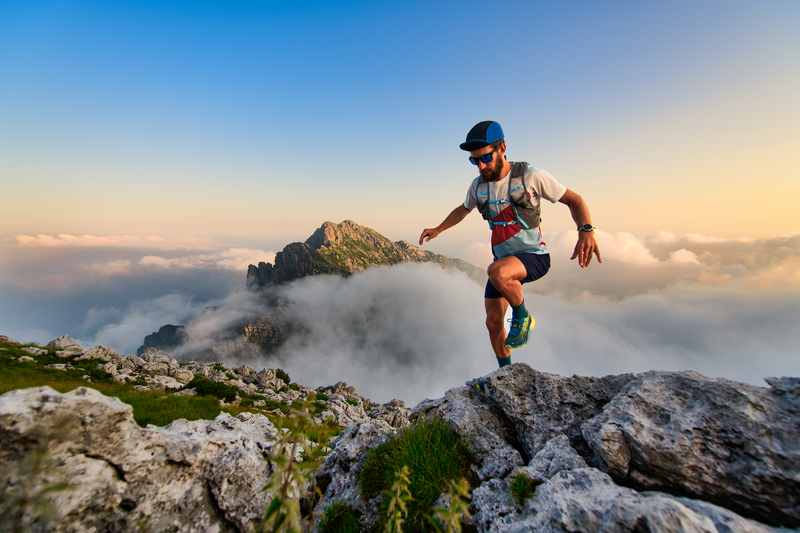
94% of researchers rate our articles as excellent or good
Learn more about the work of our research integrity team to safeguard the quality of each article we publish.
Find out more
ORIGINAL RESEARCH article
Front. Med. , 06 December 2022
Sec. Intensive Care Medicine and Anesthesiology
Volume 9 - 2022 | https://doi.org/10.3389/fmed.2022.1017371
This article is part of the Research Topic Post COVID-19: Analysing and Addressing the Challenges Faced by Patients Following Intensive Care Treatment for COVID-19 View all 16 articles
Background: Intensive care unit (ICU) prolonged immobilization may lead to lower-extremity muscle deconditioning among critically ill patients, particularly more accentuated in those with 2019 Novel Coronavirus (COVID-19) infection. Electrical stimulation (E-Stim) is known to improve musculoskeletal outcomes. This phase I double-blinded randomized controlled trial examined the safety and efficacy of lower-extremity E-Stim to prevent muscle deconditioning.
Methods: Critically ill COVID-19 patients admitted to the ICU were randomly assigned to control (CG) or intervention (IG) groups. Both groups received daily E-Stim (1 h) for up to 14 days on both gastrocnemius muscles (GNMs). The device was functional in the IG and non-functional in the CG. Primary outcomes included ankle strength (Ankles) measured by an ankle-dynamometer, and GNM endurance (GNMe) in response to E-Stim assessed with surface electromyography (sEMG). Outcomes were measured at baseline, 3 and 9 days.
Results: Thirty-two (IG = 16, CG = 16) lower extremities in 16 patients were independently assessed. The mean time between ICU admission and E-Stim therapy delivery was 1.8 ± 1.9 days (p = 0.29). At 3 days, the IG showed an improvement compared to the CG with medium effect sizes for Ankles (p = 0.06, Cohen’s d = 0.77) and GNMe (p = 0.06, d = 0.69). At 9 days, the IG GNMe was significantly higher than the CG (p = 0.04, d = 0.97) with a 6.3% improvement from baseline (p = 0.029). E-Stim did not alter vital signs (i.e., heart/respiratory rate, blood saturation of oxygen), showed no adverse events (i.e., pain, skin damage, discomfort), nor interfere with ICU standard of care procedures (i.e., mechanical ventilation, prone rotation).
Conclusion: This study supports the safety and efficacy of early E-Stim therapy to potentially prevent deterioration of lower-extremity muscle conditions in critically ill COVID-19 patients recently admitted to the ICU. If confirmed in a larger sample, E-Stim may be used as a practical adjunctive therapy.
Clinical trial registration: [https://clinicaltrials.gov/], identifier [NCT04685213].
Bed rest and immobilization are time-honored treatments for managing trauma and acute or chronic illnesses. Problems arising from this treatment modality can complicate a primary disease, worsening the initial cause of admission (1). For instance, critically ill patients who require prolonged immobilization due to intensive care unit (ICU) stay often suffer from muscle weakness (2). Particularly, this condition may originate from neuro-myogenic disturbances in lower extremities (3, 4) that, when immobilized, major pathways involving inflammation, impaired oxygen delivery, and hyperglycemia arise (5, 6). These consequences are highly prevalent among hospitalized patients with 2019 Novel Coronavirus (COVID-19) in need of intensive care (7, 8). Particularly, this population receive concomitant standard therapy of paralytics and glucocorticoids that leads to inhibition of acetylcholine receptors in the neuromuscular junctions (9); ultimately, causing deleterious effects on the musculoskeletal metabolism (10).
Recent studies have explored the physiopathology of muscle wasting in critically ill COVID-19 patients (11). Cytokine storms, C-reactive protein, and pro-inflammatory molecules are thought to be part of the biological mechanism (12). These factors may induce endothelial damage and mitochondrial autophagy leading to myofibrillar breakdown (13). In the lower extremities, these consequences can contribute to muscle atrophy, weakness, functional impairment, and persistent symptoms that can last for up to 1 year following ICU discharge (14). Eventually these symptoms can increase fall risk, lack of independence, and quality of life deterioration (15, 16). Therefore, there is a need to implement a practical solution to prevent muscle deterioration of bedbound patients, particularly those with severe COVID-19 infection.
Physical therapy (PT) greatly benefits neuromuscular outcomes in patients with muscle deconditioning and weakness (17). However, reduced personnel and resources can be a limitation for ICU COVID-19 patients. Additionally, the rapid loss of muscle mass within hours after ICU admission (5) requires an immediate approach, making this condition time-dependent. One practical solution is the use of electrical stimulation (E-Stim) therapy. This modality prevents muscle deconditioning (18), improves muscle strength, and restores functionality in ICU patients (19). While it may be a suitable treatment to facilitate the rehabilitation pathways for COVID-19 patients (20), empirical evidence is needed (11). Today, this technology has been demonstrated to improve muscle strength in ICU COVID-19 patients (21). However, there is still a lack of randomized studies (22) to confirm its efficacy. Thus, it is unclear whether this adjunctive therapy prevents lower-extremity muscle deconditioning in ICU COVID-19 patients.
