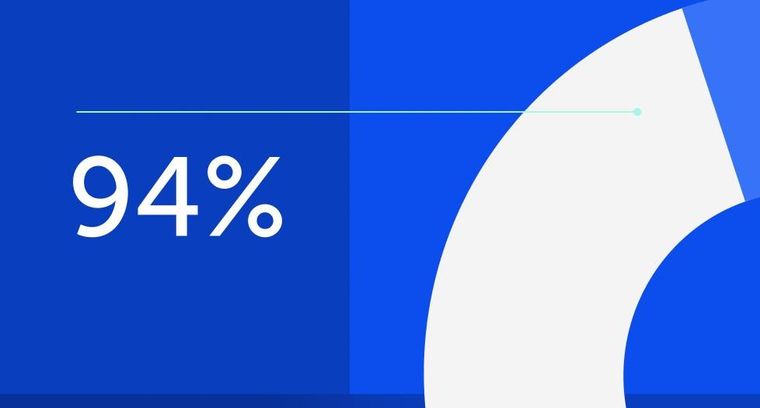
94% of researchers rate our articles as excellent or good
Learn more about the work of our research integrity team to safeguard the quality of each article we publish.
Find out more
MINI REVIEW article
Front. Med., 20 October 2022
Sec. Ophthalmology
Volume 9 - 2022 | https://doi.org/10.3389/fmed.2022.1023062
This article is part of the Research TopicOptical Coherence Tomography and OCT Angiography Examination in Systemic DiseasesView all 8 articles
Sepsis is a severe illness which results in alterations in the end organ microvascular haemodynamics and is associated with a high risk of mortality. There is currently no real-time method of monitoring microcirculatory perfusion during sepsis. Retinal microcirculation is closely linked to cerebral perfusion and may reflect systemic vascular alterations. Retinal perfusion can be assessed using the non-invasive imaging technique of optical coherence tomography angiography (OCTA). This narrative review aims to discuss the utility of using retinal imaging and OCTA in systemic illness and sepsis. OCTA can be used as a functional, non-invasive and real-time biomarker along with other haemodynamic parameters for assessing and managing patients with sepsis.
Sepsis is a dysregulated response to infection causing widespread inflammation, leading to life-threatening organ dysfunction (1). Estimates suggest that 147,000 individuals (10,000 of which are children) are admitted with sepsis annually in the UK, with sepsis killing at least 46,000 people each year (2). Sepsis can progress to septic shock with high mortality and is associated with profound circulatory and metabolic abnormalities (1), resulting in multiple organ failure (3, 4) if not intervened early.
No single diagnostic test can definitively establish the diagnosis of sepsis or septic shock. Diagnosis is clinical and relies on early recognition of hypotension, high cardiac output, and end-organ damage. The quick Sequential Organ Failure Assessment (qSOFA) score is a fast scoring method to identify patients with suspected infection who are likely to die in hospital, and which takes into consideration respiratory rate, altered mentation, and systolic blood pressure (BP) (1). The SOFA score is an expansion of the qSOFA and is used for patients with suspected infection within the intensive therapy unit (ITU) to determine the extent of the end-organ failure and mortality associated with sepsis (1).
Altered microvascular blood flow is a core feature of sepsis (5–7), involving reduced functional capillary density and faulty capillary perfusion, ultimately reducing the amount of oxygen delivered to essential organs, causing organ dysfunction and critical organ failure (3, 8).
Fluid resuscitation is a standard intervention to treat septic patients and prevent end-organ dysfunction, such as renal failure, which is a significant cause of sepsis-induced morbidity and mortality (9). However, using inotropes and fluids has variable results (10).
There is currently no real-time method to monitor microcirculatory reperfusion in septic patients, which would be beneficial for monitoring sepsis progression and response to therapeutic intervention. This review discusses the potential for retinal blood flow as a surrogate marker for end-organ (brain) perfusion in sepsis.
