Res A. (2007) 580:919-24. doi: 10.1016/j.nima.2007.06.038
12. Wei W, Rosenkrans ZT, Liu J, Huang G, Luo QY, Cai W. ImmunoPET: concept, design, and applications. Chem Rev. (2020) 120:3787-851. doi: 10.1021/acs.chemrev.9b00738
13. Manafi-Farid R, Ataeinia B, Ranjbar S, Jamshidi Araghi Z, Moradi MM, Pirich C, et al. ImmunoPET: antibody-based PET imaging in solid tumors. Front Med. (2022) 9:916693. doi: 10.3389/fmed.2022.916693
14. Kraeber-Bodéré F, Rousseau C, Bodet-Milin C, Mathieu C, Guérard F, Frampas E, et al. Tumor immunotargeting using innovative radionuclides. Int J Mol Sci. (2015) 16:3932-54. doi: 10.3390/ijms16023932
15. Boswell CA, Brechbiel MW. Development of radioimmunotherapeutic and diagnostic antibodies: an inside-out view. Nucl Med Biol. (2007) 34:757-78. doi: 10.1016/j.nucmedbio.2007.04.001
16. Zhou Y, Baidoo KE, Brechbiel MW. Mapping biological behaviors by application of longer-lived positron emitting radionuclides. Adv Drug Deliv Rev. (2013) 65:1098-111. doi: 10.1016/j.addr.2012.10.012
17. Laforest R, Lapi SE, Oyama R, Bose R, Tabchy A, Marquez-Nostra BV, et al. [89Zr]Trastuzumab: evaluation of radiation dosimetry, safety, and optimal imaging parameters in women with HER2-positive breast cancer. Mol Imaging Biol. (2016) 18:952-9. doi: 10.1007/s11307-016-0951-z
18. Lee I, Lim I, Byun BH, Kim BI, Choi CW, Woo SK, et al. A preliminary clinical trial to evaluate 64Cu-NOTA-Trastuzumab as a positron emission tomography imaging agent in patients with breast cancer. EJNMMI Res. (2021) 11:8. doi: 10.1186/s13550-021-00746-1
19. Xavier C, Vaneycken I, D'huyvetter M, Heemskerk J, Keyaerts M, Vincke C, et al. Synthesis, preclinical validation, dosimetry, and toxicity of 68Ga-NOTA-anti-HER2 nanobodies for iPET imaging of HER2 receptor expression in cancer. J Nucl Med. (2013) 54:776-84. doi: 10.2967/jnumed.112.111021
20. Deri MA, Zeglis BM, Francesconi LC, Lewis JS. PET imaging with 89Zr: from radiochemistry to the clinic. Nucl Med Biol. (2013) 40:3-14. doi: 10.1016/j.nucmedbio.2012.08.004