This study examines the potential safety and efficacy of lower-extremity E-Stim therapy to prevent lower-extremity muscle deconditioning in ICU COVID-19 patients. We hypothesized that patients receiving short-term E-Stim therapy will show significant improvement in lower-extremity outcomes [i.e., muscle endurance, ankle strength (Ankles), risk of fall] compared to those who do not receive it.
Critically ill COVID-19 patients admitted to the ICU due to acute respiratory failure at Baylor St. Luke’s Medical Center (BSLMC, Houston, TX, USA) were recruited in a phase I double-blinded randomized controlled trial. Recruitment was performed from December 2020 to March 2021 by research assistants (AZ-R and NR). The protocol of the study was registered on clinicaltrials.gov, Identifier: NCT04685213. This study followed the Consolidated Standards of Reporting Trials (CONSORT) guidelines for randomized clinical trials.
To be eligible, patients must have been admitted to the ICU due to COVID-19 infection within 3 days prior to initiating E-Stim therapy, received assisted ventilation therapy, and indicated bed rest for at least 7 days. These conditions were based on the judgment of clinical intensivist investigators (MS and JPH). Patients were excluded if they were medically paralyzed (i.e., rocuronium, cisatracurium) or under vasopressor therapy (i.e., norepinephrine, epinephrine, vasopressin) at the moment of enrollment; expected to be discharged from critical care in the next 24 h; had below the knee amputations or lower-extremity wounds; demand-type cardiac pacemaker, implanted defibrillator, or other implanted electronic devices; and any conditions that may interfere with outcomes or increase the risk of the use E-Stim based on the judgment of clinicians.
Patients were randomized (ratio: 1:1) to either control (CG) or intervention (IG) groups through a computer-generated list followed by sequential allocation. Participants and care providers were blinded to the group allocation. The IG received E-Stim through two electrode adhesive pads (2 cm × 2 cm, Conductive electrode pads, Avazzia Inc., Dallas, TX, USA) placed on proximal gastrocnemius muscle (GNM) (23) and Achilles tendon of each leg using a bio-electric stimulation technology (BEST®, Dallas, TX, USA) microcurrent platform [Tennant Biomodulator device (R), Dallas, TX, USA, Figure 1] for 1 h daily for up to 14 days. The CG was provided with an identical but non-functional device (placebo) for the same period. Therapy was delivered in supine position placing the head of the patient’s ICU bed between 30–45 degrees. In cases of prone positioning, E-Stim therapy was delivered placing the head of the patient’s ICU bed within 20° (24).
Figure 1. Study setup: electrical stimulation device, plugs and pads, and surface electromyography sensors. Participants received electrical stimulation through electrode adhesive pads placed on both proximal and distal gastrocnemius muscles using a bio-electric stimulation technology® (BEST) micro-current platform (Tennant Biomodulator®). Electrical stimulation (E-Stim) was active in the intervention group and non-functional in the control group. Two surface electromyography (Delsys Trigno Wireless EMG System, MA, USA) sensors were placed on the proximal lateral gastrocnemius of each lower extremity to evaluate muscular outcomes. Proximal medial gastrocnemius signal was also recorded, but not included for analysis. sEMG, surface electromyogram; GNM, gastrocnemius muscle; E-Stim, electrical stimulation.
The E-Stim application was set at 50 V with an interactive high voltage pulsed alternative current (HVPAC) in the shape of an asymmetrical damped sinusoidal biphasic pulsed waveform (25), which allows for muscle relaxation and avoids fatigue during therapy (26). An intensity level from 50 to 250 V has been previously FDA-cleared for the use of pain relief (25). The pulse duration was between 400 and 1400 microseconds (μs), and pulse frequency between 20 and 121 hertz (Hz). These same intensity level and pulse characteristics were shown to be harmless in a previously published clinical trial for lower-extremity ischemic lesions (27). E-Stim was discontinued if the patient presented rapid deterioration [i.e., arterial blood oxygen desaturation < 93% under ventilation assistance, hemodynamic instability, septic shock, thigh extracorporeal membrane oxygenation (ECMO) placement, or generalized gross edema] despite intensive care treatment. Intubation was not an indication for E-Stim discontinuation.
Surface Electromyography (sEMG, Delsys Trigno Wireless EMG System, MA, USA) was recorded bilaterally from the proximal lateral GNM (Figure 1) according to the Surface Electromyography for a Non-Invasive Assessment of Muscles (SENIAM) guidelines (28). Prior to electrode placement, the skin was cleaned with alcohol and prep gel (Nuprep, CO, USA) to minimize impedance. The raw sEMG signal was recorded at 2,000 Hz and filtered using a 4th order Butterworth band-pass filter with cutoff frequencies of 20 and 400 Hz (29, 30). The filtered sEMG data was full-wave rectified and smoothed using a moving average to estimate the sEMG linear envelope (29, 30). Furthermore, the area under the envelope was calculated to estimate the integrated EMG (iEMG) to quantify the level of muscular activity (31, 32). EMG analysis was performed using custom-made software programmed in MATLAB (The MathWorks Inc., Natick, MA, USA).
Lower-extremity muscle outcomes included voluntary and involuntary contraction metrics. First, in a standardized supine position (33), Ankles was determined by the average of three 5 s dorsiflexion maximum voluntary isometric contractions (MVIC) per 30 s of relaxation in-between (Figure 2) assessed with a dynamometer (RoMech Digital Hanging Scale). Second, GNM endurance [GNMe, defined as sustained muscle involuntary contraction (34)] in response to 5 min of E-Stim therapy was assessed with iEMG analysis.
Figure 2. A typical case comparison between a maximum voluntary contraction and involuntary contraction of the gastrocnemius muscle assessed via surface electromyogram. (A) Three 5–10 s dorsiflexion maximum voluntary contractions. (B) Three 5–10 s intervals of electrical stimulation set at 50 V. Both panels having a 5–10 s relaxation period between contractions.
Lower-extremity perfusion outcomes included plantar tissue oxygen saturation (SatO2), a surrogate of muscle oxygen consumption in response to E-Stim (35). SatO2 was measured using a validated Near Infra-red Spectroscopy (NIRS) camera (Snapshot NIR, KENT Imaging Inc., Calgary, AB, Canada) that detects an approximate value of real-time SatO2 level in superficial tissue. SatO2 levels were examined in the metatarsal area, including the five toes. Muscular and perfusion outcomes of the lower extremity were assessed at baseline, 3 and 9 days.