The retinal vasculature arises directly from the cerebral blood vessels, as the internal carotid artery supplies the ophthalmic artery, which supplies the central retinal artery: the sole blood supply to the retina (11). Both retinal and cerebral blood flow depends on the balance between arterial perfusion pressure and resistance from vascular beds (12, 13), with vasculature in the eyes and brain responding to local metabolic factors to maintain ocular and cerebral perfusion pressure, respectively (14). Due to shared vessel anatomy and similarities in microcirculatory regulation, changes in retinal perfusion correlate with cerebral microcirculation in healthy patients and may do so in systemic diseases (14). The retina and brain showed similar blood flow changes in animal models of hemorrhagic shock and hypercapnia models (15–17). In healthy human subjects, retinal blood flow is affected similarly to cerebral blood flow by nimodipine (18).
Optical coherence tomography (OCT) and OCT angiography (OCTA) are non-invasive imaging techniques that allow high-resolution cross-sectional retinal imaging of its structure and blood flow respectively (19, 20), without an injection of contrast (21). OCTA can capture each distinct vascular bed of the retina, including the superficial vascular plexus, composed of larger arteries/arterioles and veins/venules supplied by the central retinal artery; and the intermediate capillary plexus (Figure 1) (22). OCT and OCTA scans are based on the estimation of light reflectivity and blood flow (23). The scans quickly acquire and offer instantaneous qualitative (and quantitative) data in some devices. Previous studies have shown the feasibility (24), stability (25) and optimal analysis methods for using OCT and OCTA in a critical care setting, suggesting these scans may offer sensitive and reproducible means to study retinal manifestations of systemic disease.
Figure 1. Optical coherence tomography angiography scans from the right eye. (A) En face superficial vascular plexus layer with the foveal avascular zone visible in the centre. (B) En face intermediate capillary plexus layer. (C) Cross-section of the retina, with blood flow visible in yellow. The added colour areas correspond to the borders of panels (A,B) to demonstrate the vascular bed locations.
Sepsis is a time sensitive critical illness, with early recognition and regular monitoring of progression proving pivotal to survival, especially when there may be haemodynamic instability (1). It is therefore important to have precise haemodynamic recording and analysis to ensure that the supportive interventions are adequate to improve outcomes. Myocardial and peripheral vascular dysfunction are equally important in sepsis, with functional assessment of cardiovascular reserve and vessel perfusion necessary to ensure that end-organ blood flow is maintained, ultimately preventing critical organ failure (26).
Monitoring mean arterial pressure (MAP) is a standard assessment in sepsis as it approximates organ perfusion pressure, with a MAP of <70 mmHg deemed a criteria point for sepsis (1). Cardiac output is also monitored during sepsis and acts as an indicator of response to treatment (26). Cardiac output measurements such as pulmonary artery catheter, aortic velocimetry, arterial waveform analysis and trans oesophageal echocardiography do not measure or estimate microcirculatory dysfunction in the brain (27). Markers of tissue perfusion such as mixed venous oxygen saturation and lactate estimate global hypoperfusion and not cerebral microcirculation (26).
Retinal blood flow has been investigated in many disease states which affect different organ systems, suggesting its potential for use as a biomarker across numerous diseases. The following study results are detailed in Table 1.
In a study involving patients with systemic hypertension, OCTA showed that patients with poorly controlled BP (148 ± 8 mmHg) had a significantly lower retinal capillary density in the deep vascular plexus than patients with well-controlled BP (125 ± 9 mmHg) (p = 0.039) (28). Similar findings have been shown in patients with hypertensive crisis (systolic BP ≥ 180 mmHg or diastolic BP ≥ 110 mmHg), with retinal deep vasculature vessel density (VD) and skeletonised VD from OCTA scans significantly reduced in patients compared with healthy controls (p = 0.004 and 0.002 respectively) (29). Patients with atrial fibrillation (AF) had significantly lower retinal flow density in the superficial and deep vasculature compared with healthy controls (p = 0.000 and 0.005 respectively) before pulmonary vein isolation treatment. After treatment, only flow density in the optic nerve head significantly improved (p = 0.007), showing that retinal perfusion was reduced in patients with AF, with OCTA offering novel information on global perfusion in AF patients (30).