21. Eckelman WC, Paik CH, Reba RC. Radiolabeling of antibodies. Cancer Res. (1980) 40(Pt. 2):3036-42.
22. Wilbur DS. Radiohalogenation of proteins: an overview of radionuclides, labeling methods, and reagents for conjugate labeling. Bioconjug Chem. (1992) 3:433-70. doi: 10.1021/bc00018a001
23. Gupta S, Batra S, Jain M. Antibody labeling with radioiodine and radiometals. Methods Mol Biol. (2014) 1141:147-57. doi: 10.1007/978-1-4939-0363-4_9
24. Boswell CA, Marik J, Elowson MJ, Reyes NA, Ulufatu S, Bumbaca D, et al. Enhanced tumor retention of a radiohalogen label for site-specific modification of antibodies. J Med Chem. (2013) 56:9418-26. doi: 10.1021/jm401365h
25. Mamat C, Gott M, Steinbach J. Recent progress using the Staudinger ligation for radiolabeling applications. J Label Compd Radiopharm. (2018) 61:165-78. doi: 10.1002/jlcr.3562
26. Bolton AE, Hunter WM. The labelling of proteins to high specific radioactivities by conjugation to a 125I-containing acylating agent. Biochem J. (1973) 133:529-39. doi: 10.1042/bj1330529
27. Yordanov AT, Garmestani K, Zhang M, Zhang Z, Yao Z, Phillips KE, et al. Preparation and in vivo evaluation of linkers for 211At labeling of humanized anti-Tac. Nucl Med Biol. (2001) 28:845-56. doi: 10.1016/S0969-8051(01)00257-8
28. Bourgeois M, Guerard F, Alliot C, Mougin-Degraef M, Rajérison H, Remaud-Le Saëc P, et al. Feasibility of the radioastatination of a monoclonal antibody with astatine-211 purified by wet extraction. J Label Compd Radiopharm. (2008) 51:379-83. doi: 10.1002/jlcr.1543
29. Guérard F, Navarro L, Lee YS, Roumesy A, Alliot C, Chérel M, et al. Bifunctional aryliodonium salts for highly efficient radioiodination and astatination of antibodies. Bioorg Med Chem. (2017) 25:5975-80. doi: 10.1016/j.bmc.2017.09.022
30. Price EW, Orvig C. Matching chelators to radiometals for radiopharmaceuticals. Chem Soc Rev. (2014) 43:260-90. doi: 10.1039/C3CS60304K
31. Vugts DJ, van Dongen GAMS. (89)Zr-labeled compounds for PET imaging guided personalized therapy. Drug Discov Today Technol. (2011) 8:e53–61. doi: 10.1016/j.ddtec.2011.12.004
32. Liu S. Bifunctional coupling agents for radiolabeling of biomolecules and target-specific delivery of metallic radionuclides. Adv Drug Deliv Rev. (2008) 60:1347-70. doi: 10.1016/j.addr.2008.04.006
33. Fujiki K, Yano S, Ito T, Kumagai Y, Murakami Y, Kamigaito O, et al. A one-pot three-component double-click method for synthesis of [67Cu]-labeled biomolecular radiotherapeutics. Sci Rep. (2017) 7:1912. doi: 10.1038/s41598-017-02123-2
34. Köhler G, Hengartner H, Shulman MJ. Immunoglobulin production by lymphocyte hybridomas. Eur J Immunol. (1978) 8:82-8. doi: 10.1002/eji.1830080203
35. Frödin JE, Lefvert AK, Mellstedt H. The clinical significance of HAMA in patients treated with mouse monoclonal antibodies. Cell Biophys. (1992) 21:153-65. doi: 10.1007/BF02789485
36. Waldmann H. Human monoclonal antibodies: the benefits of humanization. Methods Mol Biol. (2019) 1904:1-10. doi: 10.1007/978-1-4939-8958-4_1
37. Lam K, Chan C, Reilly RM. Development and preclinical studies of 64Cu-NOTA-pertuzumab F(ab')2 for imaging changes in tumor HER2 expression associated with response to trastuzumab by PET/CT. mAbs. (2017) 9:154-64. doi: 10.1080/19420862.2016.1255389
38. Kang L, Li C, Rosenkrans ZT, Engle JW, Wang R, Jiang D, et al. Noninvasive evaluation of CD20 expression using 64Cu-labeled F(ab')2 fragments of obinutuzumab in lymphoma. J Nucl Med. (2021) 62:372-8. doi: 10.2967/jnumed.120.246595
39. The MICAD Research Team. 68Ga-Trastuzumab F(ab') fragment. In: Molecular Imaging and Contrast Agent Database (MICAD). Bethesda, MD: National Center for Biotechnology Information (US) (2004). Available online at: http://www.ncbi.nlm.nih.gov/books/NBK23296/ (accessed August 22, 2022).