Lower-extremity functional outcome was the likelihood of falling assessment via Morse Fall Scale (MFS) (36). This scale is a standardized assessment performed by hospitalists at BSLMC that assesses functional aspects of the lower extremity such as ambulatory aid, gait, and transferring, among other features related to risk of falling. As the score increases, it indicates proportionally worse outcomes (low risk < 24; moderate risk 25–44; high risk > 45). The functional outcome was collected from the electronic medical records at baseline, and at the time of ICU discharge (i.e., home, hospital floor, or patient expiration) to assess overall impact of intervention on subjects. Electronic medical records were also reviewed to differentiate whether outcomes were associated with any demographic characteristics or comorbidities.
Safety outcomes included monitoring of vital signs (i.e., heart/respiratory rate, blood pressure, blood saturation of oxygen), and study-related adverse events (i.e., pain, skin damage, discomfort, non-compliance). Feasibility outcomes included average of patient E-Stim therapy completion, average of measured outcomes at each time point (i.e., 3 and 9 days) excluding non-study-related adverse events (i.e., death, intubation, deep vein thrombosis, rapid deterioration) (37, 38). Acceptability outcomes included interference with ongoing COVID-19 standard of care procedures (i.e., mechanical ventilation, prone rotation, PT, other clinical trials), and interaction with the ICU staff (i.e., nurses, respiratory and occupational therapists, nutritional specialists, machinery technicians).
The sample size was estimated based on a Najafi et al. study (39), in which the effectiveness of daily lower-extremity E-Stim demonstrated a significant improvement in motor performance (Cohen effect size, d = 1.35). To observe the benefit of functional E-Stim (IG) to prevent or improve lower-extremity muscle outcomes compared to non-functional (CG), we conducted a power analysis following a (1) Conservative effect size (Cohen’s d = 0.6); (2) 80% generated power; (3) Alpha of 5%; (4) two number of groups; and (5) two repeated measurements, utilizing G *Power software (version of 3.1.6) (40). Each lower extremity was considered as an independent sample due to the variability in muscular and vascular status (41, 42).
Shapiro–Wilk test (p > 0.05) was used to assess the normality of the data. Independent t-test was used for group comparison at baseline on normally distributed continuous demographics, clinical data, and sEMG parameters. Mann–Whitney U test was used if the assumption of normal distribution was not satisfied. For categorical variables, Chi-square test was used to compare between-group differences at baseline. The effect size for baseline continuous and categorical data were measured using Cohen’s d and Cramer’s V, respectively. Values ranging from 0.20 to 0.49 indicate small effects, and values between 0.50 and 0.79 indicate medium effects. Values ranging from 0.80 to 1.29 indicate large effects, and values above 1.30 indicate very large effects. Generalized estimating equations (GEE) was used to test the main effect of group (two levels: CG and IG), time [two levels: baseline, 3/9 days (muscle and perfusion outcomes), or discharge time (MFS Score)], and their interaction on the outcome measures. For all tests, an alpha level of < 0.05 was considered statistically significant. All calculations were made using IBM SPSS Statistics 27 (IBM, IL, USA).
This study was approved by the local Institutional Review Board (IRB) at Baylor College of Medicine (Houston, TX, USA) in accordance with the Declaration of Helsinki (approval number H-47781). All participants read and signed the IRB-approved informed consent forms before initiating assessments or data collection. If the participant was cognitively impaired, consenting was performed via telephone call with a legal representative. The informed consent was obtained from all participants and/or their legal guardians.
The progress through the phases of screening, allocation, follow-up, and data analysis is shown in Figure 3. The vast majority of patients were excluded from initial screening due to anticipated discharge from critical care within 24 h. Nineteen participants satisfied the inclusion and exclusion criteria. From these, three were withdrawn due to rapid deterioration before the mid-point (3 days), leaving a total of 16 participants (Age = 64.8 ± 9.5, p = 0.43, d = 0.40) for analysis. Therefore, eight participants (n = 16 lower extremities) were allocated to the CG and eight participants (n = 16 lower extremities) to the IG. Baseline characteristics showed the IG group had significantly higher fasting glucose (p < 0.01, d = 1.767) than the CG. All other baseline characteristics were not significantly different between the groups (Table 1). The mean time between ICU admission and delivery of E-Stim therapy was 1.8 ± 1.9 days (p = 0.61, d = 0.25). At the study enrollment, the mean body SatO2 (SpO2) was 80.53 ± 13.38% (p = 0.27, d = 0.56) in the complete cohort with all the participants (100%) undergoing corticosteroid therapy. Limited or null mobility persisted for a mean of 3.3 ± 3.5 days (p = 0.54, d = 0.38) and after 7.5 ± 5.7 days (p = 0.93, d = 0.05), 68.7% (p = 0.59, V = 0.13) of the participants began vigorous activity involvement (i.e., physical/occupational therapy, standing up, walking to chair). All others (31.3%) remained immobile during their ICU stay.
Figure 3. Consort flow diagram. N, number of patients. n, number of lower extremities. ECMO, extracorporeal membrane oxygenation. First time point = 3 days. Second time point = 9 days.
At 3 days, the IG showed a non-significant improvement compared to the CG with medium effect sizes for Ankles (p = 0.06, d = 0.77, Figure 4A) and GNMe (p = 0.06, d = 0.69, Figure 4B), whereas the CG showed a non-significant deterioration for GNMe in comparison to baseline (−3.9%, p = 0.08). At 9 days, the IG showed a significant improvement compared to the CG with large effect size for GNMe (p = 0.04, d = 0.97, Figure 4C). In comparison to baseline, the IG’s GNMe showed a significant improvement (+6.3%, p = 0.029). Lower-extremity oxygen consumption (SatO2) values remained stable between and within groups through time (p > 0.05, Table 2). At the time of ICU discharge, the IG showed a significant improvement compared to the CG with small effect size for MFS score (p = 0.05, d = 0.36, Figure 4D). In comparison to baseline, the IG’s MFS score showed a significant improvement (−12.7%, p = 0.05), opposite to the CG, which showed a significant worsening score (48.1%, p = 0.04). All other parameter comparison are shown in Table 2.
Figure 4. Comparison of outcomes within and between groups through time. Ankles, ankle strength; kg, kilograms; GNMe, gastrocnemius muscle endurance; iEMG, integrated electromyography unit; MFS, Morse Fall Risk Scale. (A) Comparison of Ankles, and (B) GNMe within and between groups at 3 days from baseline. (C) Comparison of GNMe within and between groups at 9 days from baseline. (D) Comparison of MFS within and between groups at the time of intensive care unit (ICU) discharge (18.00 ± 10.19 days) from baseline; severity is proportional to high score. P-value and Cohen’s d effect size are noted from time group interaction at each determined time point.