Patients with chronic kidney disease (CKD) have differing retinal microvascular calibres depending on the disease stage (31). Using digital retinal imaging from fundus photographs, central retinal arteriolar or venular equivalent (CRAE or CRVE) were significantly lower in patients with stage 3 CKD than those with stage 2 CKD (p = 0.004), showing retinal vascular thinning in proportion to CKD severity, suggesting retinal vessel calibre may serve as a biomarker of CKD progression (31). Patients who undergo haemodialysis with hypotensive episodes in the 30 days after dialysis, had pre-dialysis measures showing a reduced foveal avascular zone (FAZ) area (p = 0.03), and a higher VD of the superficial and deep capillary plexus (p = 0.002–0.04), indicating higher retinal blood flow in these patients, compared with those who did not have hypotensive episodes (32). This suggests a single OCTA measurement may be able to stratify the risk of intradialytic hypotension.
After transcatheter aortic valve implantation, OCTA scans found new retinal capillary dropout in 28.6% of participants in both the superficial and deep capillary plexi (33), while vessel density and FAZ size remained stable between pre- and post-implantation scans, with authors suggesting lesions were too small to have significant overall blood flow effects (33).
Patients with Sjögren syndrome—a chronic systemic autoimmune disease—had reduced vessel density in the superficial and deep microvasculature (p < 0.05) compared with healthy controls, although as they only included 12 patients, a larger sample would be required to quantify vascular changes with disease progression (34).
Patients with chronic obstructive pulmonary disorder (COPD) had reduced retinal vascular density compared with healthy controls in the superficial and deep capillary plexi on OCTA (p = 0.002) (35). COPD patients also had a larger FAZ diameter than healthy participants (p = 0.002) (35), and there was a moderate correlation between partial pressure of oxygen (pO2) and superficial/deep vascular density (r = 0.559/0.800 respectively), and a highly negative correlation between pO2 and FAZ size (r = −0.876) (35), suggesting that retinal vascular density by OCTA may be useful to monitor disease progression in COPD patients (35). Retinal imaging in COPD has also shown that older age, higher systolic BP and lower levels of systemic inflammation are associated with narrower retinal venules (p < 0.05) (36).
These studies highlight the increasing use of retinal vasculature to investigate disease states, progression, and treatment response.
As cerebral and retinal perfusion are closely related, and sepsis primarily affects that microcirculation, the retina is expected to reflect disease progression during a septic episode. The following study results are detailed in Table 1.
Fluorescein angiography (FA) is a retinal imaging technique in which fluorescein dye is injected intravenously to visualise the superficial retinal vasculature (37). Thirty-one septic patients in ITU underwent FA to determine how retinal arterial filling time (RAFT) compared with macrohaemodynamic status (38). Patients with prolonged RAFT (>8.3 s) had a higher intraocular pressure (p = 0.029); lower cardiac index (CI) (p = 0.039); and lower levels of C-reactive protein (p = 0.011) than patients with a short RAFT (<8.3 s), although there were no differences when considering vasopressor dose, MAPs, or serum lactate levels (38). Septic patients with a prolonged RAFT, therefore, had an impaired inflammatory response compared with those who had a short RAFT, demonstrating the potential to indicate macro- and microcirculatory defects (38). Although FA is an invasive technique and carries risk of an adverse reaction to the fluorescein dye, the demonstration of FA showing microcirculatory defects further adds to the evidence that retinal perfusion may reflect systemic perfusion, especially as RAFT corresponded with haemodynamic measures.
Using a handheld fundus photography device, large retinal vessel calibres and density were calculated by CRAE and CRVE respectively, and in septic patients, the median CRAE was higher and vascular length density lower compared with healthy controls (p = 0.002 and p < 0.001 respectively), yet CRVE did not differ between the two groups (p = 0.396) (39). However, there was a significant correlation between CRVE and lactate levels (p = 0.005), arterial tortuosity and diastolic BP (p = 0.036), vascular length density and lactate (p = 0.047), and vascular length density and CI (p = 0.014). This use of fundus imaging—a non-invasive technique—showing retinal vascular changes in septic patients demonstrates the potential to use similar retinal imaging techniques in this cohort of patients. A strength of this technique is its ability to quantify the microvascular dysfunction reproducibly.
