- 1Department of Small Animal Medicine & Surgery, University of Veterinary Medicine Hannover, Hanover, Germany
- 2Dubai Police K9, Dubai, United Arab Emirates
- 3International Operations Department, Ministry of Interior of the United Arab Emirates, Abu Dhabi, United Arab Emirates
- 4Faculty of Veterinary Science, University of Buenos Aires, Buenos Aires, Argentina
- 5Department of Veterinary Medicine, Federal Rural University of Pernambuco, Recife, Brazil
- 6Center for Microbial Ecology and Technology, Department of Biotechnology, Ghent University, Ghent, Belgium
- 7School of Animal and Veterinary Sciences, The University of Adelaide, Roseworthy, SA, Australia
- 8École Nationale Vétérinaire d’Alfort, IMRB, Université Paris Est, Maisons-Alfort, France
- 9Kynoscience UG, Hörstel, Germany
- 10École Nationale Vétérinaire d’Alfort, Université Paris-Est, Maisons-Alfort, France
- 11Medical Detection Dogs, Milton Keynes, United Kingdom
- 12Clinical Department of Production Animals, Fundamental and Applied Research for Animals & Health Research Unit, Faculty of Veterinary Medicine, University of Liège, Liège, Belgium
- 13Department of Equine and Small Animal Medicine, University of Helsinki, Helsinki, Finland
- 14Department of Biometry, Epidemiology and Information Processing, University of Veterinary Medicine Hannover, Hanover, Germany
- 15Department of Disease Control, London School of Hygiene and Tropical Medicine, London, United Kingdom
- 16Arctech Innovation, The Cube, Dagenham, United Kingdom
- 17Laboratory OBI K19 Biodetection Center, Hermosillo, Mexico
- 18Escuela de Medicina Veterinaria, Facultad de Agronomía e Ingeniería Forestal and Facultad de Ciencias Biológicas y Facultad de Medicina, Pontificia Universidad Católica de Chile, Santiago, Chile
- 19Center for Human Genetics, College of Medicine and Health Sciences, University of Rwanda, Kigali, Rwanda
- 20Rwanda National Joint Task Force COVID-19, Kigali, Rwanda
- 21University Teaching Hospital of Butare, Butare, Rwanda
- 22Penn Vet Working Dog Center, Department of Clinical Sciences and Advanced Medicine, School of Veterinary Medicine, University of Pennsylvania, Philadelphia, PA, United States
- 23Department of Animal Morphology and Physiology, Federal Rural University of Pernambuco, Recife, Brazil
- 24Bundeswehr Medical Service Headquarters, Koblenz, Germany
- 25Laboratorio de Parasitología y Patología Acuática, Departamento de Recursos del Mar, Centro de Investigación y de Estudios Avanzados del IPN Unidad Mérida, Mérida, Yucatán, Mexico
- 26Center for Systems Neuroscience Hannover, Hanover, Germany
Introduction
The respiratory coronavirus disease 2019 (COVID-19) caused by the severe acute respiratory syndrome coronavirus type 2 (SARS-CoV-2) quickly developed into a pandemic (1). Even though laboratory diagnostic tests and vaccines were consequently developed (2, 3), the exploration of rapidly deployable, more reliable tools for addressing the current and future pandemics was vital. Toward this goal, researchers worldwide evaluated the use of medical detection dogs as a rapid, reliable and cost-effective screening method for SARS-CoV-2 infections (4). The ability of dogs to distinguish diseases by their high-resolution sense of smell is based on the volatile organic compound (VOC)-hypothesis (5). Numerous infectious and non-infectious diseases change metabolic processes releasing characteristic VOC-patterns in the form of an “olfactory fingerprint” (6–10). Many studies have shown that dogs can detect metabolic disorders, such as cancer (11) and hypoglycemia (12), predict epileptic seizures (13, 14), or even distinguish various pathogens (8, 15–17). Approximately 78% of the 27 SARS-CoV-2-canine detection studies reviewed by Meller et al. yielded > 80% sensitivity and approximately 60% of studies yielded > 95% of specificity (4), highlighting the potential of the dog as a “diagnostic system” and its recommendation for certain settings. Despite these promising results, all studies published up to now differed in numerous design features. They were mostly designed as pilot studies and case-control selection of patients was mostly favored over a more preferable cross-sectional (“cohort”) selection [study quality assessment was conducted and presented by Meller et al. (4)]. The aim of this comprehensive review summary is to provide a general overview of the divergent aspects that may impact canine disease detection and to provide recommendations for future deployment of medical detection dogs (see also summary in Table 1). Specific emphasis is placed on the choice of dogs, training paradigms, safety aspects, sample characteristics, pre-screen processing (e.g., inactivation), and screening-population and its environment related aspects, respectively (see also Figure 1 and Supplementary Figure 1), providing an outlook and proposals for the future standardization in the use of dogs for disease detection. Ultimately, this report provides a blueprint for the potential use of medical detection dogs in future epidemics and pandemics.
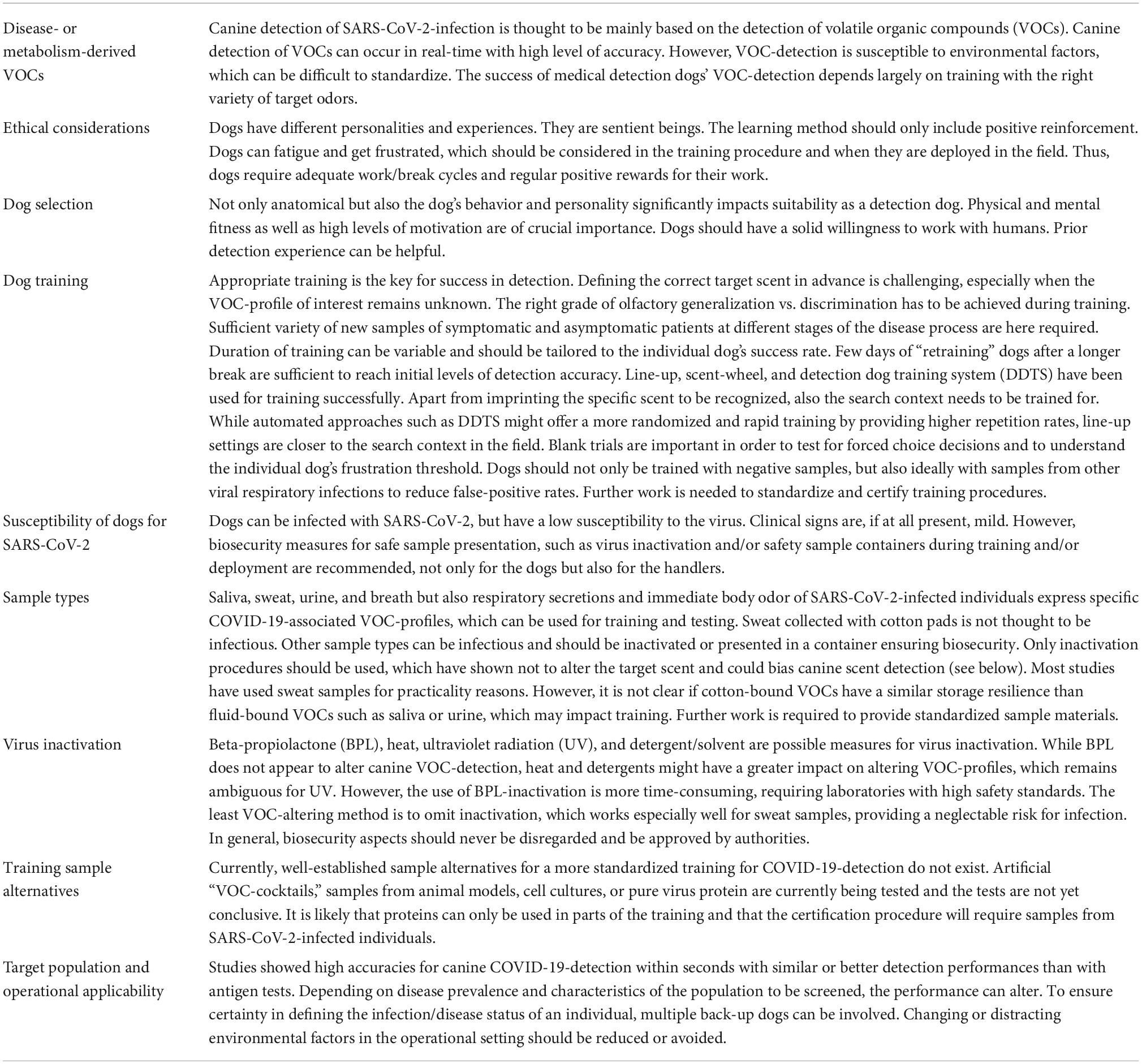
Table 1. Summarizing comments and recommendations for medical canine scent detection of samples from SARS-CoV-2-infected individuals.
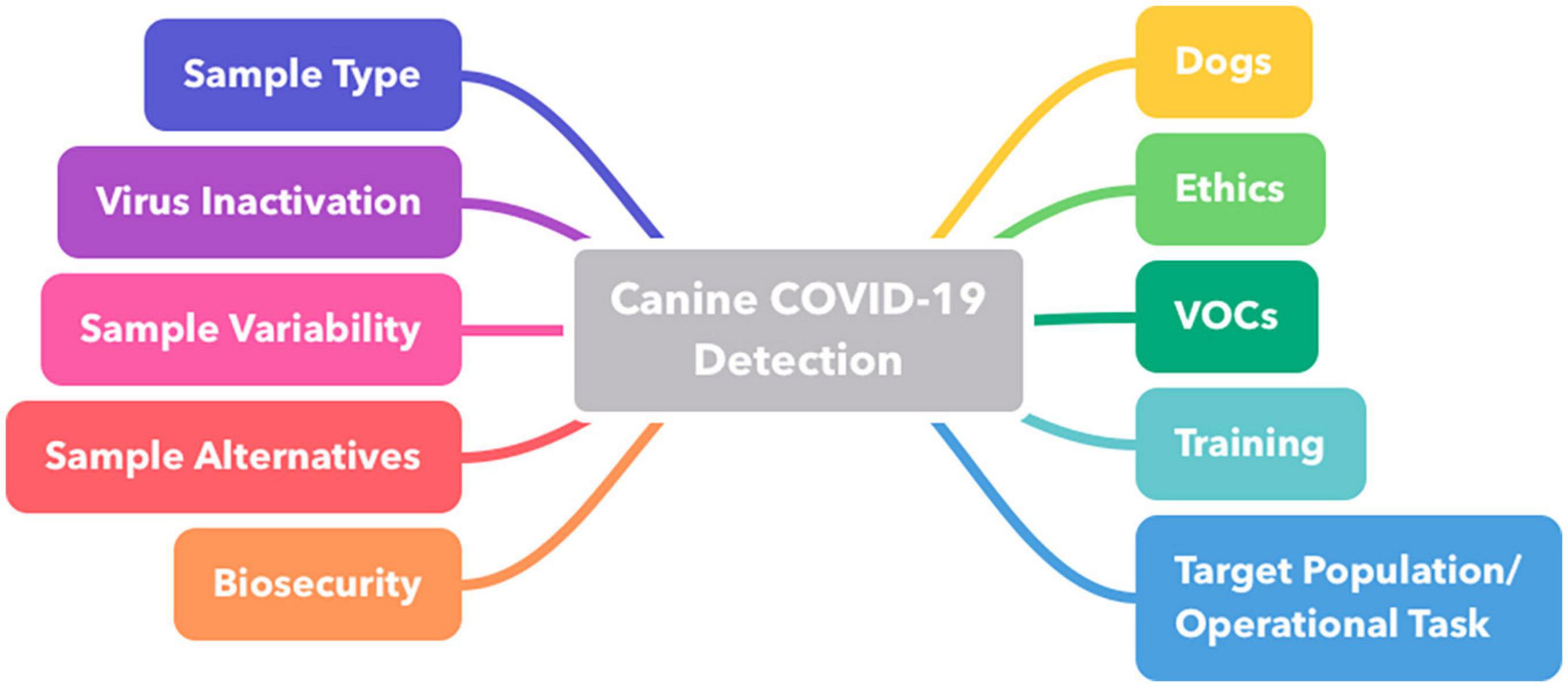
Figure 1. Mind map representing key areas of interest highlighted and discussed by the group of experts. VOC, volatile organic compound.