Electrical stimulation therapy did not alter vital signs nor result in adverse events during the study period. The device did not interfere with the ongoing standard of care procedures for COVID-19 ICU patients, nor cause a burden to the ICU staff. Protocol delivery showed 14/16 patients (87.5%, n = 28 lower extremities) were able to complete E-Stim therapy during the established study period (9 days). At the first time point (3 days), the average of measured outcomes from independent lower-extremity voluntary metrics (Ankles) data excluding non-study-related adverse events (i.e., rapid deterioration = 6) was 100% (n = 26/26 samples), and the average for involuntary metrics (muscle endurance and SatO2) was 100% (32/32 samples). At the second time point (9 days), the average of measured outcomes from independent lower-extremity voluntary metrics (Ankles) data excluding non-study-related adverse events (i.e., intubation = 10, early discharge = 2, death = 2, deep vein thrombosis = 1) was 94.1% (16/17 samples), and the average for involuntary metrics (muscle endurance and SatO2) excluding non-study-related adverse events (i.e., intubated = 4, early discharge = 2, death = 2) was 91.6% (22/24 samples). Data for MFS functional assessment at the time of ICU discharge (18.0 ± 10.2 days, p = 0.81, d = 0.11) was collected in 100% (32/32 samples) of the participants.
This study examined the safety and efficacy of lower-extremity E-Stim adjunctive therapy to prevent muscle deconditioning. Our main goal was to determine whether this system can improve musculoskeletal outcomes at the earliest application from ICU admission. Results suggest that patients undergoing active E-Stim to the GNM had an improvement in Ankles and muscle endurance after 3 days compared to those that utilized sham devices. Comparison at 9 days showed there was significantly higher muscle endurance in patients undergoing active stimulation compared to those that did not. We believe these findings are due to the prompt activation of muscle fibers which may deaccelerate the rapid atrophy, myofilament damage, protein synthesis, and wasting found within the first week of ICU length of stay (43). E-Stim induces non-selective recruitment and activation of both type I and type II muscle fibers which conform the GNM (44), ultimately enhancing strength and cross-sectional area (45) in immobilized patients (46). Despite wide evidence which supports the feasibility and safety of E-Stim in the ICU setting (47, 48), further exploration is needed to confirm its efficacy at improving lower-extremity musculoskeletal outcomes, particularly in patients with severe hypoxia (i.e., ICU COVID-19).
Electrical stimulation is known to excite motor units that are used for greater levels of force production (49), aiding lower-extremity muscle strength preservation for voluntary activation (50). In a recent prospective cohort study in (n = 5) ICU COVID-19 patients, Righetti et al. stated daily E-Stim to the quadricep muscles in mechanical ventilated patients is feasible at improving strength at 5 and 8 days per interrupted sedation assessment (21). Similar non-COVID population studies utilizing E-Stim to the peroneus longus (n = 24) (51) and anterior tibialis (n = 11) (52) found a significant improvement in ankle dorsiflexion strength. Moreover, a randomized control trial (RCT) (53) delivering E-Stim to the GNM of ICU patients (n = 36) showed an improvement in strength at 9 days. The present RCT in ICU COVID-19 patients undergoing active E-Stim to the GNM showed an improvement in ankle dorsiflexion strength compared to controls with a medium effect size (p = 0.06, d = 0.77, Figure 4A) after 3 days of starting therapy. Unfortunately, results at 9 days were limited due to the high morbimortality status (i.e., deep vein thrombosis, intubation, death) impeding patients from performing voluntary tests, and limiting further assessment post-sedation.
2019 Novel Coronavirus reviews have also claimed E-Stim therapy may improve muscle endurance (18); however, evidence is supported by different types of immobilized populations (54). Hence, Veldman et al. suggested that E-Stim may result in a fast-to-slow muscle fiber type transition, which could potentially enhance endurance in patients with severe weakness unable to perform voluntary contractions (i.e., cardiorespiratory, critically illness) (55). However, due to the challenging objective assessment of muscle endurance in patients with severe illness, these outcomes were functionally assessed (i.e., waking distance movement) after recovery. In our study, we assessed real-time muscle endurance in the ICU setting utilizing electromyography (56). This test is optimal for assessment of muscle endurance and weakness as it offers a way to study the myoelectric features of neuromuscular activation associated with E-Stim (57). That said, the present study showed the IG had a higher improvement in GNMe with medium effect size at 3 days (p = 0.06, d = 0.69, Figure 4B) than the CG, yet a significant improvement with a large effect size at 9 days (p = 0.04, d = 0.97, Figure 4C) by increasing 6.3% (p = 0.029) from baseline (Table 2). This was especially noteworthy since all patients had null mobility over the first ∼3 days from ICU admission, avoiding any confounding effect of physical or nutritional therapy. This suggest that daily E-Stim may gradually improve GNMe in ICU COVID-19 patients.
Multiple studies (58) have suggested applying E-Stim therapy immediately after ICU admission to prevent lower-extremity neuromuscular damage in ICU patients (59–61). The physiology behind this suggestion relies on the fact that early introduction to E-Stim can ensure early activation/contraction of the motor unit (15). This is important because there is an abrupt decline in amplitude of nerve action potential and motor depolarization within 24 h from ICU admission (62). In ICU COVID-19 patients, there is an additional degenerative transformation and shrinkage of skeletal muscle due to sarcopenia, oxidative stress, and hyper-catabolism induced by cytokine storms and malnutrition (11, 63). In the present study, E-Stim was provided within ∼1.8 days from ICU admission; thus, an early involuntary contraction of motor units may have led the IG muscle outcomes to improve as early as 3 days. However, we believe therapy should be provided for prolonged periods, even after ICU discharge. Further studies are needed to explore musculoskeletal outcomes with the continuous use of E-Stim after hospital discharge.
Another consequence of immobile status, loss of voluntary contraction, and weakness acquired from the ICU is physical function impairment (64–66). A recent systematic review about post-ICU syndrome (67) reported significant functional disability due to lower-extremity problems during the first year following critical illness. The present study utilized the MFS (36) that evaluates ambulatory aid, gait, and transferring, among other functional aspects. Despite some patients losing consciousness during the study period (N = 6) and high-risk morbimortality, E-Stim was safely and continuously delivered in 87.5% (n = 28 lower extremities) of the cohort for a 9 days period without interfering with the responsibilities of the hospital staff. The short-time therapy effect was reflected in the significantly lower likelihood of falling in the IG compared to the CG (p = 0.05, Figure 4D) at the time of ICU discharge. Nonetheless, a longer follow-up period with larger sample sizes targeting functional objective measurements is warranted to assess limb functionality in critically ill COVID-19 patients undergoing E-Stim therapy.