Incident dark field (IDF) imaging—a non-invasive technique using a green light source absorbed by haemoglobin to detect red blood cells—has been used to assess conjunctival microvascular blood flow (40). When comparing septic patients with healthy controls, and septic survivors against non-survivors, there were significant reductions (p < 0.001) in all IDF conjunctival microcirculation measures in septic patients compared with healthy controls. There was also significantly lower perfused VD in non-survivors compared with survivors at 24 h (p < 0.05). Non-survivors also had significantly lower retinal vascular length density (large vessels) by fundus photography at 6 and 24 h compared with baseline (p < 0.05) (40). This correlation between conjunctival and retinal flow changes in septic patients suggests the link between peripheral (conjunctival) and central (retinal) blood flow reduction during sepsis.
In patients with bilateral pneumonia caused by COVID-19 (41), OCTA scans showed FAZ area was significantly larger in COVID-19 patients in the superficial and deep vascular layers (p = 0.000) compared to healthy controls. VD was also reduced in the foveal area of the choriocapillaris (p = 0.046). However there was no difference between groups in either the superficial or deep plexus VD percentage (41).
While the previous studies have shown that retinal perfusion is altered in septic patients compared with healthy controls, it is important to investigate whether reduced retinal blood flow returns with resuscitation and treatment of sepsis, how this correlates with haemodynamic measures, and if this can be detected using non-invasive retinal imaging. The following study results are detailed in Table 1.
Researchers using a septic rat model calculated blood flow index (BFI) from retinal and choroidal OCTA scans at 6 and 24 h after induction (42) which showed both retinal and choroidal BFI reduced during shock which occurred in parallel with a decrease in MAP and an increase in blood lactate. In a rat haemorrhagic shock model, retinal and choroidal BFI reduced during shock and increased after resuscitation. MAP also decreased during shock and increased following resuscitation (42). This demonstrates that retinal blood flow may reflect systemic effects from haemorrhagic shock as it alters dynamically in correlation with haemodynamic markers, and that OCTA can detect these changes.
In an ovine model of haemorrhagic shock, MAP decreased significantly (p = 0.001) after indution and then increased close to baseline levels following resuscitation (p = 0.164). CI also decreased during shock (p = 0.051) and increased after resuscitation (p = 0.001), and central venous oxygen decreased during shock (p = 0.003) and increased to baseline levels after resuscitation (p = 0.027) (43). In the retina, OCTA flow density reduced during shock (p = 0.027) and returned to baseline levels following resuscitation (p = 0.027). Conjunctival flow also showed similar changes, with the proportion of perfused vessels reducing significantly during shock compared with baseline (p = 0.013), increasing following resuscitation to near baseline (p = 0.173). Microvascular flow index also decreased (p = 0.034) and then increased following resuscitation (p = 0.081). Further, the authors showed that flow density in the superficial retinal OCTA significantly correlated with changes to conjunctival microcirculation and haemodynamic measures (p = 0.002–0.014) (43). The changes to conjunctival blood flow demonstrate that conjunctival and retinal microcirculation may be able to reflect changes to systemic circulation during shock and therefore offer a non-invasive marker of sepsis or shock progression and treatment response due to the return of flow following resuscitation.
Sepsis is a life-threatening condition with high mortality risk without prompt recognition and treatment. Currently, there is no validated, reproducible method to measure the microcirculation in sepsis. OCTA can potentially monitor haemodynamic resuscitation by targeting good capillary perfusion. In an ideal world of precision medicine, such targeted therapy has the potential to improve outcomes in sepsis and other low-flow states. Several studies have demonstrated retinal blood flow changes in systemic illnesses, including sepsis, demonstrating the potential for OCTA to provide microcirculatory monitoring. When considering this with the studies that demonstrate retinal blood flow returns to baseline in septic models after resuscitation (41, 42), retinal blood flow could be used as a marker for sepsis progression and resuscitation. Further, these imaging techniques used were non-invasive and fast, so they have minimal impact on patients. Further studies on the responsiveness of retinal blood flow following resuscitation in septic patients are essential, but preliminary studies are promising (38–40).