40. Chan C, Scollard DA, McLarty K, Smith S, Reilly RM. A comparison of 111In- or 64Cu-DOTA-trastuzumab Fab fragments for imaging subcutaneous HER2-positive tumor xenografts in athymic mice using microSPECT/CT or microPET/CT. EJNMMI Res. (2011) 1:15. doi: 10.1186/2191-219X-1-15
41. Roksnoer LCW, Heijnen BFJ, Nakano D, Peti-Peterdi J, Walsh SB, Garrelds IM, et al. On the origin of urinary renin: a translational approach. Hypertens. (2016) 67:927-33. doi: 10.1161/HYPERTENSIONAHA.115.07012
42. Suzuki H, Kise S, Kaizuka Y, Watanabe R, Sugawa T, Furukawa T, et al. Copper-64-labeled antibody fragments for immuno-pet/radioimmunotherapy with low renal radioactivity levels and amplified tumor-kidney ratios. ACS Omega. (2021) 6:21556-62. doi: 10.1021/acsomega.1c02516
43. Knowles SM, Zettlitz KA, Tavaré R, Rochefort MM, Salazar FB, Stout DB, et al. Quantitative immunoPET of prostate cancer xenografts with 89Zr- and 124I-labeled anti-PSCA A11 minibody. J Nucl Med. (2014) 55:452-9. doi: 10.2967/jnumed.113.120873
44. Ueda M, Hisada H, Temma T, Shimizu Y, Kimura H, Ono M, et al. Gallium-68-labeled anti-HER2 single-chain Fv fragment: development and in vivo monitoring of HER2 expression. Mol Imaging Biol. (2015) 17:102-10. doi: 10.1007/s11307-014-0769-5
45. Bala G, Crauwels M, Blykers A, Remory I, Marschall ALJ, Dübel S, et al. Radiometal-labeled anti-VCAM-1 nanobodies as molecular tracers for atherosclerosis - impact of radiochemistry on pharmacokinetics. Biol Chem. (2019) 400:323-32. doi: 10.1515/hsz-2018-0330
46. Gao H, Wu Y, Shi J, Zhang X, Liu T, Hu B, et al. Nuclear imaging-guided PD-L1 blockade therapy increases effectiveness of cancer immunotherapy. J Immunother Cancer. (2020) 8:e001156. doi: 10.1136/jitc-2020-001156
47. Chigoho DM, Lecocq Q, Awad RM, Breckpot K, Devoogdt N, Keyaerts M, et al. Site-specific radiolabeling of a human Pd-l1 nanobody via maleimide-cysteine chemistry. Pharmaceuticals. (2021) 14:550. doi: 10.3390/ph14060550
48. Rashidian M, Ploegh H. Nanobodies as non-invasive imaging tools. Immuno-Oncol Technol. (2020) 7:2-14. doi: 10.1016/j.iotech.2020.07.001
49. Berland L, Kim L, Abousaway O, Mines A, Mishra S, Clark L, et al. Nanobodies for medical imaging: about ready for prime time? Biomolecules. (2021) 11:637. doi: 10.3390/biom11050637
50. Diebolder P, Mpoy C, Scott J, Huynh TT, Fields R, Spitzer D, et al. Preclinical evaluation of an engineered single-chain fragment variable-fragment crystallizable targeting human CD44. J Nucl Med. (2021) 62:137-43. doi: 10.2967/jnumed.120.249557
51. Altunay B, Morgenroth A, Beheshti M, Vogg A, Wong NCL, Ting HH, et al. HER2-directed antibodies, affibodies and nanobodies as drug-delivery vehicles in breast cancer with a specific focus on radioimmunotherapy and radioimmunoimaging. Eur J Nucl Med Mol Imaging. (2021) 48:1371-89. doi: 10.1007/s00259-020-05094-1
52. Zhou N, Liu C, Guo X, Xu Y, Gong J, Qi C, et al. Impact of 68Ga-NOTA-MAL-MZHER2 PET imaging in advanced gastric cancer patients and therapeutic response monitoring. Eur J Nucl Med Mol Imaging. (2021) 48:161-75. doi: 10.1007/s00259-020-04898-5