Under E-Stim therapy, oxygen consumption of the lower extremity increases to supply energy to the lower-extremity muscles and thus maintain isometric muscle contraction (35, 68). At hypoxic levels, glycogen substitutes oxygen for energy supplementation via the anaerobic metabolism pathway (69) that, when depleted, may result in muscle fatigue and subsequent injury (70). Although no study has explored the effect of E-Stim on the tissue perfusion in the lower extremities of ICU COVID-19 patients (71), Gerovasili et al. examined it the thenar muscle of (n = 29) ICU patients. With a provoked vascular occlusion, they found that mean SatO2 assessed with Near Infra-red Spectroscopy (NIRS) did not differ before and after E-Stim therapy. With the severe hypoxia and blood oxyhemoglobin disassociation that critically ill COVID-19 patients present (72), one could expect this population may be more susceptible to muscle perfusion deterioration after undergoing stress (73). In the present RCT, the IG’s lower-extremity distal perfusion showed a similar pattern to the CG by remaining stable during the study period, meaning that daily E-Stim did not alter the level of muscle oxygen consumption in patients with severe hypoxia. However, studies evaluating SatO2 before and after 1 h E-Stim are needed to explore the real-time effects of COVID-19 in lower-extremity muscle perfusion.
We acknowledge the main limitation of this study is the small sample size, which may be underpowered for some of the outcomes. However, from the feasibility standpoint, we successfully collected all outcomes (i.e., Ankles, muscle endurance, and muscle perfusion), excluding those who underwent non-study-related adverse events, in 100% of the available samples at the 3 days time point, and 91.6% at the 9 days time point. Based on the observed effect sizes (d = 0.69–0.77, Table 2) at the 3 days time point, the available 26 samples (lower extremities) resulted in a generated power in range of 92–96% for Ankles, whereas for muscle endurance, the available 32 samples (lower extremities) resulted in a generated power in range of 97–99%. At the 9 days time point, the generated power for muscle endurance was greater than 80% (available samples = 22, d = 0.97, Table 2). However, the power was insufficient for Ankles (less than 80%) because of the reduced available samples (n = 15) in patients with deep vein thrombosis, intubation, or death due to COVID-19; thus, were not reported. This study was preventative; therefore, patient selection focused on those at high risk of muscle deconditioning but not clinically diagnosed with established guidelines for myopathy or neuropathy. COVID-19 variants were not reported. Creatinine phosphokinase, serum lactate, nor blood indicators for muscle damage were measured. SatO2 was not directly measured from the GNM. There were no other muscles stimulated or assessed. The duration of follow-up was short due to the high mortality rate in this particular population. Despite these limitations, the observed medium effects for benefit of E-Stim, ease of administration without overwhelming the nursing staff, and high acceptability encourage future studies to confirm the observed effects in preventing muscle deconditioning among clinically ill patients who require prolonged bed rest.
Our study supports the safety and efficacy of E-Stim in the ICU setting to prevent deterioration of lower-extremity muscle weakness in critically ill COVID-19 patients. This adjunctive therapy may provide a potential benefit for gastrocnemius muscle endurance and Ankles, thus, possibly aid on the prevention of functional sequelae in critically ill bedbound patients or those with similar characteristics of severe hypoxia or low SpO2. This is also true given the fact the benefit was observed in those intubated patients who continued to receive E-Stim therapy during their hospital length of stay. The benefits of E-Stim rely on the rapid involvement of therapy at the time of ICU admission. However, E-Stim does not replace PT, but rather enhances gastrocnemius muscle endurance and Ankles as an adjunctive treatment. Moreover, a portable and practical system that is easy to use does not interfere with the daily duties of the ICU staff. In addition, E-Stim did not alter vital signs or lower-extremity oxygen consumption, nor did it show adverse events during the study period. Further studies with larger sample sizes and longer follow-ups are warranted to examine the effectiveness of E-Stim to prevent muscle deconditioning in critically ill COVID-19 patients.
The data that support the findings of this study are not publicly available but are available from the corresponding author BN, bmFqYWZpLmJpamFuQGdtYWlsLmNvbQ== upon reasonable request.
The studies involving human participants were reviewed and approved by the Institutional Review Board for Human Subject Research for Baylor College of Medicine and Affiliated Hospitals (BCM IRB). The patients/participants provided their written informed consent to participate in this study. If the participant was cognitively impaired, consenting was performed via telephone call with a legal representative. The informed consent was obtained from all participants and/or their legal guardians.
BN, AZ-R, NR, MS, and JPH: concept and study design. AZ-R, NR, RM, RB, ML, and AB: acquisition, analysis, and interpretation of the data. AZ-R, RM, RB, ML, and AB: drafting the manuscript. BN, MS, and JPH: critical revision of the manuscript for important intellectual context. RM: statistical analysis. BN: obtained the funding. AZ-R, NR, and RB: administrative, technical, or material support. BN, MS, and JPH: supervision. All authors contributed to the article and approved the submitted version.
This study received funding from Avazzia Inc. (Dallas, TX, USA). The funder was not involved in the study design, collection, analysis, interpretation of data, and the writing of this article or the decision to submit it for publication.
We thank Michele Loor, Andrea Braun, and the ICU Staff from BSLMC for assisting with the data collection and coordination of this research study between involved key investigators.
The authors declare that the research was conducted in the absence of any commercial or financial relationships that could be construed as a potential conflict of interest.
All claims expressed in this article are solely those of the authors and do not necessarily represent those of their affiliated organizations, or those of the publisher, the editors and the reviewers. Any product that may be evaluated in this article, or claim that may be made by its manufacturer, is not guaranteed or endorsed by the publisher.
1. Brower RG. Consequences of bed rest. Crit Care Med. (2009) 37(Suppl. 10):S422–8. doi: 10.1097/CCM.0b013e3181b6e30a
2. Ferreira LL, Vanderlei LC, Valenti VE. Neuromuscular electrical stimulation in critically ill patients in the intensive care unit: a systematic review. Einstein (Sao Paulo Brazil). (2014) 12:361–5.