In addition to reporting retinal blood flow alterations in systemic disease (29, 30, 33–35), Kannenkeril et al. also indicate that OCTA changes are associated with disease progression in CKD (31), showing promise as a marker of disease severity in other systemic disorders affecting the microcirculation. Coppolino et al. (32) found an association between pre-dialysis retinal VD and patients who subsequently experienced intradialytic hypotension, demonstrating prognostic value and concluding that OCTA could stratify the short-term risk of this complication. OCTA flow density correlated with IDF conjunctival microcirculation in addition to haemodynamic measures during haemorrhagic shock and after resuscitation, suggesting that peripheral microcirculation as seen in the conjunctiva changed in association with central microcirculation as seen in the retina (43). Both measures could therefore serve as non-invasive markers of microcirculation during sepsis and critical illness, but this finding requires validation in human studies.
There are gaps in this area of research, with the main limitation of small sample sizes in human studies. Different retinal blood flow metrics were used in each study, which makes comparison difficult, as well as different imaging techniques used and different OCTA manufacturers between studies. It would also be beneficial to measure OCT and OCTA sequentially in systemically ill and septic patients to investigate the extent of perfusion dysfunction and its correlation with neurocognitive dysfunction. Future studies would benefit from larger cohorts of patients, and it would be of value to include patients in different severities of sepsis to investigate how retinal perfusion may be different.
Using OCTA to visualise retinal blood flow is a promising method of monitoring microcirculation during sepsis and response to resuscitation. Further human studies are warranted to determine how this could be implemented in a cohort of septic patients.
EC was a major contributor in writing the manuscript. All authors read, edited and approved the final manuscript.
This study was funded by the National Institute for Health Research (NIHR) Surgical Reconstruction and Microbiology Research Centre (SRMRC). TV’s research programme was supported by Medical Research Council and NIHR.
The authors declare that the research was conducted in the absence of any commercial or financial relationships that could be construed as a potential conflict of interest.
All claims expressed in this article are solely those of the authors and do not necessarily represent those of their affiliated organizations, or those of the publisher, the editors and the reviewers. Any product that may be evaluated in this article, or claim that may be made by its manufacturer, is not guaranteed or endorsed by the publisher.
The views expressed are those of the author(s) and not necessarily those of the NIHR or the Department of Health and Social Care.
qSOFA, quick Sequential Organ Failure Assessment; BP, blood pressure; SOFA, Sequential Organ Failure Assessment; ITU, intensive therapy unit; OCT, optical coherence tomography; OCTA, optical coherence tomography angiography; MAP, mean arterial pressure; VD, vessel density; AF, atrial fibrillation; CKD, chronic kidney disease; CRAE, central retinal arteriolar equivalent; CRVE, central retinal venular equivalent; FAZ, foveal avascular zone; COPD, chronic obstructive pulmonary disorder; pO2, partial pressure of oxygen; FA, fluorescein angiography; RAFT, retinal arterial filling time; CI, cardiac index; IDF, incident dark field; BFI, blood flow index.
1. Singer M, Deutschman CS, Seymour CW, Shankar-Hari M, Annane D, Bauer M, et al. The third international consensus definitions for sepsis and septic shock (Sepsis-3). JAMA. (2016) 315:801. doi: 10.1001/jama.2016.0287
2. Hex N. Whitewater Charitable Trust The Cost of Sepsis Care in the UK Final Report. New York, NY: York Health Economics Consortium (2017).