53. Oroujeni M, Rinne SS, Vorobyeva A, Loftenius A, Feldwisch J, Jonasson P, et al. Preclinical evaluation of 99mTc-ZHER2:41071, a second-generation affibody-based HER2-visualizing imaging probe with a low renal uptake. Int J Mol Sci. (2021) 22:2770. doi: 10.3390/ijms22052770
54. Cheal SM, Chung SK, Vaughn BA, Cheung NKV, Larson SM. Pretargeting: a path forward for radioimmunotherapy. J Nucl Med. (2022) 63:1302-15. doi: 10.2967/jnumed.121.262186
55. Keinänen O, Fung K, Brennan JM, Zia N, Harris M, van Dam E, et al. Harnessing 64Cu/67Cu for a theranostic approach to pretargeted radioimmunotherapy. Proc Natl Acad Sci USA. (2020) 117:28316-27. doi: 10.1073/pnas.2009960117
56. Sarrett SM, Keinänen O, Dayts EJ, Dewaele-Le Roi G, Rodriguez C, Carnazza KE, et al. Inverse electron demand Diels-Alder click chemistry for pretargeted PET imaging and radioimmunotherapy. Nat Protoc. (2021) 16:3348-81. doi: 10.1038/s41596-021-00540-2
57. Meyer JP, Houghton JL, Kozlowski P, Abdel-Atti D, Reiner T, Pillarsetty NVK, et al. 18F-Based pretargeted PET imaging based on bioorthogonal Diels-Alder click chemistry. Bioconjug Chem. (2016) 27:298-301. doi: 10.1021/acs.bioconjchem.5b00504
58. Divgi CR, Pandit-Taskar N, Jungbluth AA, Reuter VE, Gönen M, Ruan S, et al. Preoperative characterisation of clear-cell renal carcinoma using iodine-124-labelled antibody chimeric G250 (124I-cG250) and PET in patients with renal masses: a phase I trial. Lancet Oncol. (2007) 8:304-10. doi: 10.1016/S1470-2045(07)70044-X
59. Verhoeff SR, van Es SC, Boon E, van Helden E, Angus L, Elias SG, et al. Lesion detection by [89Zr]Zr-DFO-girentuximab and [18F]FDG-PET/CT in patients with newly diagnosed metastatic renal cell carcinoma. Eur J Nucl Med Mol Imaging. (2019) 46:1931-9. doi: 10.1007/s00259-019-04358-9
60. Merkx RIJ, Lobeek D, Konijnenberg M, Jiménez-Franco LD, Kluge A, Oosterwijk E, et al. Phase I study to assess safety, biodistribution and radiation dosimetry for 89Zr-girentuximab in patients with renal cell carcinoma. Eur J Nucl Med Mol Imaging. (2021) 48:3277-85. doi: 10.1007/s00259-021-05271-w
61. Al-Zubaidi M, Viswambaram P, McCombie S, Liow E, Lenzo N, Ferguson T, et al. 89Zirconium-labelled girentuximab (89Zr-TLX250) PET in Urothelial Cancer Patients (ZiPUP): protocol for a phase I trial of a novel staging modality for urothelial carcinoma. BMJ Open. (2022) 12:e060478. doi: 10.1136/bmjopen-2021-060478
62. Waaijer SJH, Warnders FJ, Stienen S, Friedrich M, Sternjak A, Cheung HK, et al. Molecular imaging of radiolabeled bispecific T-cell engager 89Zr-AMG211 targeting CEA-positive tumors. Clin Cancer Res. (2018) 24:4988-96. doi: 10.1158/1078-0432.CCR-18-0786
63. Bodet-Milin C, Faivre-Chauvet A, Carlier T, Rauscher A, Bourgeois M, Cerato E, et al. Immuno-PET using anticarcinoembryonic antigen bispecific antibody and 68Ga-labeled peptide in metastatic medullary thyroid carcinoma: clinical optimization of the pretargeting parameters in a first-in-human trial. J Nucl Med. (2016) 57:1505-11. doi: 10.2967/jnumed.116.172221
64. Bodet-Milin C, Faivre-Chauvet A, Carlier T, Ansquer C, Rauscher A, Frampas E, et al. Anti-CEA pretargeted immuno-PET shows higher sensitivity than DOPA PET/CT in detecting relapsing metastatic medullary thyroid carcinoma: post hoc analysis of the iPET-MTC study. J Nucl Med. (2021) 62:1221-7. doi: 10.2967/jnumed.120.252791