3. Shepherd S, Batra A, Lerner DP. Review of critical illness myopathy and neuropathy. Neurohospitalist. (2017) 7:41–8. doi: 10.1177/1941874416663279
4. McKendry J, Thomas ACQ, Phillips SM. Muscle mass loss in the older critically ill population: potential therapeutic strategies. Nutr Clin Pract. (2020) 35:607–16. doi: 10.1002/ncp.10540
5. Puthucheary ZA, Rawal J, McPhail M, Connolly B, Ratnayake G, Chan P, et al. Acute skeletal muscle wasting in critical illness. JAMA. (2013) 310:1591–600. doi: 10.1001/jama.2013.278481
6. Roberson AR, Starkweather A, Grossman C, Acevedo E, Salyer J. Influence of muscle strength on early mobility in critically ill adult patients: systematic literature review. Heart Lung. (2018) 47:1–9. doi: 10.1016/j.hrtlng.2017.10.003
7. Physiopedia,. COVID-19: Medium-to-Longer Term Health Considerations. (2020). Available online at: https://www.physio-pedia.com/index.php?title=COVID-19:_Medium-to-Longer_Term_Health_Considerations&oldid=254440 (accessed June 5, 2022).
8. Xiong TY, Redwood S, Prendergast B, Chen M. Coronaviruses and the cardiovascular system: acute and long-term implications. Eur Heart J. (2020) 41:1798–800. doi: 10.1093/eurheartj/ehaa231
9. Segredo V, Caldwell JE, Matthay MA, Sharma ML, Gruenke LD, Miller RD. Persistent paralysis in critically ill patients after long-term administration of vecuronium. N Engl J Med. (1992) 327:524–8. doi: 10.1056/NEJM199208203270804
10. Bonorino KC, Cani KC. Early mobilization in the time of COVID-19. Rev Bras Ter Intensiva. (2020) 32:484–6. doi: 10.5935/0103-507X.20200086
11. Ali AM, Kunugi H. Skeletal muscle damage in COVID-19: a call for action. Medicina (Kaunas). (2021) 57:372. doi: 10.3390/medicina57040372
12. Disser NP, De Micheli AJ, Schonk MM, Konnaris MA, Piacentini AN, Edon DL, et al. Musculoskeletal consequences of COVID-19. JBJSA. (2020) 102:11 97–204. doi: 10.2106/JBJS.20.00847
13. Piotrowicz K, Gąsowski J, Michel JP, Veronese N. Post-COVID-19 acute sarcopenia: physiopathology and management. Aging Clin Exp Res. (2021) 33:2887–98. doi: 10.1007/s40520-021-01942-8
14. Heesakkers H, van der Hoeven JG, Corsten S, Janssen I, Ewalds E, Simons KS, et al. Clinical outcomes among patients with 1 year survival following intensive care unit treatment for COVID-19. JAMA. (2022) 327:559–65. doi: 10.1001/jama.2022.0040
15. Larsson L, Degens H, Li M, Salviati L, Lee YI, Thompson W, et al. Sarcopenia: aging-related loss of muscle mass and function. Physiol Rev. (2019) 99:427–511. doi: 10.1152/physrev.00061.2017
16. Mishra R, Park C, York MK, Kunik ME, Wung SF, Naik AD, et al. Decrease in mobility during the COVID-19 pandemic and its association with increase in depression among older adults: a longitudinal remote mobility monitoring using a wearable sensor. Sensors (Basel). (2021) 21:3090. doi: 10.3390/s21093090
17. Hodgson CL, Tipping CJ. Physiotherapy management of intensive care unit-acquired weakness. J Physiother. (2017) 63:4–10. doi: 10.1016/j.jphys.2016.10.011
18. Nussbaum EL, Houghton P, Anthony J, Rennie S, Shay BL, Hoens AM. Neuromuscular electrical stimulation for treatment of muscle impairment: critical review and recommendations for clinical practice. Physiother Can. (2017) 69:1–76. doi: 10.3138/ptc.2015-88
19. Wageck B, Nunes GS, Silva FL, Damasceno MC, de Noronha M. Application and effects of neuromuscular electrical stimulation in critically ill patients: systematic review. Med Intensiva. (2014) 38:444–54. doi: 10.1016/j.medin.2013.12.003
20. Burgess LC, Venugopalan L, Badger J, Street T, Alon G, Jarvis JC, et al. Effect of neuromuscular electrical stimulation on the recovery of people with COVID-19 admitted to the intensive care unit: a narrative review. J Rehabil Med. (2021) 53:jrm00164. doi: 10.2340/16501977-2805
21. Righetti RF, Grams ST, Costa W, Saraiva LT, de Salles ICD, Yamaguti WP. Neuromuscular electrical stimulation in patients with severe COVID-19 associated with sepsis and septic shock. Front Med (Lausanne). (2022) 9:751636. doi: 10.3389/fmed.2022.751636
22. Minetto MA, Fior SD, Busso C, Caironi P, Massazza G, Maffiuletti NA, et al. Effects of neuromuscular electrical stimulation therapy on physical function in patients with COVID-19 associated pneumonia: study protocol of a randomized controlled trial. Contemp Clin Trials Commun. (2021) 21:100742. doi: 10.1016/j.conctc.2021.100742
23. Silva PE, Babault N, Mazullo JB, de Oliveira TP, Lemos BL, Carvalho VO, et al. Safety and feasibility of a neuromuscular electrical stimulation chronaxie-based protocol in critical ill patients: a prospective observational study. J Crit Care. (2017) 37:141–8. doi: 10.1016/j.jcrc.2016.09.012
24. Henzler D. Prone position technique. In: Vincent J-L, Hall JB editors. Encyclopedia of Intensive Care Medicine. Heidelberg: Springer Berlin Heidelberg (2012). p. 1851–4.
25. Senergy Medical Group, LLC. Tennant Biomodulator-Pro Owner’s Manual P/N 75019-0004K Manufactured by. Dallas, TX: Avazzia Inc (2017).
26. Zulbaran-Rojas, A, Park C, Lepow B, Najafi B. Effectiveness of lower-extremity electrical stimulation to improve skin perfusion. J Am Podiatr Med Assoc. (2021) 111. doi: 10.7547/20-172
27. Zulbaran-Rojas, A, Park C, El-Refaei N, Lepow B, Najafi B. Home-based electrical stimulation to accelerate wound healing-a double-blinded randomized control trial. J Diabetes Sci Technol. (2021):19322968211035128. doi: 10.1177/19322968211035128
28. Hermens HJ, Freriks B, Disselhorst-Klug C, Rau G. Development of recommendations for SEMG sensors and sensor placement procedures. J Electromyogr Kinesiol. (2000) 10:361–74. doi: 10.1016/S1050-6411(00)00027-4
29. Konard P. The ABC of EMG: A Practical Introduction to Kinesiological Electromyography. Scottsdale, AZ: Noraxon Inc (2005). p. 30–5.