3. De Backer D, Orbegozo Cortes D. Pathophysiology of microcirculatory dysfunction and the pathogenesis of septic shock. Virulence. (2014) 5:73–9. doi: 10.4161/viru.26482
4. Trzeciak S, McCoy JV, Dellinger RP, Arnold RC, Rizzuto M, Abate NL, et al. Early increases in microcirculatory perfusion during protocol-directed resuscitation are associated with reduced multi-organ failure at 24 hours in patients with sepsis. Intensive Care Med. (2008) 34:2210. doi: 10.1007/s00134-008-1193-6
5. Farquhar I, Martin CM, Lam C, Potter R, Ellis CG, Sibbald WJ. Decreased capillary densityin vivoin bowel mucosa of rats with normotensive sepsis. J Surg Res. (1996) 61:190–6. doi: 10.1006/jsre.1996.0103
6. Verdant CL, De Backer D, Bruhn A, Clausi CM, Su F, Wang Z, et al. Evaluation of sublingual and gut mucosal microcirculation in sepsis: a quantitative analysis. Crit Care Med. (2009) 37:2875–81. doi: 10.1097/CCM.0b013e3181b029c1
7. De Backer D, Donadello K, Taccone FS, Ospina-Tascon G, Salgado D, Vincent J-L. Microcirculatory alterations: potential mechanisms and implications for therapy. Ann Intensive Care. (2011) 1:27. doi: 10.1186/2110-5820-1-27
8. De Backer D, Donadello K, Sakr Y, Ospina-Tascon G, Salgado D, Scolletta S, et al. Microcirculatory alterations in patients with severe sepsis: impact of time of assessment and relationship with outcome. Crit Care Med. (2013) 41:791–9. doi: 10.1097/CCM.0b013e3182742e8b
9. Hotchkiss RS, Moldawer LL, Opal SM, Reinhart K, Turnbull IR, Vincent JL. Sepsis and septic shock. Nat Rev Dis Prim. (2016) 2:16045. doi: 10.1038/nrdp.2016.45
10. Asfar P, Meziani F, Hamel J-F, Grelon F, Megarbane B, Anguel N, et al. High versus low blood-pressure target in patients with septic shock. N Engl J Med. (2014) 370:1583–93. doi: 10.1056/NEJMoa1312173
11. Remington, LA. Orbital blood supply. Clinical Anatomy and Physiology of the Visual System. St. Louis, MI: Elsevier (2011) 202–17. doi: 10.1016/B978-1-4377-1926-0.10011-6
12. Bill A, Sperber GO. Control of retinal and choroidal blood flow. Eye. (1990) 4:319–25. doi: 10.1038/eye.1990.43
13. Cipolla MJ. The Cerebral Circulation. Morgan and Claypool Life Sciences ISBN 1-61504-013-7. San Rafael, CA: Morgan and Claypool Life Sciences (2010).
14. Courtie E, Veenith T, Logan A, Denniston AK, Blanch RJ. Retinal blood flow in critical illness and systemic disease: a review. Ann Intensive Care. (2020) 10:1–18. doi: 10.1186/s13613-020-00768-3
15. Linder J. Effects of cervical sympathetic stimulation on cerebral and ocular blood flows during hemorrhagic hypotension and moderate hypoxia. Acta Physiol Scand. (1982) 114:379–86. doi: 10.1111/j.1748-1716.1982.tb06998.x
16. Stiris T, Odden JP, Hansen TWR, Hall C, Bratlid D. The effect of arterial Pco2-variations on ocular and cerebral blood flow in the newborn piglet. Pediatr Res. (1989) 25:205–8. doi: 10.1203/00006450-198902000-00025
17. Pouliot M, Deschênes MC, Hétu S, Chemtob S, Lesk MR, Couture R, et al. Quantitative and regional measurement of retinal blood flow in rats using N-isopropyl-p-[14C]-iodoamphetamine ([14C]-IMP). Exp Eye Res. (2009) 89:960–6. doi: 10.1016/j.exer.2009.08.005
18. Michelson G, Wärntges S, Leidig S, Lötsch J, Geisslinger G. Nimodipine plasma concentration and retinal blood flow in healthy subjects. Invest Ophthalmol Vis Sci. (2006) 47:3479–86. doi: 10.1167/iovs.05-1350
19. Adhi M, Duker JS. Optical coherence tomography–current and future applications. Curr Opin Ophthalmol. (2013) 24:213–21. doi: 10.1097/ICU.0b013e32835f8bf8