65. Touchefeu Y, Bailly C, Frampas E, Eugène T, Rousseau C, Bourgeois M, et al. Promising clinical performance of pretargeted immuno-PET with anti-CEA bispecific antibody and gallium-68-labelled IMP-288 peptide for imaging colorectal cancer metastases: a pilot study. Eur J Nucl Med Mol Imaging. (2021) 48:874-82. doi: 10.1007/s00259-020-04989-3
66. Rousseau C, Goldenberg DM, Colombié M, Sébille JC, Meingan P, Ferrer L, et al. Initial clinical results of a novel immuno-Pet theranostic probe in human epidermal growth factor receptor 2-negative breast cancer. J Nucl Med. (2020) 61:1205-11. doi: 10.2967/jnumed.119.236000
67. Ilovich O, Natarajan A, Hori S, Sathirachinda A, Kimura R, Srinivasan A, et al. Development and validation of an immuno-pet tracer as a companion diagnostic agent for antibody-drug conjugate therapy to target the CA6 Epitope. Radiology. (2015) 276:191-8. doi: 10.1148/radiol.15140058
68. Smith NL, Halliday BE, Finley JL, Wennerberg AEK. The spectrum of immunohistochemical reactivity of monoclonal antibody DS6 in nongynecologic neoplasms. Appl Immunohistochem Mol Morphol AIMM. (2002) 10:152-8. doi: 10.1097/00129039-200206000-00010
69. Dijkers EC, Oude Munnink TH, Kosterink JG, Brouwers AH, Jager PL, de Jong JR, et al. Biodistribution of 89Zr-trastuzumab and PET imaging of HER2-positive lesions in patients with metastatic breast cancer. Clin Pharmacol Ther. (2010) 87:586-92. doi: 10.1038/clpt.2010.12
70. Bensch F, Brouwers AH, Lub-de Hooge MN, de Jong JR, van der Vegt B, Sleijfer S, et al. 89Zr-trastuzumab PET supports clinical decision making in breast cancer patients, when HER2 status cannot be determined by standard work up. Eur J Nucl Med Mol Imaging. (2018) 45:2300-6. doi: 10.1007/s00259-018-4099-8
71. Ulaner GA, Hyman DM, Lyashchenko SK, Lewis JS, Carrasquillo JA. 89Zr-Trastuzumab PET/CT for detection of human epidermal growth factor receptor 2-positive metastases in patients with human epidermal growth factor receptor 2-negative primary breast cancer. Clin Nucl Med. (2017) 42:912-7. doi: 10.1097/RLU.0000000000001820
72. Dehdashti F, Wu N, Bose R, Naughton MJ, Ma CX, Marquez-Nostra BV, et al. Evaluation of [89Zr]trastuzumab-PET/CT in differentiating HER2-positive from HER2-negative breast cancer. Breast Cancer Res Treat. (2018) 169:523-30. doi: 10.1007/s10549-018-4696-z
73. Huisman MC, Menke-van der Houven van Oordt CW, Zijlstra JM, Hoekstra OS, Boellaard R, van Dongen GAMS, et al. Potential and pitfalls of 89Zr-immuno-PET to assess target status: 89Zr-trastuzumab as an example. EJNMMI Res. (2021) 11:74. doi: 10.1186/s13550-021-00813-7
74. Kang M, Shin JI, Han S, Kim JY, Park J, Kim KI, et al. Therapeutic response monitoring with 89Zr-DFO-pertuzumab in HER2-positive and trastuzumab-resistant breast cancer models. Pharmaceutics. (2022) 14:1338. doi: 10.3390/pharmaceutics14071338
75. Gebhart G, Lamberts LE, Wimana Z, Garcia C, Emonts P, Ameye L, et al. Molecular imaging as a tool to investigate heterogeneity of advanced HER2-positive breast cancer and to predict patient outcome under trastuzumab emtansine (T-DM1): the ZEPHIR trial. Ann Oncol. (2016) 27:619-24. doi: 10.1093/annonc/mdv577