30. Mishra RK, Maiti R. Non-linear signal processing techniques applied on EMG signal for muscle fatigue analysis during dynamic contraction. In: Mishra RK, Maiti R editors. CIRP Design 2012. London: Springer London (2013). p. 193–203. doi: 10.1007/978-1-4471-4507-3_19
32. Truong Quang Dang K, Le Minh H, Nguyen Thanh H, Vo Van T. Analyzing surface EMG signals to determine relationship between jaw imbalance and arm strength loss. Biomed Eng Online. (2012) 11:55. doi: 10.1186/1475-925X-11-55
33. Burns S, Biering-Sørensen F, Donovan W, Graves DE, Jha A, Johansen M, et al. International standards for neurological classification of spinal cord injury, revised 2011. Topics Spinal Cord Injury Rehabil. (2012) 18:85–99. doi: 10.1310/sci1801-85
34. Hagberg M. Muscular endurance and surface electromyogram in isometric and dynamic exercise. J Appl Physiol Respir Environ Exer Physiol. (1981) 51:1–7. doi: 10.1152/jappl.1981.51.1.1
35. Banerjee P, Clark A, Witte K, Crowe L, Caulfield B. Electrical stimulation of unloaded muscles causes cardiovascular exercise by increasing oxygen demand. Eur J Cardiovasc Prev Rehabil. (2005) 12:503–8. doi: 10.1097/01.hjr.0000169188.84184.23
36. Morse JM, Morse RM, Tylko SJ. Development of a scale to identify the fall-prone patient. Can J Aging. (2010) 8:366–77. doi: 10.1017/S0714980800008576
37. Kho ME, Molloy AJ, Clarke FJ, Reid JC, Herridge MS, Karachi T, et al. Multicentre pilot randomised clinical trial of early in-bed cycle ergometry with ventilated patients. BMJ Open Respir Res. (2019) 6:e000383. doi: 10.1136/bmjresp-2018-000383
38. Windholz T, Swanson T, Vanderbyl BL, Jagoe RT. The feasibility and acceptability of neuromuscular electrical stimulation to improve exercise performance in patients with advanced cancer: a pilot study. BMC Palliat Care. (2014) 13:23. doi: 10.1186/1472-684X-13-23
39. Najafi B, Talal TK, Grewal GS, Menzies R, Armstrong DG, Lavery LA. Using plantar electrical stimulation to improve postural balance and plantar sensation among patients with diabetic peripheral neuropathy: a randomized double blinded study. J Diabetes Sci Technol. (2017) 11:693–701. doi: 10.1177/1932296817695338
40. Kang H. Sample size determination and power analysis using the G*Power software. J Educ Eval Health Prof. (2021) 18:17. doi: 10.3352/jeehp.2021.18.17
41. Khan SZ, Awn Bin Z, Waris N, Miyan Z, Ulhaque MS, Fawwad A. Comparison of ankle-brachial index (ABI) measured by an automated oscillometric apparatus with that by standard hand-held doppler in patients with type-2 diabetes. Pak J Med Sci. (2019) 35:1167–72. doi: 10.12669/pjms.35.4.30
42. Häkkinen K, Kraemer WJ, Newton RU. Muscle activation and force production during bilateral and unilateral concentric and isometric contractions of the knee extensors in men and women at different ages. Electromyogr Clin Neurophysiol. (1997) 37:131–42.
43. Balke M, Teschler M, Schäfer H, Pape P, Mooren FC, Schmitz B. Therapeutic potential of electromyostimulation (EMS) in critically ill patients-a systematic review. Front Physiol. (2022) 13:865437. doi: 10.3389/fphys.2022.865437
44. Gregory CM, Bickel CS. Recruitment patterns in human skeletal muscle during electrical stimulation. Phys Ther. (2005) 85:358–64. doi: 10.1093/ptj/85.4.358
45. Gondin J, Guette M, Ballay Y, Martin A. Neural and muscular changes to detraining after electrostimulation training. Eur J Appl Physiol. (2006) 97:165–73. doi: 10.1007/s00421-006-0159-z
46. Paillard T, Noé F, Passelergue P, Dupui P. Electrical stimulation superimposed onto voluntary muscular contraction. Sports Med. (2005) 35:951–66. doi: 10.2165/00007256-200535110-00003
47. Edwards J, McWilliams D, Thomas M, Shah S. Electrical muscle stimulation in the intensive care unit: an integrative review. J Intensive Care Soc. (2014) 15:142–9. doi: 10.1177/175114371401500212
48. Zayed Y, Kheiri B, Barbarawi M, Chahine A, Rashdan L, Chintalapati S, et al. Effects of neuromuscular electrical stimulation in critically ill patients: a systematic review and meta-analysis of randomised controlled trials. Aust Crit Care. (2020) 33:203–10. doi: 10.1016/j.aucc.2019.04.003
49. Enoka RM, Amiridis IG, Duchateau J. Electrical stimulation of muscle: electrophysiology and rehabilitation. Physiology (Bethesda). (2020) 35:40–56. doi: 10.1152/physiol.00015.2019
50. Hollis S, McClure P. Intramuscular electrical stimulation for muscle activation of the tibialis anterior after surgical repair: a case report. J Orthop Sports Phys Ther. (2017) 47:965–9. doi: 10.2519/jospt.2017.7368
51. Karatzanos E, Gerovasili V, Zervakis D, Tripodaki ES, Apostolou K, Vasileiadis I, et al. Electrical muscle stimulation: an effective form of exercise and early mobilization to preserve muscle strength in critically ill patients. Crit Care Res Pract. (2012) 2012:432752. doi: 10.1155/2012/432752
52. Falavigna LF, Silva MG, Freitas AL, Silva PF, Paiva Júnior MD, de Castro CM, et al. Effects of electrical muscle stimulation early in the quadriceps and tibialis anterior muscle of critically ill patients. Physiother Theory Pract. (2014) 30:223–8. doi: 10.3109/09593985.2013.869773
53. Kho ME, Truong AD, Zanni JM, Ciesla ND, Brower RG, Palmer JB, et al. Neuromuscular electrical stimulation in mechanically ventilated patients: a randomized, sham-controlled pilot trial with blinded outcome assessment. J Crit Care. (2015) 30:32–9. doi: 10.1016/j.jcrc.2014.09.014
54. Erickson ML, Ryan TE, Backus D, McCully KK. Endurance neuromuscular electrical stimulation training improves skeletal muscle oxidative capacity in individuals with motor-complete spinal cord injury. Muscle Nerve. (2017) 55:669–75. doi: 10.1002/mus.25393
55. Veldman MP, Gondin J, Place N, Maffiuletti NA. Effects of neuromuscular electrical stimulation training on endurance performance. Front Physiol. (2016) 7:544. doi: 10.3389/fphys.2016.00544
56. Plaut T, Weiss L. Electrodiagnostic Evaluation Of Critical Illness Neuropathy. StatPearls. Treasure Island, FL: StatPearls Publishing (2022).