Disease- or metabolism-derived volatile organic compounds
Infectious and non-infectious diseases can produce metabolic alterations that may be associated with the release of volatile organic compounds (VOCs) from the body (6–10). In this way, specific volatile biochemical fingerprints may be detected and function as biomarkers for corresponding diseases and their clinical course, provided that appropriate sensory means are available (18, 19). The detective olfactory potential of dogs and other animals has been researched in the medical field concerning various infectious viral, bacterial, and parasitic as well as non-infectious diseases and disorders like epilepsy, diabetes, and cancer (5, 11, 20, 21). Horvath et al. demonstrated that dogs can differentiate between normal and neoplastic tissue as well as non-neoplastic disease processes such as inflammation, necrosis or emergence of metabolic products (22). For example, Ehmann et al. reported that detection dogs were able to differentiate lung cancers from chronic obstructive pulmonary disease (COPD) by sniffing the breath (23). The occurrence of specific disease-associated VOC-profiles using chemical analytical methods and technical sensory devices was shown in ovarian (24) and breast cancer (25) or in various respiratory diseases (26) and other infections (27). By applying quantitative analytical methods in animal or in vitro models, interesting questions about the temporal and quantitative dynamics of VOC-production across infection states and progress can be addressed. Traxler et al. (28) detected VOC-changes in the breath of pigs after influenza A infection versus control animals. Interestingly, none of the animals in the study displayed clinical signs, indicating that changes in VOCs still remain despite a lack of significant host immune responses (28). Another study measured VOCs produced by B lymphoblastoid cells following infection with specific avian and human influenza strains in vitro. VOCs did change depending on infection status, which coincided with the many cellular processes that occur when an organism becomes infected (29).
Gould et al. summarized that, in various viral infections, glycolysis in host cells is elevated due to the necessary energy supply for replication, accompanied with increased production of fatty acids, alkanes and related products (30). SARS-CoV-2-infections were shown to lead to characteristic immune and metabolic dysregulation in proteins and lipids in blood serum (31). SARS-CoV-2-specific biochemical processes, such as those associated with modes of entry and replication in cells, combined with induction of humoral and cellular immunologic reactions as well as the dynamic cytokine release might play an important role in COVID-19-specific VOC-expression (32).
The smell of COVID-19
Various studies exist, which give striking insights into SARS-CoV-2-VOC-profiles with differing identifiable VOCs mainly via gas chromatography-mass spectrometry (GC-MS), gas chromatography-ion mobility spectrometry (GC-IMS), time-of-flight-mass spectrometry (TOF-MS) or related techniques (33–38). In principle, spectrometric techniques enable the identification and quantification of VOCs in breath samples, preceded by gas chromatographic separation if needed. Prior studies have reported quantifiable differences in about two dozen VOCs between individuals with COVID-19 versus healthy individuals as well as individuals with other respiratory diseases. Particularly striking here are COVID-19-associated elevated concentrations of certain alcohols such as butanol and propanol or derivatives (33, 35, 37, 38), aldehydes such as heptanal, octanal, and nonanal (33, 34, 36), as well as ketones such as acetone and butanone or derivatives (33, 38). Other substances with reported increased concentrations are various alkanes, alkenes, further aldehydes, aromatic substances, and their derivatives (33, 34, 36–38). Decreased VOC-concentrations in COVID-19-breath were shown for methanol (33) and – in contrast to Ruszkiewicz et al. (33) – acetone (35). In addition, Feuerherd et al. showed by headspace air sampling of virus-infected cell cultures that specific differences in 2-butanone, nonane, and pentanal concentrations represent robust discriminatory features between SARS-CoV- 2-, human coronavirus NL63-, and influenza A virus subtype H1N1-infections (39). Similarly, Steppert et al. were able to discriminate between individuals infected with influenza A virus or SARS-CoV-2 analyzing breath samples via IMS coupled with a multicapillary column (40). In a study from ten Hagen et al. dogs were able to discriminate supernatants of SARS-CoV-2-infected human cell cultures from 15 other viruses including coronaviridae, orthomyxoviridae, paramyxoviridae, pneumoviridae, adenovirus, and rhinovirus among others (41).
The use of electronic noses (eNoses) has also been explored by some studies for the detection of COVID-19. Sensors and nanotechnology allow to detect differences in the chemical composition of air samples by means of chemical reactions with sensor arrays consisting of specific coatings of certain metal oxides, organic polymers, nanoparticles, etc. (42, 43). The emerging differences in resistance and conductivity produce corresponding “volatile finger-” or “breathprints” via artificial neural networks (44). eNoses were able to discriminate breath samples between individuals with symptomatic COVID-19 versus healthy individuals (45–47) or other respiratory diseases (48), Post-COVID-19 condition (49), and non-symptomatic COVID-19 (47, 50). Two recent studies provided evidence that also dogs can detect Post-COVID-19 conditions (51, 52).
Detection of disease-related volatile organic compounds by devices versus dogs
Despite good discriminatory potential within individual studies, the comparison of the described chemical analytical or sensor methods between studies nevertheless highlights some drawbacks of these techniques, which may create challenges for their use in an open screening process. In the following paragraphs, certain features of the canine and technical methods are critically discussed.
First, it is not ensured that all relevant VOCs are reliably detected via MS or sensor methods. Differences in databases and small number of metabolites available as standards complicate interpretations of MS analyses (53). Small ions, molecules or molecular fragments cannot be easily detected and make it difficult to interpret and draw conclusions about originally contained compounds. For example, small hydrocarbon-based molecules occur abundantly in exhaled breath, making their detection complicated due to overlap with molecules of similar spectra (38). In addition, certain measurable VOCs are non-specifically altered across diseases making disease discrimination prone to errors. For example, elevated propanol in breath is associated with infectious and non-infectious respiratory diseases other than COVID-19 (35, 54–57). Analogously, a certain “roughness” of detection is also given with eNoses, since the selective and susceptible coatings of the sensors might lead to physical limitations in qualitative and quantitative resolution (42, 58, 59). These aspects become impactful, especially when considering that VOCs in exhaled breath are numerous and most of the VOC-compositions have wide inter-individual variations (60). Similarly, some uncertainties exist in canine detection, as well, since research in perception and processing of certain olfactory cues in dogs is not yet very advanced. Thus, the definition of the target odor, especially in the medical field, remains one of the main challenges in canine scent detection.
Second, differences in the detection of COVID-19-VOCs across studies with MS-detection might emerge due to the choice of different detection and analytical techniques, different patient recruitment procedures and the environment (33, 37). Snitz et al. and Rodriguez-Aguilar et al., who conducted cross-sectional trials in a real-life scenario with eNoses, showed the significant impact of differing sample acquisition methods and environmental factors on the results (45, 50). Although disease discrimination was possible, certain environment-associated deterioration in eNose performance could not be excluded (50). Therefore, it is probable that the chemical analytical and sensor detection methods are susceptible to “olfactory noise” for COVID-19-detection. While these devices might feed intrinsic and extrinsic VOCs to the analyzer in an unfiltered, noisy, and “one-dimensional” manner, living biosensors such as dogs may perceive the learned sensations that are evoked due to a certain key composition, or “network,” of complex and low-concentration VOCs. Dogs are therefore possibly more capable of searching specifically for the “needle in the haystack” than current technical solutions, provided that training samples are correctly and meticulously defined according to the target condition. However, olfactory noise and other distractors may play an important role for canine detection, as well, especially when using detection dogs in the open environmental space. Further research is needed to increase control of these confounding factors.
Third, chemical analytical instruments are often stationary devices. They are mainly used offline and are coupled with software for evaluative steps. eNoses are mobile and online analysis is possible, but they require further software and deep-learning approaches in order to “learn” and analyze specific VOC-patterns. For example, the sensors must be able to detect the correct compounds and in the correct ratio and at low concentrations. The software then has to interpret the signals correctly, and environmental factors can cause difficulties, as described above. Each specific application needs considerable method development work in advance and is cost-intensive which is a drawback in rapid pandemic dynamics of emerging pathogens. Marder et al. stated that “data processing is a major bottleneck of metabolomics” (38). Furthermore, sensors often have a short life and their sensitivity deteriorates in presence of humidity (42, 48, 50). The analysis time for chemical analytical devices or sensors used for COVID-19-detection in the aforementioned studies (see section “The smell of COVID-19”) revealed a range of one to 16 min per sample. Dogs, on the other hand, are mobile and can identify COVID-19-samples within a few seconds, i.e., in real-time. This requires preceding specific canine training for high discriminating performance of approximately 4 weeks with a range of 2–15 weeks regardless of the chosen training method (when studies with dogs that had previous COVID-19-scent experience were excluded) (4). However, a variety of factors can have a large influence on learning efficiency, e.g., number of sample exposures, environmental factors, the success of odor generalization, etc. Furthermore, personality traits of dogs and emerging fatigue during work (see also section “Considerations regarding Dog Selection”) are impacting factors, which represent a disadvantage compared to well established artificial devices.
Finally, the lower limit of detection in dogs is one part per trillion (ppt), exceeding the range of detection of current available instruments by around three orders of magnitude (61–63). A new study shows that dogs are indeed able to detect even far lower concentrations, in the order of 10–21 (Turunen et al., unpublished). Since it was reported that VOCs from breath are released in the range of parts per billion (ppb) to ppt, dogs might appear more suitable for VOC-detection in comparison to instruments with sensitivities in the ppb range (50, 64). However, the canine range of detection was validated in controlled environments, which could mask an actual lower sensitivity. In addition, sensitivity might also depend on the qualitative characteristics of the target odor.
In hospitals and other health care facilities, chemical analytical and sensory instruments are well suited for sensitive and relatively rapid isolation of patients (33, 37), provided that they are swiftly fed with sufficient data for rapid adaptive purposes (36, 65). For external mass screening, the use of such technical devices for VOC-detection is complex due to sample processing time, limited selectivity, and increased susceptibility to material damage as well as to external olfactory noise in a poorly controllable environment. Although similar challenges may exist for dogs, their ability to learn and to process information immediately can make them more capable of searching for specific odors in real time, particularly in complex environments. However, the success of canine detection depends significantly on the training methods and the choice of the right training samples, which is one of the main challenges and disadvantages compared to established analytical and sensory methods. Finally, dogs are likely to be complimentary to sensors and analytical methods and more appropriate for certain scenarios.