20. Chen C-L, Wang RK. Optical coherence tomography based angiography [Invited]. Biomed Opt Express. (2017) 8:1056. doi: 10.1364/BOE.8.001056
21. Shin YU, Lee DE, Kang MH, Seong M, Yi J-H, Han S-W, et al. Optical coherence tomography angiography analysis of changes in the retina and the choroid after haemodialysis. Sci Rep. (2018) 8:17184. doi: 10.1038/s41598-018-35562-6
22. Campbell JP, Zhang M, Hwang TS, Bailey ST, Wilson DJ, Jia Y, et al. Detailed vascular anatomy of the human retina by projection-resolved optical coherence tomography angiography. Sci Rep. (2017) 7:42201. doi: 10.1038/srep42201
23. Wylęgała A. Principles of OCTA and applications in clinical neurology. Curr Neurol Neurosci Rep. (2018) 18:96. doi: 10.1007/s11910-018-0911-x
24. Liu X, Kale AU, Capewell N, Talbot N, Ahmed S, Keane PA, et al. Original research: optical coherence tomography (OCT) in unconscious and systemically unwell patients using a mobile OCT device: a pilot study. BMJ Open. (2019) 9:1–7. doi: 10.1136/bmjopen-2019-030882
25. Courtie EF, Kale AU, Hui BTK, Liu X, Capewell NI, Bishop JRB, et al. Stability of OCT and OCTA in the intensive therapy unit setting. Diagnostics. (2021) 11:1516. doi: 10.3390/diagnostics11081516
26. Valeanu L, Bubenek-Turconi SI, Ginghina C, Balan C. Hemodynamic monitoring in sepsis—a conceptual framework of macro- and microcirculatory alterations. Diagnostics. (2021) 11:1559. doi: 10.3390/diagnostics11091559
27. Jhanji S, Dawson J, Pearse RM. Cardiac output monitoring: basic science and clinical application. Anaesthesia. (2008) 63:172–81. doi: 10.1111/j.1365-2044.2007.05318.x
28. Chua J, Chin CWL, Hong J, Chee ML, Le TT, Ting DSW, et al. Impact of hypertension on retinal capillary microvasculature using optical coherence tomographic angiography. J Hypertens. (2019) 37:572–80. doi: 10.1097/HJH.0000000000001916
29. Terheyden JH, Wintergerst MWM, Pizarro C, Pfau M, Turski GN, Holz FG, et al. Retinal and choroidal capillary perfusion are reduced in hypertensive crisis irrespective of retinopathy. Transl Vis Sci Technol. (2020) 9:42. doi: 10.1167/tvst.9.8.42
30. Lange PS, Mihailovic N, Esser E, Frommeyer G, Fischer AJ, Bode N, et al. Improvement of retinal microcirculation after pulmonary vein isolation in patients with atrial fibrillation—an optical coherence tomography angiography study. Diagnostics. (2022) 12:38. doi: 10.3390/diagnostics12010038
31. Kannenkeril D, Frost S, Nolde JM, Kiuchi MG, Carnagarin R, Lugo-Gavidia LM, et al. Microvascular changes at different stages of chronic kidney disease. J Clin Hypertens. (2021) 23:309–16. doi: 10.1111/jch.14138
32. Coppolino G, Carnevali A, Gatti V, Battaglia C, Randazzo G, Figlia I, et al. OCT angiography metrics predict intradialytic hypotension episodes in chronic hemodialysis patients: a pilot, prospective study. Sci Rep. (2021) 11:7202. doi: 10.1038/s41598-021-86609-0
33. Gunzinger JM, Ibrahimi B, Baur J, Wiest MRJ, Picirelli M, Pangalu A, et al. Assessment of retinal capillary dropout after transcatheter aortic valve implantation by optical coherence tomography angiography. Diagnostics. (2021) 11:2399. doi: 10.3390/diagnostics11122399
34. Yang QC, Yao F, Li QY, Chen MJ, Zhang LJ, Shu HY, et al. Ocular microvascular alteration in sjögren syndrome. Quant Imaging Med Surg. (2022) 12:1324. doi: 10.21037/qims-21-234
35. Songur MS, İntepe YS, Bayhan SA, Bayhan HA, Çiftçi B, Çıtırık M. The alterations of retinal vasculature detected on optical coherence tomography angiography associated with chronic obstructive pulmonary disease. Clin Respir J. (2022) 16:284–92. doi: 10.1111/crj.13478