76. Gebhart G, Lamberts LE, Garcia C, Ameye L, Stroobants S, Huizing M, et al. PET/CT with 89Zr-trastuzumab and 18F-FDG to individualize treatment with trastuzumab emtansine (T-DM1) in metastatic HER2-positive breast cancer (mBC). J Clin Oncol. (2014) 32:11001. doi: 10.1200/jco.2014.32.15_suppl.11001
77. Mortimer JE, Bading JR, Colcher DM, Conti PS, Frankel PH, Carroll MI, et al. Functional imaging of human epidermal growth factor receptor 2-positive metastatic breast cancer using (64)Cu-DOTA-trastuzumab PET. J Nucl Med. (2014) 55:23-9. doi: 10.2967/jnumed.113.122630
78. Mortimer JE, Bading JR, Park JM, Frankel PH, Carroll MI, Tran TT, et al. Tumor uptake of 64Cu-DOTA-trastuzumab in patients with metastatic breast cancer. J Nucl Med. (2018) 59:38-43. doi: 10.2967/jnumed.117.193888
79. Mortimer JE, Bading JR, Frankel PH, Carroll MI, Yuan Y, Park JM, et al. Use of 64Cu-DOTA-trastuzumab PET to predict response and outcome of patients receiving trastuzumab emtansine for metastatic breast cancer: a pilot study. J Nucl Med. (2022) 63:1145-8. doi: 10.2967/jnumed.121.262940
80. Carrasquillo JA, Morris PG, Humm JL, Smith-Jones PM, Beylergil V, Akhurst T, et al. Copper-64 trastuzumab PET imaging: a reproducibility study. Q J Nucl Med Mol Imaging. (2019) 63:191-8. doi: 10.23736/S1824-4785.16.02867-3
81. O'Donoghue JA, Lewis JS, Pandit-Taskar N, Fleming SE, Schöder H, Larson SM, et al. Pharmacokinetics, biodistribution, and radiation dosimetry for 89Zr-trastuzumab in patients with esophagogastric cancer. J Nucl Med. (2018) 59:161-6. doi: 10.2967/jnumed.117.194555
82. Keyaerts M, Xavier C, Heemskerk J, Devoogdt N, Everaert H, Ackaert C, et al. Phase I study of 68Ga-HER2-nanobody for PET/CT assessment of HER2 expression in breast carcinoma. J Nucl Med. (2016) 57:27-33. doi: 10.2967/jnumed.115.162024
83. Fung EK, Cheal SM, Fareedy SB, Punzalan B, Beylergil V, Amir J, et al. Targeting of radiolabeled J591 antibody to PSMA-expressing tumors: optimization of imaging and therapy based on non-linear compartmental modeling. EJNMMI Res. (2016) 6:7. doi: 10.1186/s13550-016-0164-0
84. Osborne JR, Green DA, Spratt DE, Lyashchenko S, Fareedy SB, Robinson BD, et al. A prospective pilot study of (89)Zr-J591/prostate specific membrane antigen positron emission tomography in men with localized prostate cancer undergoing radical prostatectomy. J Urol. (2014) 191:1439-45. doi: 10.1016/j.juro.2013.10.041
85. Pandit-Taskar N, O'Donoghue JA, Durack JC, Lyashchenko SK, Cheal SM, Beylergil V, et al. A phase I/II study for analytic validation of 89Zr-J591 immunoPET as a molecular imaging agent for metastatic prostate cancer. Clin Cancer Res. (2015) 21:5277-85. doi: 10.1158/1078-0432.CCR-15-0552
86. Menke-van der Houven van Oordt CW, Gootjes EC, Huisman MC, Vugts DJ, Roth C, Luik AM, et al. 89Zr-cetuximab PET imaging in patients with advanced colorectal cancer. Oncotarget. (2015) 6:30384-93. doi: 10.18632/oncotarget.4672