Ethical considerations for using detection dogs
One important consideration in repurposing dogs’ olfactory abilities for the detection of specific odors is that dogs are living beings with different and individual needs, characters, experiences, behaviors, and capabilities (66). In addition, these elements may differ in the same individual over time due to intrinsic and extrinsic factors. These characteristics, which from an ethical point of view must be protected and respected, considerably distinguish dogs from standardized, industrially produced test kits that have been tailored to a specific purpose (67). Ethical considerations in using dogs’ abilities for human purposes are therefore paramount. The method of operant conditioning including positive reinforcement of correct searching behavior by reward (food, toy, etc.) and absence of reward for undesired searching behavior, is considered as ethically unobjectionable, and was the method used across the canine SARS-CoV-2-detection literature (4). For the dogs, the method forms a motivation and pleasure driven detection exercise using olfaction as one of the most important sensory and cognitive tools in macrosmatics. On the other hand, one is confronted with potential short-term issues such as fatigue and/or boredom after a certain time of action, highlighting the importance of specific and individual adaptations in training and deployment according to the different personality traits of the dogs (67). However, Guest et al. drawing on their experience of deploying medical scent detection dogs, suggest that two trained dogs would have the potential to screen 300 individuals in 30 min in a COVID-19-screening scenario (47), which exceeds the capacity of current available testing methods by far. After the fast screening of a population by canine detection, reference standard reverse transcription quantitative real-time polymerase chain reaction (RT-qPCR) of positive scent detected individuals can be applied as further downstream verification (47). Such approaches were already being pursued early in the pandemic, e.g., at Dubai Airport in July 2020.
Importantly, when dogs are deployed in the field and the disease of interest has a low prevalence, reducing the opportunity for the animals to succeed in detection, positive affective and motivational states of dogs have to be sustained in order to avoid frustration (68). This can be achieved, for example, through regular rewards for the respective detection procedure or for detecting specifically prepared (positive) samples (69–71). Interestingly, variation in reward types may lead to a more pronounced maintenance of motivation in some dogs (72). In addition, adequate work/rest cycles for the dogs are of significant relevance for animal welfare and for high efficiency in scent detection work (73, 74).
Considerations regarding dog selection
Besides a well-functioning and harmonic partnership between dog and its handler, many other individual factors can ultimately influence the effectiveness of olfactory detection. These are highlighted in the following. Three recent reviews provide a more detailed overview about the anatomy, physiology, and other factors related to canine olfaction performance (75–77).
Intrinsic factors
Breed-specific anatomy and physiology
The paucity of comparative studies on the olfactory abilities of different dog breeds including intra-breed variations represents a challenge for the selection of suitable detection dogs (75, 76). Although it can be hypothesized that anatomical and physiological characteristics of the olfactory organ play a crucial role, behavioristic and mental aspects, personal traits, and experiences are of no less importance for adequate canine screening work (76).
The mechanisms involved in molecular recognition in olfactory receptors (OR) and olfactory sensory neurons and in the identification of specific odorants are still only partially understood. In this regard, the current consensus is that each OR has a characteristic ligand spectrum and each odorant can also be detected by a combination of ORs (78). Gene polymorphisms in expression of ORs in the same breed but also between breeds differ and may be used as an indicator for scent discrimination performance (79–83). In addition, the total number of neurons, i.e., the size of the olfactory epithelium, may have an effect on olfactory acuity in dogs (79), which might be due to enhanced olfactory resolution with increased numbers of neurons (84). One study showed that dolichocephalic or normocephalic dogs (often classified as scent breeds) and wolves have better olfactory capabilities than non-scent breeds and brachycephalic dogs (85). Brachycephalic breeds have less space for the olfactory epithelium to expand in the nasal cavity and less olfactory cells reducing olfactory sensitivity, and pronounced breathing issues leading to reduced cerebral oxygen supply, reduced heat elimination, and therefore to quicker fatigue (84, 86, 87). Thickened conchae and less ramifications inside the nasal cavity may be a reason for less epithelial surface (88). Brachycephalic breeds should therefore be avoided for scent detection tasks (76). Controversially and surprisingly, Hall et al. showed that pugs are able to outperform German shepherds in olfactory tasks (89), highlighting that behavioral aspects play a crucial role as well.
Furthermore, olfactory airflow in dogs in ethmoidal regions is laminar which is optimized for scent molecule transport (62, 90). This type of airflow is impacted in brachycephalic breeds due to an obstructive and deforming development of the nasal cavity and nasal conchae (87, 88), especially since the dorsal meatus in the canine nasal cavity, functioning as a bypass for olfactory laminar air supply, is only ventilated when high inspiratory pressure is applied (62, 90, 91). However, Wagner and Ruf showed that a large surface of the bony turbinates in dolichocephalic dogs is not the main reason for a better smelling ability (92), highlighting, again, the fact that breed and anatomy should not be the ultimate reason for defining a good detection dog (76).
All mentioned dog breeds in the reviewed literature of canine COVID-19-detection by Meller et al. were normocephalic breeds, which are typically used for scent detection work and are known for their outstanding olfactory capabilities and resilience (e.g., Belgian Malinois, German Shepherd, Labrador Retriever) (4).
Dog health, behavior, and sex
Impact of physical, behavioral, and sex related factors are less investigated than anatomical properties. In addition to a well-functioning olfactory system, a high degree of physical and mental fitness and especially motivation are essential for dogs to focus on the target scent in different environments (76, 93). Although the speed of dogs’ olfactory system is currently unsurpassable by itself, a high level of stamina, agility, athleticism, and motivation in dogs is of great benefit to enhance testing throughput. A high motivation and fitness level can compensate for difficulties in search tasks that prove fatiguing and where target odors are scarce (94–96).
Good cooperative work with humans, especially a balance between obedience and independence, is essential in deployed dogs. On the one hand, independence ensures self-determined searching strategies rather than being potentially misguided by the dog handler. On the other hand, obedience leads to efficiency, where the dog handler can narrow down the area for the search (76).
Aggression toward humans and other animals should be excluded and distractibility and anxiety levels should be as low as possible. Female dogs are generally considered less aggressive and more cooperative (97–99). In terms of neurophysiology, cells in the olfactory bulb of female dogs were shown to be more active than in males. Furthermore, female dogs have a better long-term memory (100). Neutering was assumed to decrease levels of aggressiveness and distractibility (101, 102) and males were shown to perform better in terms of directionality assessment of the target odor (103). Importantly, however, Jamieson et al. summarized that breed and sex finally should not be the crucial cornerstones to assess suitability of detection dogs since training, socialization, experience, and long-term and short-term environmental exposure can have far-reaching influences (76). Sex aspects and potential differences in terms of olfactory performance were not relevant in the screened COVID-19-detection studies (4).
Physical health of deployed dogs should always be guaranteed in the first place from the perspective of ethics and animal welfare and, secondly, not to interfere with their scent performance. Diseases and disorders capable of impacting canine olfaction are, e.g., tumors and injuries in the nasal cavity, infections like aspergillosis, distemper and parainfluenza as well as endocrinological disorders like hyperadrenocorticism, hypothyroidism, and diabetes (104, 105). Also, the function of the vomeronasal organ, supposed to be responsible for detection of pheromones and low-volatile substances, can be impacted by diseases (77). A parotitis was shown to decrease the accuracy of SARS-CoV-2-detection in one study dog (69).
SARS-CoV-2-infections can affect olfaction in people (106) and in some animal models [e.g., hamster (107) and mouse (108)]. Although dogs can be infected by SARS-CoV-2 (109, 110), typically, no clinical signs or mild and reversible signs are observed and susceptibility appears to be low (111). However, biosafety measures should be used when deploying scent detection dog teams (see also sections “Susceptibility of dogs for SARS-CoV-2” and “No viral inactivation”).
Dog mental condition and age
Olfaction is affected by aging processes, and can manifest in atrophic degeneration of the olfactory epithelium, decreased neurogenesis and loss of olfactory cells and their cilia (112). Wells and Hepper showed that younger dogs perform better in olfactory directionality perception than older dogs (103). In addition, pathological aging (e.g., canine cognitive dysfunction) is associated with lower olfactory capabilities (113–115), analogous to human patients with Alzheimer’s disease (116). In 27 studies reviewed by Meller et al. (4) the median age of dogs involved in COVID-19-detection was 3 years (range 0.5–12.0). Age dependent canine olfactory performance in COVID-19-detection cannot be provided as such comparisons were not in the scope of the reviewed studies (4).
Extrinsic factors
Prior scent detection experience
Previous detection experience in dogs is of great advantage. However, inexperienced dogs can be trained and deployed for COVID-19-detection, as well, achieving high diagnostic accuracies (4). For example, Chaber et al. showed that inexperienced dogs were as efficient and accurate as experienced dogs (117). Experienced dogs that are accustomed to the mechanics and environment of odor detection tasks, may only need to learn the new target odor-profile, whereas more time must be allowed for inexperienced dogs to learn to handle the procedure in the setting confidently and efficiently. However, more time should be calculated when changes in setting between training and testing occur (71, 118), even for experienced dogs, in order to ensure understanding of the new search context. Interestingly, the experience of dogs in detecting odors seems to be positively correlated with the ability to cope with more complicated odor information and with stability of long-term memory (100). Inexperienced dogs seem to use olfaction to a lesser extent than experienced dogs (119), highlighting that olfaction is subject to learning processes and plasticity and can be shaped accordingly. Therefore, frequent olfactory exercises with alternating scenarios are beneficial to the training repertoire and experience.
Dog operational environment
High environmental humidity seems to be favorable for scent perception in dogs probably due to increased nasal humidity and enhanced odorant trapping (120), while high temperatures might have a negative impact on the general work flow (121). The dehydration of the mucosal layer in the dog’s nose can decrease the odor detection capabilities (122). The training and sample assessment by the dogs is preferably done in a spacious and conditioned room with controlled temperature and humidity. Still, dogs should be let out in between runs to avoid boredom and increase their odor detection capacity. It was found that dogs had a lower performance when they were exposed to direct sunlight and at higher temperatures (Callewaert et al., in preparation). Kokocińska-Kusiak et al. described environmental factors concerning canine scent detection in the open field, highlighting how sudden environmental changes might impact olfactory abilities (77). However, even in a more spatially restricted searching context, where dogs are involved as screening tools, alterations of external factors should be kept to a minimum. In the study of Vesga et al., COVID-19-detection dogs directly sniffing people in public transport performed at 69% sensitivity. However, those dogs had no training for 2.5 months prior to this testing scenario and still performed well (118). In the study of ten Hagen et al., dogs had high detection accuracy in line-up screenings at concerts (sweat samples, sensitivity 82%, specificity 100%) following the training phase of one to two weeks in a line-up setting (71). Apparently, dogs’ performance was not affected by potentially constantly changing odor-profiles at the testing location, although testing was restricted to a dedicated, roofed, and protected area (71). It may be probable, that a setting of stationary and somewhat isolated dog detection procedures in the form of a checkpoint, e.g., in an isolated roofed space, is more suitable, efficient, and more constant than letting dogs pass through crowds of individuals where olfactory and further environmental sensory distractions may have a higher impact (69, 123). Interestingly, there are also specific and rigorous training programs for explosive detection dogs (e.g., Vapor Wake® dogs) designed for the reliable detection of odorants in aerodynamic wakes of moving individuals in crowds of people (124).