36. Vaes AW, Spruit MA, Van Keer K, Barbosa-Breda J, Wouters EFM, Franssen FME, et al. Structural analysis of retinal blood vessels in patients with COPD during a pulmonary rehabilitation program. Sci Rep. (2020) 10:31. doi: 10.1038/s41598-019-56997-5
37. Spaide RF, Klancnik JM. Retinal vascular layers imaged by fluorescein angiography and optical coherence tomography angiography. JAMA Ophthalmol. (2015) 133:45. doi: 10.1001/jamaophthalmol.2014.3616
38. Erikson K, Liisanantti JH, Hautala N, Koskenkari J, Kamakura R, Herzig KH, et al. Retinal arterial blood flow and retinal changes in patients with sepsis: preliminary study using fluorescein angiography. Crit Care. (2017) 21:86. doi: 10.1186/s13054-017-1676-3
39. Simkiene J, Pranskuniene Z, Patasius M, Trumpaitis J, Boerma EC, Pranskunas A. Alterations of retinal vessels in patients with sepsis. J Clin Monit Comput. (2019) 34:1–6. doi: 10.1007/s10877-019-00401-0
40. Simkiene J, Pranskuniene Z, Vitkauskiene A, Pilvinis V, Boerma EC, Pranskunas A. Ocular microvascular changes in patients with sepsis: a prospective observational study. Ann Intensive Care. (2020) 10:38. doi: 10.1186/s13613-020-00655-x
41. Parisi V, Carnevali A, Scorcia V, Giannaccare G, Kal M, Winiarczyk M, et al. Retinal microvascular changes in COVID-19 bilateral pneumonia based on optical coherence tomography angiography. J Clin Med. (2022) 23:3621. doi: 10.3390/jcm11133621
42. Park JR, Lee BK, Lee MJ, Kim K, Oh WY. Visualization of three-dimensional microcirculation of rodents’ retina and choroid for studies of critical illness using optical coherence tomography angiography. Sci Rep. (2021) 11:14302. doi: 10.1038/s41598-021-93631-9
Keywords: optical coherence tomography angiography, OCTA, sepsis, critical illness, retinal blood flow
Citation: Courtie E, Gilani A, Veenith T and Blanch RJ (2022) Optical coherence tomography angiography as a surrogate marker for end-organ resuscitation in sepsis: A review. Front. Med. 9:1023062. doi: 10.3389/fmed.2022.1023062
Received: 19 August 2022; Accepted: 03 October 2022;
Published: 20 October 2022.
Edited by:
Maddalena De Bernardo, University of Salerno, ItalyReviewed by:
Bernardes Rui, University of Coimbra, PortugalCopyright © 2022 Courtie, Gilani, Veenith and Blanch. This is an open-access article distributed under the terms of the Creative Commons Attribution License (CC BY). The use, distribution or reproduction in other forums is permitted, provided the original author(s) and the copyright owner(s) are credited and that the original publication in this journal is cited, in accordance with accepted academic practice. No use, distribution or reproduction is permitted which does not comply with these terms.
*Correspondence: Richard J. Blanch, ci5qLmJsYW5jaEBiaGFtLmFjLnVr; Tonny Veenith, dC52LnZlZW5pdGhAYmhhbS5hYy51aw==
†These authors share last authorship
Disclaimer: All claims expressed in this article are solely those of the authors and do not necessarily represent those of their affiliated organizations, or those of the publisher, the editors and the reviewers. Any product that may be evaluated in this article or claim that may be made by its manufacturer is not guaranteed or endorsed by the publisher.
Research integrity at Frontiers
Learn more about the work of our research integrity team to safeguard the quality of each article we publish.