87. Even AJG, Hamming-Vrieze O, van Elmpt W, Winnepenninckx VJL, Heukelom J, Tesselaar MET, et al. Quantitative assessment of Zirconium-89 labeled cetuximab using PET/CT imaging in patients with advanced head and neck cancer: a theragnostic approach. Oncotarget. (2017) 8:3870-80. doi: 10.18632/oncotarget.13910
88. van Loon J, Even AJG, Aerts HJWL, Öllers M, Hoebers F, van Elmpt W, et al. PET imaging of zirconium-89 labelled cetuximab: a phase I trial in patients with head and neck and lung cancer. Radiother Oncol J Eur Soc Ther Radiol Oncol. (2017) 122:267-73. doi: 10.1016/j.radonc.2016.11.020
89. Nayak TK, Brechbiel MW. 86Y based PET radiopharmaceuticals: radiochemistry and biological applications. Med Chem Shariqah United Arab Emir. (2011) 7:380-8. doi: 10.2174/157340611796799249
90. Rizvi SNF, Visser OJ, Vosjan MJWD, van Lingen A, Hoekstra OS, Zijlstra JM, et al. Biodistribution, radiation dosimetry and scouting of 90Y-ibritumomab tiuxetan therapy in patients with relapsed B-cell non-Hodgkin's lymphoma using 89Zr-ibritumomab tiuxetan and PET. Eur J Nucl Med Mol Imaging. (2012) 39:512-20. doi: 10.1007/s00259-011-2008-5
91. Muylle K, Flamen P, Vugts DJ, Guiot T, Ghanem G, Meuleman N, et al. Tumour targeting and radiation dose of radioimmunotherapy with (90)Y-rituximab in CD20+ B-cell lymphoma as predicted by (89)Zr-rituximab immuno-PET: impact of preloading with unlabelled rituximab. Eur J Nucl Med Mol Imaging. (2015) 42:1304-14. doi: 10.1007/s00259-015-3025-6
92. Jauw YWS, Zijlstra JM, de Jong D, Vugts DJ, Zweegman S, Hoekstra OS, et al. Performance of 89Zr-labeled-rituximab-PET as an imaging biomarker to assess CD20 targeting: a pilot study in patients with relapsed/refractory diffuse large B cell lymphoma. PLoS ONE. (2017) 12:e0169828. doi: 10.1371/journal.pone.0169828
93. Ulaner GA, Sobol NB, O'Donoghue JA, Kirov AS, Riedl CC, Min R, et al. CD38-targeted immuno-PET of multiple myeloma: from xenograft models to first-in-human imaging. Radiology. (2020) 295:606-15. doi: 10.1148/radiol.2020192621
94. Krishnan A, Adhikarla V, Poku EK, Palmer J, Chaudhry A, Biglang-Awa VE, et al. Identifying CD38+ cells in patients with multiple myeloma: first-in-human imaging using copper-64-labeled daratumumab. Blood Adv. (2020) 4:5194-202. doi: 10.1182/bloodadvances.2020002603
95. Farwell MD, Gamache RF, Babazada H, Hellmann MD, Harding JJ, Korn R, et al. CD8-Targeted PET imaging of tumor-infiltrating T cells in patients with cancer: a phase I first-in-humans study of 89Zr-Df-IAB22M2C, a radiolabeled anti-CD8 minibody. J Nucl Med. (2022) 63:720-6. doi: 10.2967/jnumed.121.262485
96. Wu AM, Pandit-Taskar N. ImmunoPET: harnessing antibodies for imaging immune cells. Mol Imaging Biol. (2022) 24:181-97. doi: 10.1007/s11307-021-01652-7
97. Qin T, Zeng Y duo, Qin G, Xu F, Lu J bin, Fang W feng, et al. High PD-L1 expression was associated with poor prognosis in 870 Chinese patients with breast cancer. Oncotarget. (2015) 6:33972-81. doi: 10.18632/oncotarget.5583
98. Yang J, Dong M, Shui Y, Zhang Y, Zhang Z, Mi Y, et al. A pooled analysis of the prognostic value of PD-L1 in melanoma: evidence from 1062 patients. Cancer Cell Int. (2020) 20:96. doi: 10.1186/s12935-020-01187-x