Other external influences on canine olfaction performance can originate from food and drugs. Certain food compositions and ingredients can enhance or decrease olfactory acuity (120), which seems to be dependent on the level of physical exercise in dogs. Angle et al. found benefits to olfactory performance when corn oil supplemented diets were used together with exercise (125), whereas feeding coconut oil supplemented diets without exercise impaired olfaction (126). Interestingly, relatively few studies exist concerning commonly used drugs in dogs and their impact on olfactory performance (77). Especially, metronidazole (127) and steroids like dexamethasone or hydrocortisone (128) have the potential to impair olfaction.
Considerations regarding dog training
Training is the most critical step in predicting the success of dogs in any form of detection work. Dogs without prior odor detection training must learn the value of odor detection, associating a reward with the smell of the target sample. The physical mechanics of searching for and responding to odor in the training and testing environment may be novel to dogs, even to those with prior odor detection experience. Many dogs trained in odor detection (e.g., explosives, narcotics) are trained to recognize an odor, but are not required to discriminate between two very similar odors (i.e., human scent from a diseased state versus human scent from a non-diseased state). Therefore, dogs must learn that the background scent (i.e., individual people) can vary greatly, but the target is the common scent present in only diseased individuals, a task which requires generalization (129). Thus, defining the correct target scent in advance is crucial for the training and subsequent testing in the field (see section “Variability of samples”). Because little is yet known about the COVID-19-odor, target scent definition may seem inconsistent, especially early in a pandemic. Nevertheless, the majority of dogs involved in COVID-19-screening studies performed with high diagnostic accuracies with novel samples in the diagnostic test evaluations (DTEs) (4).
The training method used across COVID-19-studies was operant conditioning with positive reinforcement of correct searching and indication behavior using reward (food, toy, etc.) and the classical conditioning for odor imprinting (presentation and conditioning of the target scent). This method allows for an intrinsically arising motivational boost, which is the determining factor for successful learning. However, training protocols differ depending on the materials, settings, and learning approaches that were used (4). Therefore, there is a lack of standardization of canine training methods for disease recognition, especially for COVID-19, resulting in uncertainty in intra- and inter-dog reproducibility and in translation to real-world scenarios (130). Currently, standardization methods are being developed and a detailed training protocol is provided as supplemental material by Chaber et al. (117). Furthermore, ten Hagen et al. emphasized that integrating other, similarly acting pathogens into training procedures is reasonable in order to decrease the false positive rate and to sharpen the accuracy of dogs for SARS-CoV-2-detection (41). Once dogs learn to reject samples of similar pathogens that appear frequently in a population, sharper discrimination between these pathogens and the target pathogen can be achieved (41).
Olfactory generalization
A key component for consideration during the training process is the scent generalization, which ensures that the dog searches for the common scent-profile of a target condition among all samples of interest rather than recognizing individuals (129). The degree to which generalization is required also depends on the search context. When deploying dogs as a pandemic countermeasure, exposing the dogs to numerous and varied samples from both affected and unaffected individuals will likely lead to higher proportions of correct decisions in an open field screening-scenario, where sources of olfactory confounding factors may be numerous (e.g., age, physiological condition, other diseases, diets, hygiene, habits, environment, etc.). However, too broad of a generalization gradient can also lead to a “dilution effect” of the target scent perception. In this condition, a wide range of different odors is present in the learning repertoire, which differ gradually from the target odor. Thus, too much generalization may mean that dogs also recognize odor-profiles that are merely COVID-19- or SARS-CoV-2-associated. This would lead to an increased false-positive screening rate. Training on a narrow and invariable scent repertoire, on the other hand, can lead to increased discrimination and to confident recognition of very explicit odor patterns or individual samples. This situation can be a problem for screening of a disease-associated odor-profile among plethora of individual odors, potentially leading to an increased false-negative screening rate (129). The main challenge in canine medical scent detection is to assess the origin of the olfactory profile of interest through a myriad of metabolic and other processes, and thereby to define the target odor. The lack of knowledge about the exact odor-profile of COVID-19 and whether this odor-profile is consistent among individuals, represents a “black box” for dog training and makes balanced generalization very challenging. Both balanced generalization and discrimination can be useful, depending on the search context, to enable multi-layered searches, e.g., starting with a broad screening by dog x (e.g., condition) followed by a specific search for a particular target (e.g., pathogen or variant) by dog y (see also section “Standardized sample alternatives”). In order to assess adequate degrees of generalization dogs should be regularly confronted with new samples, both during training and, more importantly, when DTE is conducted. Dogs’ reaction should always be carefully observed, especially when confronted with novel samples, to determine whether generalization processes took place. However, the exact mechanisms of olfactory generalization remain poorly understood (129). An interesting contribution could be made by studies that titrate the intensity of generalization upon detection of disease odor against dogs’ performance. In this way, rates of correct choices for defined samples, or a defined condition, could be compared between dogs trained with different odor-profiles varying in their odorant spectrum. This could provide important conclusions about the relative generalization process. However, it is probable that generalization depends on further properties of odorants, e.g., source, quality, and quantity of odorants, interactions between odorants, etc., as well as on the individual dog’s personality or learning style.
Training duration
Training periods varied between canine COVID-19-detection studies as durations were chosen arbitrarily. Overall, dogs were trained in 2–15 weeks (median 4 weeks), including habituation (e.g., familiarization with scent work, search contexts, and workflow) and/or imprinting, for the detection of COVID-19- or SARS-CoV-2-infections, if no prior COVID-19-scent experience was present. No systematic testing for detection accuracy after different previously defined training periods has been reported and typically the increasing training performance over time was used as a basis for the decision to start the DTE (4). Vesga et al. showed that dogs still performed in an acceptable way (69% sensitivity, 94% specificity) after a training gap of 2.5 months (118). Interestingly, half a week of robust “retraining” of dogs with previous COVID-19-detection experience resulted in comparable high COVID-19-detecting performance as observed after initial training (41, 52, 71). In contrast to the results in the study of Vesga et al. (118), a recently published study highlighted that dogs indeed can remember at least 40 different defined odors, not experienced within 12 months, with 100% accuracy (131). However, the metabolism-induced smell of a disease may be more complex and more difficult to detect (and to remember) than more simple odors, especially among numerous individuals. In addition, it appears important to regularly confront dogs with fresh samples in order to react dynamically to changing disease conditions (e.g., new virus variants) early and reliably. Further research is necessary to assess the potential of canine olfactory memory in order to establish efficient training and break plans for the maintenance of high olfactory performance and for the reduction of fatigue- and boredom-related performance losses.
Training setting
The majority of the 27 reviewed studies by Meller et al. (4) used training with line-ups (19 studies; Figure 2). Scent-wheel training (Figure 3) was used in three studies while five studies used the Detection Dog Training System (DDTS; Figure 4), a device dedicated to the automated, randomized and software-driven presentation of samples (4). In the more classical training methods, great care needs to be taken when exchanging the scent containers, so that sequencing is randomized and blinding of the study is guaranteed which, in addition, requires sufficient personnel and material. Manual and frequent exchange of containers is time-consuming and contamination of containers needs to be avoided. In case of reusable sample containers cleaning after usage and between sessions with different dogs is of crucial importance. While in the traditional approach the dog usually works together with its handler, in the DDTS-approach the dog works independently, significantly decreasing handler bias [“Clever-Hans”-effect (132)], and the sample presentation frequency is high with multiple presentations per minute and an automated reward system. The studies that used DDTS observed generalization quickly despite a limited number of samples used (41, 52, 71, 133, 134). While such automated approaches might enable fast scent conditioning (135), they lack the reference to real-life scenarios where samples (or individuals) would be presented along a line (e.g., airports, schools, events, etc.) to the dog and its handler. Therefore, it is recommended to use a mixed approach using automated methods for initial fast, unbiased scent imprinting and generalization with subsequent habituation and training at line-up or scent-wheel settings to train dogs for systematic and controlled screening in real-life scenarios. It is also recommended to regularly challenge dogs in training with “blank trials”. These trials, which do not contain samples with the target odor, are conducted to evaluate whether dogs perform forced choice decisions, possibly due to rapid frustration after not finding the target odor. Especially, when prevalence of a certain disease is low, such frustration thresholds in detection dogs must be high and monitored. Based on the frustration level of the individual dog, target scent samples should be presented at a dog specific interval to keep frustration levels low (see also section “Ethical Considerations for Using Detection Dogs”). Of the 27 reviewed studies by Meller et al., only nine studies reported the use of blank trials (4).
Susceptibility of dogs for SARS-CoV-2
To date, little is known about the susceptibility of dogs to SARS-CoV-2 and the disease caused by it but initial findings indicate that the susceptibility is low (111). However, studies have shown that dogs can be infected, accompanied by seroconversion, but usually do not show symptoms of disease (110, 136–140). However, a rare association between SARS-CoV-2-infections and the development of myocarditis has been suggested (141). Virus shedding seems to occur to a small extent due to limited titers and during a very short period of time (136, 139, 140). In addition, infection appears to be complicated because only a small percentage of dogs living in households of COVID-19 patients become infected (110, 137, 138). Therefore, there is currently no evidence that dogs play a determining role in virus circulation or transmission to humans, but this should not be ruled out at this stage (110). In contrast to dogs, ferrets and cats seem to be more susceptible to SARS-CoV-2-infections (111, 142, 143).
In the reviewed studies of canine COVID-19-detection, there are no reports of SARS-CoV-2-infections in the involved dogs (4). Of the three studies that conducted PCR-testing of the dogs after the tasks, none of the dogs tested positive (118, 134, 144). However, those studies had high biosafety standards. Biosafety measures should be addressed in training and testing, such as safety containers (118, 134, 145) or chemical and physical viral inactivation measures (41, 52, 71, 133, 134, 145–147). In addition, personal protective equipment should be used to protect involved individuals, even in the case that samples show low infectivity (see section “Sample types”). The following sections discuss the wide variability of sample types and inactivation measures used in reviewed canine COVID-19-detection reports (4).
Samples for use in training and testing
Upper respiratory tract samples like nasopharyngeal (NPS) or oropharyngeal (OPS) swabs and, under certain circumstances, lower respiratory tract samples (e.g., tracheobronchial aspirates) are routinely used for the detection of viral nucleic acid via PCR-techniques (148). Especially RT-qPCR as well as lateral flow immunoassays (LFIA) are currently widely used to identify ongoing infections and rely on the direct detection of viral presence by identifying certain nucleic acids or antigens, respectively (149). Temporal and quantitative presence of SARS-CoV-2-RNA detected via RT-qPCR varies across different human biological sample types and across the duration of infection (150, 151).
Although the virus is essential for the induction of VOCs, the metabolic changes detected by dogs are not necessarily linked to the persistence of the virus, neither locally nor temporally, and VOC-release may lag or precede detectable viral infection (51, 52). A global COVID-19-VOC-profile affecting the whole organism seems to be plausible since Jendrny et al. could show that dogs were able to detect SARS-CoV-2-infections in different body fluids although being trained with only one sample type (134). How essential VOCs change over the time course, disease state, and other disease characteristics still needs to be elucidated. Nevertheless, if biological samples are used for training, it is of crucial importance to “capture” the odor-profile related to the operational usage, e.g., acute and active infections, since only then dogs can be involved as screening tools.