57. Hayashibe M. Evoked electromyographically controlled electrical stimulation. Front Neurosci. (2016) 10:335. doi: 10.3389/fnins.2016.00335
58. Baron MV, Pinto MVDM, Koepp J, Brandenburg C, Martins PR, dos Santos AC, et al. Neuromuscular electrical stimulation in intensive care unit patients: integrative review. Modern Res Inflamm. (2019) 8:11–27. doi: 10.4236/mri.2019.82002
59. Burke D, Gorman E, Stokes D, Lennon O. An evaluation of neuromuscular electrical stimulation in critical care using the ICF framework: a systematic review and meta-analysis. Clin Respir J. (2016) 10:407–20. doi: 10.1111/crj.12234
60. Maggioni MA, Cè E, Rampichini S, Ferrario M, Giordano G, Veicsteinas A, et al. Electrical stimulation versus kinesitherapy in improving functional fitness in older women: a randomized controlled trial. Arch Gerontol Geriatr. (2010) 50:e19–25. doi: 10.1016/j.archger.2009.04.015
61. Mignardot JB, Deschamps T, Le Goff CG, Roumier FX, Duclay J, Martin A, et al. Neuromuscular electrical stimulation leads to physiological gains enhancing postural balance in the pre-frail elderly. Physiol Rep. (2015) 3:e12471. doi: 10.14814/phy2.12471
62. Latronico N, Fagoni N, Gobbo M. Chapter 46 - Neuromuscular electrical stimulation in critically ill patients. In: Prabhakar H editor. Essentials of Neuroanesthesia. Cambridge, MA: Academic Press (2017). p. 771–81. doi: 10.1016/B978-0-12-805299-0.00046-4
63. Welch C, Greig C, Masud T, Wilson D, Jackson TA. COVID-19 and acute sarcopenia. Aging Dis. (2020) 11:1345–51. doi: 10.14336/AD.2020.1014
64. Rudrappa SS, Wilkinson DJ, Greenhaff PL, Smith K, Idris I, Atherton PJ. Human skeletal muscle disuse atrophy: effects on muscle protein synthesis, breakdown, and insulin resistance-a qualitative review. Front Physiol. (2016) 7:361. doi: 10.3389/fphys.2016.00361
65. Herridge MS, Cheung AM, Tansey CM, Matte-Martyn A, Diaz-Granados N, Al-Saidi F, et al. 1 year outcomes in survivors of the acute respiratory distress syndrome. N Engl J Med. (2003) 348:683–93. doi: 10.1056/NEJMoa022450
66. Herridge MS, Tansey CM, Matté A, Tomlinson G, Diaz-Granados N, Cooper A, et al. Functional disability 5 years after acute respiratory distress syndrome. N Engl J Med. (2011) 364:1293–304. doi: 10.1056/NEJMoa1011802
67. Ohtake PJ, Lee AC, Scott JC, Hinman RS, Ali NA, Hinkson CR, et al. Physical impairments associated with post-intensive care syndrome: systematic review based on the World Health Organization’s international classification of functioning, disability and health framework. Phys Ther. (2018) 98:631–45. doi: 10.1093/ptj/pzy059
68. Black S, Carter GM, Nitz AJ, Worthington JA. Oxygen consumption for lower extremity exercises in normal subjects and burned patients. Phys Ther. (1980) 60:1255–8. doi: 10.1093/ptj/60.10.1255
69. A skeletal muscle protein that regulates endurance. PLoS Biol. (2004) 2:e315. doi: 10.1371/journal.pbio.0020315
70. Dugan SA, Frontera WR. Muscle fatigue and muscle injury. Phys Med Rehabil Clin North Am. (2000) 11:385–403. doi: 10.1016/S1047-9651(18)30135-9
71. Gerovasili V, Tripodaki E, Karatzanos E, Pitsolis T, Markaki V, Zervakis D, et al. Short-term systemic effect of electrical muscle stimulation in critically ill patients. Chest. (2009) 136:1249–56. doi: 10.1378/chest.08-2888
72. Núñez-Cortés R, Rivera-Lillo G, Arias-Campoverde M, Soto-García D, García-Palomera R, Torres-Castro R. Use of sit-to-stand test to assess the physical capacity and exertional desaturation in patients post COVID-19. Chron Respir Dis. (2021) 18:1479973121999205. doi: 10.1177/1479973121999205
Keywords: COVID-19, critically ill patients, lower extremity weakness, electrical stimulation, intensive care unit
Citation: Zulbaran-Rojas A, Mishra R, Rodriguez N, Bara RO, Lee M, Bagheri AB, Herlihy JP, Siddique M and Najafi B (2022) Safety and efficacy of electrical stimulation for lower-extremity muscle weakness in intensive care unit 2019 Novel Coronavirus patients: A phase I double-blinded randomized controlled trial. Front. Med. 9:1017371. doi: 10.3389/fmed.2022.1017371
Received: 11 August 2022; Accepted: 17 November 2022;
Published: 06 December 2022.
Edited by:
Giuliana Scarpati, University of Salerno, ItalyReviewed by:
Felipe González-Seguel, Universidad del Desarrollo, ChileCopyright © 2022 Zulbaran-Rojas, Mishra, Rodriguez, Bara, Lee, Bagheri, Herlihy, Siddique and Najafi. This is an open-access article distributed under the terms of the Creative Commons Attribution License (CC BY). The use, distribution or reproduction in other forums is permitted, provided the original author(s) and the copyright owner(s) are credited and that the original publication in this journal is cited, in accordance with accepted academic practice. No use, distribution or reproduction is permitted which does not comply with these terms.
*Correspondence: Bijan Najafi, YmlqYW4ubmFqYWZpQGJjbS5lZHU=
†These authors have contributed equally to this work and share first authorship
Disclaimer: All claims expressed in this article are solely those of the authors and do not necessarily represent those of their affiliated organizations, or those of the publisher, the editors and the reviewers. Any product that may be evaluated in this article or claim that may be made by its manufacturer is not guaranteed or endorsed by the publisher.
Research integrity at Frontiers
Learn more about the work of our research integrity team to safeguard the quality of each article we publish.