99. Natarajan A, Mayer AT, Reeves RE, Nagamine CM, Gambhir SS. Development of novel immunoPET tracers to image human PD-1 checkpoint expression on tumor-infiltrating lymphocytes in a humanized mouse model. Mol Imaging Biol. (2017) 19:903-14. doi: 10.1007/s11307-017-1060-3
100. England CG, Ehlerding EB, Hernandez R, Rekoske BT, Graves SA, Sun H, et al. Preclinical pharmacokinetics and biodistribution studies of 89zr-labeled pembrolizumab. J Nucl Med. (2017) 58:162-8. doi: 10.2967/jnumed.116.177857
101. Kok IC, Hooiveld JS, van de Donk PP, Giesen D, van der Veen EL, Lub-de Hooge MN, et al. 89Zr-pembrolizumab imaging as a non-invasive approach to assess clinical response to PD-1 blockade in cancer. Ann Oncol. (2022) 33:80-8. doi: 10.1016/j.annonc.2021.10.213
102. Bensch F, van der Veen EL, Lub-de Hooge MN, Jorritsma-Smit A, Boellaard R, Kok IC, et al. 89Zr-atezolizumab imaging as a non-invasive approach to assess clinical response to PD-L1 blockade in cancer. Nat Med. (2018) 24:1852-8. doi: 10.1038/s41591-018-0255-8
103. Smit J, Borm FJ, Niemeijer ALN, Huisman MC, Hoekstra OS, Boellaard R, et al. PD-L1 PET/CT imaging with radiolabeled durvalumab in patients with advanced-stage non-small cell lung cancer. J Nucl Med. (2022) 63:686-93. doi: 10.2967/jnumed.121.262473
104. Bahce I, Huisman MC, Verwer EE, Ooijevaar R, Boutkourt F, Vugts DJ, et al. Pilot study of (89)Zr-bevacizumab positron emission tomography in patients with advanced non-small cell lung cancer. EJNMMI Res. (2014) 4:35. doi: 10.1186/s13550-014-0035-5
Keywords: nuclear medicine, immuno-PET, diagnosis, theranostic, monoclonal antibody
Citation: Lugat A, Bailly C, Chérel M, Rousseau C, Kraeber-Bodéré F, Bodet-Milin C and Bourgeois M (2022) Immuno-PET: Design options and clinical proof-of-concept. Front. Med. 9:1026083. doi: 10.3389/fmed.2022.1026083
Received: 23 August 2022; Accepted: 29 September 2022;
Published: 14 October 2022.
Edited by:
Francisca Mulero, Spanish National Cancer Research Center (CNIO), SpainReviewed by:
Weijun Wei, Shanghai Jiao Tong University, ChinaCopyright © 2022 Lugat, Bailly, Chérel, Rousseau, Kraeber-Bodéré, Bodet-Milin and Bourgeois. This is an open-access article distributed under the terms of the Creative Commons Attribution License (CC BY). The use, distribution or reproduction in other forums is permitted, provided the original author(s) and the copyright owner(s) are credited and that the original publication in this journal is cited, in accordance with accepted academic practice. No use, distribution or reproduction is permitted which does not comply with these terms.
*Correspondence: Mickaël Bourgeois, Ym91cmdlb2lzQGFycm9uYXgtbmFudGVzLmZy
Disclaimer: All claims expressed in this article are solely those of the authors and do not necessarily represent those of their affiliated organizations, or those of the publisher, the editors and the reviewers. Any product that may be evaluated in this article or claim that may be made by its manufacturer is not guaranteed or endorsed by the publisher.
Research integrity at Frontiers
Learn more about the work of our research integrity team to safeguard the quality of each article we publish.