The following sections will give a brief overview of sample types used in the reviewed COVID-19-scent dog literature by Meller et al. (4). Sample handling and options for preserving VOCs in samples (e.g., storage, etc.) can be found in the individual study protocols.
Sample types
Saliva and respiratory secretions
Most studies comparing viral content in saliva and respiratory samples showed saliva samples to contain SARS-CoV-2-RNA in patients of differing age and with differing severities of COVID-19-infections (152). Saliva may substantially contribute to the airborne/droplet transmission (153, 154). It is suggested that in the oral cavity and in epithelial cells of minor salivary gland ducts significant expression of angiotensin-converting enzyme 2 (ACE2) and transmembrane protease serine subtype 2 (TMPRSS2) may contribute to enhanced viral invasion of the host cells by coronaviruses (154–157). High levels of SARS-CoV-2 in saliva are usually already detectable at COVID-19-symptom onset, and usually the loads are similar or slightly lower than in NPS/OPS (151, 152, 158–164). Some studies showed higher viral loads in saliva in some patients or positive tested saliva samples while NPS/OPS presented negative (163, 165–167), which could be due to poor NPS/OPS sampling quality or due to earlier viral manifestation in the oral cavity (165). Nevertheless, a significant decline of viral loads in saliva takes place in the later time points of infection compared to NPS/OPS (151, 158, 159, 162, 164). Interestingly, the grade of salivary viral load does not seem to be associated with disease states (158, 168).
For scent dog detection, saliva was used in the DTEs of six of the reviewed studies, whereas upper airway samples were used in four of the 27 studies (4). Saliva is relatively easy and quick to obtain, but can contain high loads of viable SARS-CoV-2 in infected individuals. Therefore, samples have to be inactivated or presented in a high-security setting in order to protect dogs and their handlers from infection (see section “Pre-processing of samples”). These crucial steps can considerably complicate the training. Jendrny et al. showed that dogs trained with beta-propiolactone (BPL)-inactivated saliva samples can transfer their gained olfactory abilities to the detection of previously unknown non-inactivated SARS-CoV-2-positive saliva samples, and even to previously unknown non-inactivated SARS-CoV-2-positive sweat and urine samples (134). This successful transfer performance simplifies the training (and the real scenario deployment) considerably as it can be extrapolated from the results that regardless of the training samples used, COVID-19 can be detected by trained dogs in the real screening scenario based on a global and specific disease odor. Similarly, Essler et al. showed canine transfer abilities between urine and saliva as well (145). These results support the GC-MS-based studies by Penn et al. and Soini et al. who revealed that general VOC-compositions in human saliva and sweat overlap to a large extent (169, 170). The probable direct infection of the epithelial cells in the salivary gland ducts would provide a high grade of COVID-19-associated VOCs dissolved in saliva and further secretions from the oral cavity functioning as stable carrier media. However, it remains to be elucidated if the fluid-bound condition in saliva might elongate VOC-presence in contrast to non-fluid-bound VOCs as it occurs in sweat/body odor samples. Interestingly, research in biomarkers established the term “salivaomics” since composition of saliva appears to be sensitive to differing disease states of the organism (171). Therefore, it can be speculated that the metabolism-based olfactory fingerprint of COVID-19 in saliva has a relatively specific representation. Further important questions to elucidate are under what conditions and how long saliva samples can be stored without significant loss of characteristic COVID-19-VOCs, and how VOC-production and -dynamics are related to the temporal and clinical course of the infection. One of the authors successfully used frozen aliquots of BPL-inactivated saliva samples for training purposes within a year with success (personal communication).
Sweat and body odor
In contrast to the biological material from the respiratory tract, the potential for SARS-CoV-2-infectivity via sweat or skin is considered negligible. However, based on research on previously described human beta-coronaviruses, attention should be drawn to sweat as one possible vehicle of SARS-CoV-2-transmission (172). Skin, sweat and sebaceous glands express ACE2-receptors (173, 174), making SARS-CoV-2-infections of the resident cells probable (175, 176). However, viral load in epidermis and sebaceous glands was shown to be extremely low by immunohistochemical analysis (175, 177, 178). In contrast, cells in sweat glands contained high levels of viral spike proteins whereas cells in the sweat ducts contained low levels (175). Recalcati et al. tested the sweat of 22 hospitalized COVID-19 patients, the sweat of only five patients was SARS-CoV-2-positive via RT-PCR (179). In contrast, Arslan et al. did not detect viral nucleic acids in multiple sweat samples from both axilla and forehead in 50 patients with COVID-19 (180). Similarly, Fathizadeh et al. did not detect SARS-CoV-2 in sweat from the forehead of 25 patients with COVID-19 (181). These results indicate that despite potential viral presence in sweat glands, viral shedding through skin and sweat is unlikely (but not impossible), allowing for less strict security measures concerning sweat/body odor samples.
Sweat/body odor on pads, gauze, etc., or clothes was used in the DTEs of 20 of the 27 reviewed studies, while direct sniffing of live humans was conducted in only one study (4). Sweat and skin surface also appear to release VOCs which may vary depending on the internal health state of the organism (18). However, bacteria on the skin surface can also influence the metabolism of the released VOCs (6). Whether the composition of the microbiome on the skin has an impact on the COVID-19-associated VOC-profile, and whether these variations may alter scent dog acuity, needs to be elucidated. Nevertheless, body parts frequently or constantly exposed to personal care products, cosmetics, and perfumes are not ideal for sample acquisition since interactions of these products with bacteria and VOCs can occur. Furthermore, clothes intended for SARS-CoV-2-detection by scent dogs should not be washed before being presented to the dogs since important VOCs like organic acids would be destroyed by this process (182). Importantly, the non-homogeneous distribution of apocrine and eccrine glands in the skin implicates different compositions of VOCs depending on the sampled body region (183). Indeed, a varying collection period was applied depending on the body region across the reviewed COVID-19-detection studies. While a short swabbing of the crook of the arm, wrist, face or neck was sufficient for high diagnostic accuracies with sensitivities and specificities above 91% in three studies (69, 70, 134), studies that used axillary sweat or other sweat type chose a longer collection period of around 1–20 min (51, 117, 184–192) or even periods of hours in case of clothes (47, 144). However, in most cases these periods were arbitrarily chosen (4). Callewaert et al. (in preparation) found that 30 min sampling of the underarm skin yielded better canine results versus 15 min sampling.
Compared to saliva or urine sampling and processing, sampling of sweat/body odor on cotton pads or clothes represents a quicker, safer, and more feasible method without inactivation procedures and is well suited for rapid scent dog mass screening. However, it is unclear whether VOCs have a comparable half-life on solid materials such as cotton pads compared to liquids. This could complicate the creation of a long-lasting training sample set if not stored appropriately, but this remains speculative and needs to be elucidated in future studies. For example, Gokool et al. provided preliminary evidence that the specific odor persists for months in worn cotton shirts (193). Sweat samples for COVID-19-detection were stored cooled or at room temperature for around 2 h (194), 24–72 h (70, 185–187, 189, 190, 192), 1 week (188), or even up to 6 months in triple zip-lock plastic bags (69) before being presented to a dog. In some studies, sweat samples (and clothes) were frozen and then presented to the dogs thawed after longer storage periods in order to preserve VOCs (47, 52, 117, 134, 191). Those qualitative and temporal differences in storage did not seem to impact canine performance (4). A combination of training with inactivated saliva or other liquid-bound respiratory material with a stable VOC-profile and testing with rapidly obtainable sweat samples in a real-life scenario could be an effective, safe, and sustainable learning and testing method for infectious disease testing. However, an additional challenge with fresh sweat samples during training is recommended.
Urine
Viable SARS-CoV-2 or its RNA was detected in urine of infected individuals in various studies (153, 195–197). Although some studies showed no detectable virus in urine (198, 199) or, at least, very low viral loads compared to respiratory samples (200), other studies suggested similar viral loads in both sample types (153). These discrepancies might suggest that urinary transmission of SARS-CoV-2 is less likely in general, but that dynamics of viral shedding via urine could be highly dependent on the clinical and temporal stage of the disease (153, 200–203). These concepts are supported by a longitudinal study from Joukar et al. (204) who showed that at clinic admission of COVID-19 patients (n = 100), only 7% of the urinary RT-PCR-tests were positive. The maximal duration of viral persistence in urine was 11 days post admission which was shorter than for all other examined sample types (204). Similarly, Yoon et al. revealed a rapid decline of viral loads in urine to levels below detection limit after only 3 days post admission (151), narrowing the temporal window of virus detection in urine (202). Possible enhanced viral infections of the urogenital tract are plausible due to a prominent expression of ACE2 and TMPRSS2 (205) and renal abnormalities due to SARS-CoV-2-infections cannot be excluded (206, 207).
Urine was used in the DTEs of three studies (52, 134, 145) of the reviewed COVID-19-scent dog detection studies by Meller et al. (4). Chemical analyses of VOCs in urine have been used to detect olfactory fingerprints of different types of cancer (208) and bacterial infections like tuberculosis (209, 210). Since urine contains the intermediate or end products of numerous converging metabolic pathways it can be considered a VOC-rich body fluid (6), although saliva contains a larger variety of VOCs (208). However, due to glomerular filtration VOCs might be more concentrated in the urine than in other body fluids (211). Interestingly, in a direct comparison between saliva, sweat and urine, dogs were able to detect urine from COVID-19 patients with high certainty (median sensitivity 96% and specificity 98%), although the dogs had been trained with saliva beforehand. This might indicate a high concentration of COVID-19-associated VOCs in urine (134). Urine sampling requires more infrastructure, time, and effort and, therefore, is less suitable for mass screening scenarios compared to saliva or sweat. In addition, viral inactivation or high-security measurements should be considered due to the potential risk of viral transmission. Due to the high detection accuracy achieved in the study from Jendrny et al. (134), testing of urine could be used for additional post hoc confirmation after detection of a positive case during screening with other sample types. As with other sample types, however, optimized storage properties still need to be investigated. Furthermore, aspects like diet can impact urinary VOCs significantly (6), which needs to be addressed in future studies.
Breath
Exhaled breath contains high concentrations of various particles and molecules (212–214) including VOCs (60), with differing compositions among certain pathological conditions (6, 215). Although “violent” expiratory events such as coughing and sneezing have previously been considered the main contributors to infectious aerosol and droplet infections (216), aerosols generated by breathing can transmit SARS-CoV-2 and may have a major impact on the infection dynamics (217, 218). Breath VOCs were already investigated in many other diseases (19, 219) and initial approaches have been made in COVID-19 (see also section “The smell of COVID-19”).
Breath samples were used in the DTEs of six of the COVID-19-detecting dog studies, especially in combination with masks (4). The collection and conservation of VOCs from breath is challenging. Lomonaco et al. showed that general VOCs of breath samples stored in sorbent tubes at room temperature were stable up to 72 h (220). A study by Kang and Thomas stated that significant loss in some endogenous breath VOCs was already discernible after 6 months of –80°C storage, although specialized adsorbent tubes were used (221). It is therefore probable that VOCs in masks, similar to cotton pads or clothes, have a storage resilience of shorter duration. However, Guest et al. showed that clothes (socks) gave a stronger specific olfactory signature for dogs than breath samples (face masks) (47). This suggests a high inter-individual VOC-variability in breath samples (60) (see also section “Detection of disease-related VOCs by devices versus dogs”). Furthermore, higher storage temperatures drive a greater loss of breath VOCs on adsorbent materials (222) and longer storage times can lead to exogenous contamination (223). These properties impair the establishment of stable breath sample sets for training. On the other side, subtle skin abrasions in masks, cotton pads, and clothes certainly contribute to a prolonged retention of certain VOC-profiles (see also section “Sweat and body odor”) (6). Due to the impressive acuity of canine olfaction, the potential storage artifacts of breath samples might represent a negligible drawback, this issue however has to be addressed in further studies.
Unlike eNoses, into which the breath sample is usually fed directly, the direct presentation of pure breath in training and in real-life screening to the dogs is challenging, which is not the case for solid-/adsorbent- or fluid-bound biological material. Furthermore, due to technical and hygienic reasons, the throughput rate of current eNoses in real-life screening is lower (minutes per sample) than the throughput rate of trained dogs evaluating line-ups with self-taken sweat samples (seconds per sample) (69, 71) (see also sections “Detection of disease-related VOCs by devices versus dogs” and “Sweat and body odor”). In terms of breath VOCs, masks (or specialized adsorbent material) would be more suitable than pure exhaled breath both for canine training and screening. However, generating those mask samples generally required a longer duration of approximately 10 min to 24 h with a median of 180 min (47, 144, 146, 147, 190). Vlachová et al. however, conducted breath sampling on sterile surgical compresses of only 3 min (189). Furthermore, due to the evidence of airborne/droplet infections for SARS-CoV-2, biosecurity associated with the immediate presentation of pure breath samples is more complex than presentation of carrier material-bound samples.
Variability of samples
Origin of samples for training and DTE purposes is an important factor due to the potential contaminating impact of environmental VOCs (6, 208, 215, 224). For example, it could be a major issue if samples from SARS-CoV-2-positive patients originated from only one facility, conditioning dogs on facility-associated smell rather than SARS-CoV-2-associated smell. Likewise, if SARS-CoV-2-negative patients are collected from a different environment, e.g., the community, and SARS-CoV-2-positive patients are all collected from hospital environments, the systematic difference between the samples may lead to inaccurate responses by the dogs.
Similarly, geographical conditions may also have an impact on VOC-profiles (225). Chaber et al. showed slight differences in canine olfactory performance depending on the geographical origin of samples (117). However, special care should be taken to ensure that handling of utensils during sampling and processing proceeds in the same manner for both negative and positive samples across testing locations (47, 226, 227). In addition, Callewaert et al. (in preparation) found that dissimilarities in canine performance occur depending on the carrier material (e.g., cotton pad, cotton gauze, commercial odor carrier, etc.). Therefore, it is advised to use one and the same carrier throughout training and DTE.
For training and DTE purposes, a large representation of different demographic aspects (sex, age, etc.), localities of sample origin, and temporally different stages of infection among SARS-CoV-2-positive and -negative samples is crucial to adequately map the olfactory fingerprint of the disease. In addition, other aspects such as pre-existing infectious and non-infectious pathological conditions, recovered SARS-CoV-2-infections, Post-COVID-19 condition, COVID-19-vaccination status, or differing virus variants may play an important role for VOC-patterns and are subject of current research (51, 52, 69, 71). ten Hagen et al. studied the ability of dogs to discriminate between SARS-CoV-2-infections and other viral respiratory infections in NPS/OPS and infected cell cultures, when trained with saliva from SARS-CoV-2-positive individuals or with SARS-CoV-2-infected cell culture supernatants. Although sensitivity was lower (61.2–75.8%) than in other studies from the same laboratory, dogs rejected the samples of other viral infections in the DTE more often than SARS-CoV-2-infected samples, which is reflected by a high specificity of 90.2–95.1%. This indicates that further respiratory viral diseases defined as SARS-CoV-2-negative samples should always be integrated into training procedures in order to enhance diagnostic acuity for SARS-CoV-2 (41).
In terms of infection state, the crucial intervals dogs should be able to recognize is any phase in which viable virus is shed in order to contain the pandemic effectively. Therefore, samples across all phases of infection should be used for training in order to reliably indicate all potentially changing relevant odor-profiles in the course of infection. However, further research is needed to evaluate if and how dogs are able to transfer their olfactory detection abilities from a certain stage of disease to another. Recent studies found that dogs which were trained with samples from acute SARS-CoV-2-infection did not indicate patients with Post-COVID-19 condition as positive, when tested versus acute infection. Nevertheless, when tested against samples from healthy individuals, Post-COVID-19 condition samples were identified (51, 52). These results might suggest a titration effect, which could be based on a slow gradual decomposition of characteristic VOCs even if the virus is only residually or not present anymore.
Furthermore, an appropriate mapping of disease severity (e.g., asymptomatic, mild, severe) should be taken into account and integrated into training. However, COVID-19-VOC-measurement indicated that there was no relationship between VOCs and viral loads (34) or disease states, although mainly severe cases were included (36). Importantly, more research is needed to explore to what extent PCR-cycle threshold values, representing viral loads, influence canine olfactory performance [see also (47)].
Dogs can even be trained to certain concentration differences of the target scent (228). For example, this is used in diabetes alert dogs, which detect increases or decreases of blood glucose values of patients beyond predetermined levels (229). This emphasizes that the samples used in canine training procedures must be as versatile as possible. The issues described in this section will be minimized when further efforts are made in the profiling of critical SARS-CoV-2-VOCs and in the processing techniques of samples in order to reduce olfactory noise from potential exogenous and irrelevant endogenous factors (215). A crucial question which arises is whether training conditions can be reduced to the lowest common denominator by, for example, training with pure viral proteins or proteins produced in cell cultures or animal models (see section “Standardized sample alternatives”). Cell cultures were used in one (41) of the 27 reviewed studies by Meller et al. (4).
Pre-processing of samples
Many protocols for inactivation of viral pathogens with differing grades of loss of functional and structural viral integrity exist. The main purpose of viral inactivation in scent dog detection studies is the safe handling of training samples for animals and humans. On the other side, olfactory fingerprints of samples deriving from SARS-CoV-2-infections have to be preserved, probably requiring gentle inactivation methods. Different approaches up to renunciation of inactivation procedures were used in the reviewed COVID-19-scent dog literature (4), which is discussed below. The study from Jendrny et al. revealed that inactivated samples can be used for training to subsequently screen non-inactivated “armed” samples with a median sensitivity and specificity of 84 and 95%, respectively (134).
Beta-propiolactone
Beta-propiolactone (BPL) is an organic chemical compound which has historically been used for effective inactivation of various known viruses (230), especially in the field of vaccine development (231–234). BPL inactivates SARS-CoV-2 as well (235). Its inactivating properties are based on opening its lactone ring which is unstable in aqueous media and highly reactive (230). Due to rapid hydrolyzation in aqueous media, the substance is transformed within a few hours to non-toxic 3-hydroxypropionic acid making it highly suitable and safe for biological preparations (236). Despite the rapid degradation, viral activity has usually subsided long before the last detectable residuals of BPL in samples have been measured (230). BPL appears to have affinity for viral nucleic acids blocking viral replication while mostly sparing the protein structures, which preserves the immunogenicity of the virus. However, not all the organic chemical modifications coming from BPL are elucidated and proteins may be affected as well (237). On the other hand, Determann and Joachim showed that a higher reactivity toward certain functional groups of amino acids results from a lower hydrolyzation capacity of the medium (238), highlighting that water is a preferred nucleophilic reagent of BPL. In summary, variations in nucleophilic characteristics of the reaction with BPL and the “nucleophilic potential” as well as further physicochemical properties of the medium might explain why varying quantitative and qualitative dynamics among reaction products from different organic compounds exist (237). In the study from Jendrny et al. dogs did not smell a relevant difference between BPL-inactivated (training) and non-inactivated (DTE) SARS-CoV-2-infected samples (134). Although more research is needed in this field, this might indicate that nucleophilicity of relevant VOCs is low and that microenvironmental aspects of the samples could further contribute to the lack of involvement of respective VOCs in the reaction with BPL so that those are kept preserved. Furthermore, it is possible that the BPL-manipulation has no effect on the high discriminatory power of the dog’s olfactory system. It is noteworthy that in the first work by Jendrny et al., non-inactivated negative samples were used in addition to BPL-inactivated negative samples (133). The dogs did not indicate the latter more often than the former even though they were trained with BPL-inactivated positive samples (133). Only three of the reviewed studies used BPL for viral inactivation in their DTEs (41, 52, 133). However, in terms of safety versus VOC-preservation, BPL inactivation represents a highly effective and reasonable method.
Heat
Heat inactivation is a possible and common method to destroy viral pathogens effectively (239, 240). At the same time, maintenance of antigen integrity is important to preserve the diagnostic value of samples, e.g., for serological analysis (240–247). Heating methods can prevent infectivity of SARS-CoV-2 and at the same time preserve RNA when appropriate temperatures are applied (247). In contrast, BPL preserves proteins but not RNA (see above). Heat has denaturizing properties on proteins and other compounds leading to disruption in the interaction between virion and cell. Even slight alterations might also have a crucial and persistent impact on quality of the VOC-emitting properties of organic material, changing VOC-concentrations and their chemical composition. In addition, Lomonaco et al. showed that heat treatment is able to alter VOC-composition in human breath samples (220).
Heat inactivation or treatment in the DTEs was used in three (118, 145, 147) of the reviewed studies (4). Essler et al. (145) trained dogs with detergent-inactivated urine (see below) and tested the dogs for detection of heat-inactivated urine. Especially when dogs were confronted with a novel heat-inactivated sample, overall sensitivity was only 62%, whereas specificity was 98%. This may indicate that – at least in relation to detergent treatment – heat may alter critical COVID-19-VOC-profiles to a certain extent inducing uncertainty, or that the use of detergent inactivation made the odor more obvious. However, it has to be mentioned that these transfer trials consisted of only one set of presented samples to eight dogs. Interestingly, the performance of dogs, which were trained with heat-inactivated samples and tested with new heat-inactivated samples, deteriorated significantly, which possibly was due to a poor generalization process as sample availability was limited at the time the experiments were performed (145). Possibly, the process of heat-inactivation might produce different VOC-profiles among individual samples, depending on their original chemical and physical composition. The learned VOC-spectrum would thus present too broad to be finally used in detection of COVID-19-specific smell with adequate generalization and high diagnostic acuity. The assumption of global and individual changes in the key VOC-profile through heat-inactivation is also supported by the fact that the olfactory transfer performance from heat-inactivated urine-training to heat-inactivated saliva-testing produced very low sensitivities in two trials (11 and 22%, respectively), whereas the accurate recognition of negative samples was maintained (specificity of 94 and 100%, respectively) (145). However, only one positive sample was presented per trial across nine dogs. Furthermore, the discussed aspects of heat treatment remain speculative since other possible complicating factors have to be taken into account. In contrast, Jendrny et al. showed that dogs’ transfer performance from BPL inactivated training samples to completely novel non-inactivated samples of the same and even different type is maintained at the same or even higher levels (134). BPL seems to retain the assumed global COVID-19-associated smell. Heat-inactivation appears to be more time-saving and cheaper than BPL-inactivation, but the former might lead to less robust learning results in dogs. Consistent with those statements, Salgirli et al. have also reported that dogs initially had problems recognizing heat-inactivated masks worn by COVID-19 patients when previously trained with non-inactivated masks (147). In contrast, Vesga et al., who used heat treatment in order to prevent proliferation of microbiota, reported a high performance quality of dogs, however, the treatment was not further specified (118).
Ultraviolet radiation
Ultraviolet-C (UV-C) radiation may be used to inactivate coronaviruses effectively (240, 248–250). It acts mainly by photochemical conversions of heterocyclic bases in the structure of nucleic acids without spontaneous reversion (251–253). Amino acids are affected to a lesser extent while carbohydrates and lipids are hardly modified (251). Mendel et al. used 10 minutes of UV-C-irradiation (254 nm) per side of mask material for SARS-CoV-2-positive cases, for canine training and for DTEs (146). Similarly, Salgirli et al. also used UV-inactivation (147). However, it is a significant concern of methodology in both studies that it is not clearly stated whether negative samples were also inactivated in order to control for potential pronounced or subtle UV-induced alterations in COVID-19-associated VOCs. In an additional experiment Mendel et al. showed that UV exposure did not result in statistically significant alterations in headspace solid phase microextraction GC-MS-profiles of at least 36 typical human-derived scent compounds pipetted on unused masks, suggesting a lack of significant photocatalytic effects on these VOCs (146). Conversely, UV-radiation of different wavelengths (especially UV-C) and dosage can have a great photocatalytic impact on gaseous emissions and VOCs by eliminating many of them from air samples, even within seconds (254–257), or from liquid media (258, 259). Therefore, the extent to which specific COVID-19-associated VOCs are altered by UV-irradiation remains uncertain and needs to be elucidated. If there are alterations, it has to also be clarified whether the discriminatory power of the canine olfactory system is nevertheless sufficient to compensate for those changes.
However, Mendel et al. (146) reported in two cases that trained dogs were able to indicate locations at workplaces where SARS-CoV-2-infected individuals had been situated 3–4 weeks prior to canine inspection. It would represent a promising indication that UV-irradiation might have no relevant effect on COVID-19-associated VOCs and that the temporal range of detection might extend well beyond acute infections, however, these are only few individual cases reported (146) and it remains questionable whether COVID-19-associated VOCs persist in a confined area for such a long time without appropriate storage [SARS-CoV-2 itself survives only a few days in the environment (260)]. In summary, comparative studies of training with UV-inactivated and DTEs with new, non-inactivated samples under high security standards (see also section “Susceptibility of dogs for SARS-CoV-2”) are an essential step to ultimately verify the suitability of UV-inactivation for establishing canine training sample sets. Although the actual process of viral inactivation by UV takes longer than by BPL (230), the use of UV would be a time-saving and an ecological method since, in contrast to BPL- or detergent-inactivation, no chemicals, no targeted chemical manipulations of the samples, and no waiting time for hydrolysis are required.
Detergent–solvent
Detergent/solvent applications are a further method for efficient viral inactivation by complete destruction of the lipid membrane of enveloped viruses while preserving the structure of proteins from the virus and from the biological microenvironment (261–263). This method is widely and commercially used especially in the treatment of therapeutic human plasma, as it robustly destroys enveloped viruses while at the same time retaining physiological activity levels of plasma proteins (262, 264). Nonidet™ NP-40 in combination with further detergents seems to successfully disrupt coronavirions (240) and was used by one canine COVID-19-detection study for urine inactivation (145). A possible VOC-alterating effect of Nonidet™ NP-40 or the closely related substance Triton X-100 on VOCs in treated samples is not elucidated. They represent gentle inactivation methods, but it might be assumed that lytic effects on membranes of contained cells (265) might slightly change the biochemical properties of those samples. Triton X-100 has a vapor pressure of 130 Pa at 20°C and is therefore considered an organic volatile substance (266). It can therefore be assumed that the detergents themselves change the odor-profile of the samples while they are still dissolved. In order to clarify these issues, comparative canine olfaction studies with both detergent-inactivated and non-inactivated positive and negative samples are necessary. However, Essler et al. (145) could show that cognitive transfer from detergent-inactivated to heat-inactivated samples is possible. Although sensitivities decreased, this may also be due to heat-inactivation [(145); see also section “Heat”]. Finally, chemical treatments with detergents or BPL are more environmentally damaging, time-consuming, and eventually more expensive than, for example, the use of UV-C. Nevertheless, they appear to allow satisfactory and safe olfactory transfer to non-inactivated samples in canine COVID-19-detection (134, 145).
No viral inactivation
No inactivation can represent a biosafety issue, but is probably also the best method for the preservation of crucial VOC-profiles. SARS-CoV-2 can survive a few days in the environment depending on the type of contaminated surface (260). In secreted biological material like aerosols, virus was shown to be infectious for minutes to hours (260, 267) whereas other studies show higher viral activity up to 21 days in different body fluids like e.g., sputum, saliva, urine, and blood, depending on seasonal factors (268). No virus inactivation was used by the majority of reviewed canine COVID-19-detection studies in their DTEs (n = 21) (4). Sweat samples were the main material used without inactivation which per se have no high infectivity (see also section “Sweat and body odor”). Similarly, four studies did not use inactivation of mask or clothes samples (47, 144, 147, 190). Apparently, the material on which sweat or body odor was collected impacts the viral persistence as well, since cotton and related material seems to ensure decomposition of its RNA within minutes (269, 270). The duration between sample acquisition and presentation to dogs was variable across studies between hours and months (see also section “Sweat and body odor”). However, in some reports, inactivation was omitted also in other sample types like saliva (184) and nasopharyngeal secretions (144) without use of further biosafety measures. Leaving out inactivation generated robust and good results (4) suggesting that characteristic VOCs outlast the virus presence or at least high viral loads. Nevertheless, independent of inactivation status, special safety measures should be used (118, 134, 145), e.g., Training Aid Delivery Device (TADD) containers (134, 145), which are supposed to allow odor particles to pass through but not droplet- or particle-bound virions, in order to protect both animals and humans (134). This is especially interesting for training purposes where body fluids with higher viral loads may be used. For detection of other infectious diseases, corresponding data about pathogen dynamics in body fluids and environment should be used, or reliable data should be generated first in the case of future emerging zoonotic diseases in order to determine susceptibility of dogs to pathogens of interest and to guarantee adequate safety.
Standardized sample alternatives
Training sample sets derived from naturally obtained human biological fluids can be used for extended periods of time. However, more research is needed in appropriate storage conditions (see section “Sample types”). In addition, acquiring samples is not trivial both from an ethical and logistic sense, especially early in a pandemic or while pandemic dynamics are low. Furthermore, storage duration and divergent storage conditions can affect VOC-patterns (221, 271, 272). There may also exist uncertainties regarding true infection status with possible false negative or false positive PCR-status potentially corrupting the sensitive training process for the right olfactory cue, differences in temporal and clinical infection states, demographic differences, etc.
Producing specific COVID-19-associated VOC-profiles artificially for dog training purposes represents a challenging endeavor although first approaches with a “VOC-cocktail” have been conducted in combination with eNoses (273). However, sensor array composition of the eNose and environmental influences still represent a major limitation and studies are merely scratching the surface of decoding the volatilome of SARS-CoV-2-infections (see section “The smell of COVID-19”). For the current state of the canine COVID-19-detection research, it was important to cover the majority of VOC-variations, which can emerge from varying disease-associated factors, for adequate broad training and generalization. Nevertheless, it is only the attempt to “catch” the true critical COVID-19-odor of an active infection in an as broad as possible way, for the simple reason that the critical VOC-composition is not known yet but at the same time early investigation of anti-pandemic measures appeared reasonable. In this way, dogs were taught to perceive key signals from a broad array of positive samples, which were not present in a broad array of negative samples. Therefore, dogs did not learn to detect an absolute COVID-19-VOC-profile, but a certain scent-profile of relative difference to what healthy individuals did not express.
The VOC-hypothesis is based on viable metabolic entities with the result of VOC-production, which may have fingerprint-like properties for certain pathological conditions, e.g., viral infections. Some have suggested that dogs might be able to detect viral proteins, i.e., spike proteins (51, 52), which could be perceived by olfaction without any metabolic intermediate step. Amino acids are not among the substances typically defined as odorants, and to date have been little studied in the context of odor perception, except in fish (274–278). Humans have been shown to be able to distinguish among certain amino acids by olfaction (279, 280). Whether dogs are able to smell parts of the pure SARS-CoV-2-proteins and reliably discriminate it against other distractors is currently being investigated. In this context, the function of the vomeronasal organ in dogs should be emphasized, which serves as an additional olfactory organ for intra-species communication through pheromones and is located rostrally at the bottom of the nasal cavity (5). Interestingly, in contrast to the main olfactory organ, the vomeronasal organ is capable of detecting non-volatile molecules of higher molecular weight, such as proteins (281), which might indicate the presence of different receptor cell types in both olfactory organs (282).
If dogs are able to smell viral proteins, a standardized, broad, and sustainable training infrastructure based on appropriately manufactured proteins could be established and research is already underway. Safety would be guaranteed due to the absence of the viable virus, however, the risk of contamination of such sensitive samples is high. In addition, costs of sampling body fluids versus production of protein samples must be considered. An essential consideration, however, is the periodic emergence of new variants of SARS-CoV-2 with differing mutations in spike genes and protein expression (283, 284). When dogs are trained on a single protein the spectrum of detection would be extremely narrow and certainly highly specific, increasing olfactory discrimination (129), but it has to be studied whether it would suffice to cover different virus variants. It would therefore seem reasonable to mix the variants during training sessions according to the current viral occurrence in the population. However, it may take some time before the next corresponding protein is available after discovery and identification of a new variant.
It is probable that the impact of viral variants on variation in COVID-19-VOC-patterns is less pronounced. Chaber et al. stated that dogs had no difficulties recognizing the virus despite being confronted with different strains in biological samples (117). On the contrary, Kantele et al. showed a significant difference in accuracy between variants, when the training included only biological samples with the wild-type virus (69). Furthermore, by using only proteins for training, it would be essential that viral material is present and “readily accessible” in the screening samples of diseased individuals, which is not always the case for sweat/body odor or urine as described above (see section “Sample types”). In contrast, only infected individuals who acutely excrete the virus would be detected in this way sparing individuals who do not shed the virus anymore but still express COVID-19-VOC-patterns. Nevertheless, this can be deceptive because the viral load may vary temporally across the samples while the individual is still infectious or may depend on vaccine-induced immune response (see section “Sample types”).
In order to circumvent these issues, supernatants from infected human cell cultures (41) could be used for additional training with negative/distractor samples belonging to the same culture or with different cell lines among positive and negative samples, profiting from the advantages of VOCs. The combinatorial approach of VOCs and proteins (e.g., one or more “sets” of specifically trained dogs, see section “Olfactory generalization”) could maintain high levels of sensitivity in general screening and be used in special confirmatory cases as a highly specific detection method for certain dangerous viral variants, which could be continuously updated in dogs’ olfactory memory (117).
Although cell cultures are a very interesting alternative, it should be noted that the VOC-profile does not necessarily correspond to the versatility of VOC-patterns from naturally obtained biological samples. Murarka et al. trained dogs with an ovarian cancer cell line and showed that olfactory transfer or switch from cell culture to samples of patients with ovarian cancer did not readily occur in dogs (285). Similarly, there was a lower detection ability in SARS-CoV-2-positive cell culture supernatants after dogs had been trained with naturally acquired saliva samples (41). These problems could be circumvented to some extent by using different cell lines in cell cultures, but mimicking the olfactory versatility of naturally acquired samples remains difficult. Another alternative may be the use of SARS-CoV-2-training samples from animal models. Nevertheless, the question of effective translation to human derived VOCs needs to be addressed (286). Despite the great advantages of sample alternatives with regard to trainability and standardization, the use of “real” biological samples will probably still be necessary to prepare dogs for real-life screening scenarios.
Target and screening population and the operational applicability
The World Health Organization (WHO) and the German Paul Ehrlich Institute (PEI) recommend thresholds for diagnostic sensitivities and specificities for point-of-care-antigen tests to be more than 80% and more than 97%, respectively (287). 78% of reviewed canine detection studies showed ≥ 80% sensitivity and 60% of studies showed ≥ 95% specificity. Therefore, dogs’ detection is in line with or even better than other rapid diagnostic tests. Dogs achieved even better performances when only considering high-quality studies with a low risk of bias (4).
However, when considering the entire components influencing the dog as a detection system, it has to be taken into account that the characteristics of the population to be tested has its impact on the accuracy as well. Besides the actual prevalence of COVID-19 within the target population to be screened the detection performance differs between different populations and search scenarios, which has a direct impact on the practicality. Therefore, the calculation of expected positive and negative predictive values is crucial for the decision on screening scenarios in order to avoid any vilification of the dogs’ detection (Table 2).
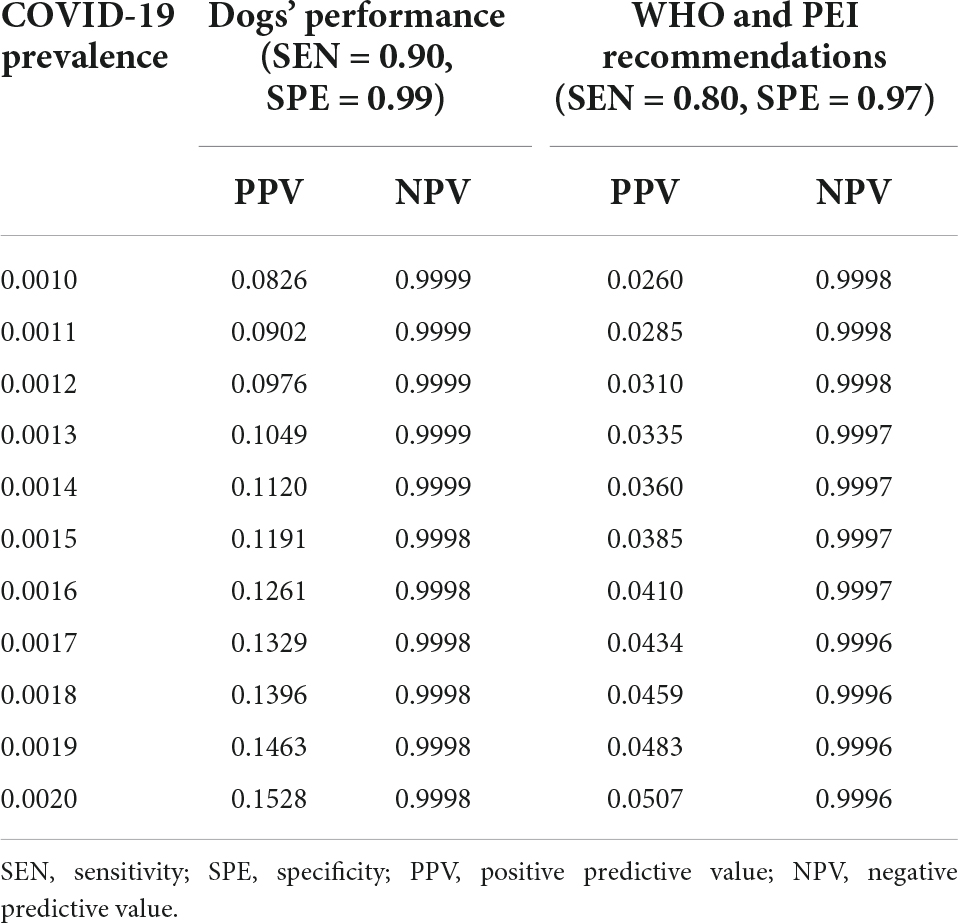
Table 2. Positive and negative predictive values for dogs’ performance of 90% sensitivity and 99% specificity and for the recommendations of the World Health Organization (WHO) and Paul Ehrlich Institute (PEI) among different COVID-19 prevalences in the target population.
It is furthermore important to note that not all studies relied on a single dog’s decision to determine sensitivity and specificity. In particular, in some cross-sectional studies, decisions from multiple dogs were used to ensure certainty in defining the infection/disease-status of tested individuals (69, 71, 191, 194). Those considerations, which also might depend on the number of available trained dogs, are important especially for the planning and conduction of a screening test. Furthermore, changing and distracting environmental factors should be reduced or avoided in the operational screening setting (see also section “Dog operational environment”).
Dog detection as the one health approach to tackle COVID-19
The increase of zoonotic infectious diseases highlights the importance of collaborative, multisectoral and interdisciplinary work to address challenges that could impact public health, animal health and production, and environmental conservation. The World Health Organization (WHO), the Food and Agriculture Organization of the United Nations (FAO), the World Organization for Animal Health (WOAH) and the United Nations Environment Programme (UNEP) have established an intersectoral collaboration aiming to implement initiatives under the concept of “One Health” to address main global problems at the human-animal-environment interface (288). The four organizations are working together to mainstream One Health so that they are better prepared to prevent, predict, detect, and respond to global health threats and promote sustainable development. The use of COVID-19-detecting dogs is a great example of using this concept to respond to the current COVID-19-pandemic. In this review, we showed how multi-sectoral communication and joint work resulted in the generation of evidence that the use of dogs trained to detect SARS-CoV-2-infections has been shown to be a rapid, mobile, and non-invasive tool for early detection of affected individuals. Collaborative efforts are crucial to minimize the rapid viral transmission requiring massive testing (289, 290). The use of detection dogs to pre-screen infections among the population could overcome the overloaded response capacity of laboratories due to the higher number of required tests, the lack of needed reagents to perform these tests, and technical issues in sampling infected individuals (i.e., inappropriate sample collection, storage, or transportation) or false-negative results related to the disease status with low viral multiplication levels (291–293).
Conclusion
Dogs can detect samples from SARS-CoV-2-infected individuals with a high degree of diagnostic accuracy. However, the search context, study design and quality of the current studies varied considerably, and only a small percentage of studies were of high quality with a low risk of bias. In contrast to an industrially produced test kit, dogs and their olfactory performance are naturally subject to many variations. In addition, disease detection involves difficult to measure and volatile amounts of substances and little is known about the olfactory dynamics of a pathological process, making it difficult to control the process of adequate odor imprinting. However, the evidence of canine COVID-19 recognition has been replicated by several different groups, and the dog proved to be an incomparably fast detection tool. Importantly, in epi/pandemic conditions, dogs can be trained quickly with a good level of sensitivity before specific laboratory methods are available, helping with isolation of infected patients presenting with or without symptoms. Therefore, further research on influences of the odor profile (or the perception of it) by factors such as training sample number and type, sampling method, inactivation type, training procedures, dogs’ personalities, environment, translation from training to test scenario, etc. proves to be very important for harmonization and optimization of canine scent detection and for maintenance of high study quality. Those considerations pave the way for the canine olfaction to become a reliable, stable and quick test method. Thus, standardization and validation processes such as those used in the field of drug and explosive detection dogs are urgently needed, if medical detection dogs should be deployed in the field to detect samples from SARS-CoV-2-infected individuals.
We recommend the use of dogs as VOC-detectors in mass screenings as a quick, highly adaptive, and effective countermeasure both at the emergence and also in the further course of a pandemic, provided that sufficient numbers of diverse positive and negative, high quality, safe samples for training purposes can be generated early, the pathophysiological condition of those samples is known with a high certainty, and that training procedures, dogs, and their handlers are certified similarly as described for scent detection in explosives (294).
Author contributions
HV and SM discussed and planned the form of the comprehensive analysis. SM wrote the manuscript and conducted the main literature research. All other authors have contributed significantly to the completion and development of the review and provided their expert opinion. All authors have read and approved the final manuscript.
Funding
This Open Access publication was funded by the Deutsche Forschungsgemeinschaft (DFG, German Research Foundation) – 491094227 “Open Access Publication Funding” and the University of Veterinary Medicine Hannover, Foundation.
Acknowledgments
We would like to thank Stéphane de la Rocque (WHO) for critically reviewing the manuscript. Furthermore, we would also like to thank Sonja von Brethorst and Ulrich Stamm for providing the image for Figure 2.
Conflict of interest
JGL was employed by the Arctech Innovation. HE and JE were employed by the Kynoscience UG.
The remaining authors declare that the research was conducted in the absence of any commercial or financial relationships that could be construed as a potential conflict of interest.
Publisher’s note
All claims expressed in this article are solely those of the authors and do not necessarily represent those of their affiliated organizations, or those of the publisher, the editors and the reviewers. Any product that may be evaluated in this article, or claim that may be made by its manufacturer, is not guaranteed or endorsed by the publisher.
Supplementary material
The Supplementary Material for this article can be found online at: https://www.frontiersin.org/articles/10.3389/fmed.2022.1015620/full#supplementary-material
Supplementary Figure 1 | Detailed mind map representing areas of interest and related aspects that played a role in the reviewed canine COVID-19-detection studies by Meller et al. (4) and have been highlighted by the experts in this publication.
References
1. Wiersinga WJ, Rhodes A, Cheng AC, Peacock SJ, Prescott HC. Pathophysiology, transmission, diagnosis, and treatment of coronavirus disease 2019 (COVID-19). JAMA. (2020) 324:782–93. doi: 10.1001/jama.2020.12839
2. Chen C-L, Lai C-C, Luh D-L, Chuang S-Y, Yang K-C, Yeh Y-P, et al. Review of epidemic, containment strategies, clinical management, and economic evaluation of COVID-19 pandemic. J Formos Med Assoc. (2021) 120:S6–18. doi: 10.1016/j.jfma.2021.05.022
3. Mercer TR, Salit M. Testing at scale during the COVID-19 pandemic. Nat Rev Genet. (2021) 22:415–26. doi: 10.1038/s41576-021-00360-w
4. Meller S, Twele F, ten Hagen NA, Jendrny P, Volk HA Diagnoses by dog noses? Real-time detection of SARS-CoV-2 infections by trained dogs. In: Paper Presented at: 32nd Annual Meeting of the German Society for Cytometry (DGfZ). Berlin: Charité – Universitätsmedizin Berlin (2022).
5. Jendrny P, Twele F, Meller S, Osterhaus ADME, Schalke E, Volk HA. Canine olfactory detection and its relevance to medical detection. BMC Infect Dis. (2021) 21:838. doi: 10.1186/s12879-021-06523-8
6. Shirasu M, Touhara K. The scent of disease: volatile organic compounds of the human body related to disease and disorder. J Biochem. (2011) 150:257–66. doi: 10.1093/jb/mvr090
7. Broza YY, Mochalski P, Ruzsanyi V, Amann A, Haick H. Hybrid volatolomics and disease detection. Angew Chemie Int Ed. (2015) 54:11036–48. doi: 10.1002/anie.201500153
8. Angle TC, Passler T, Waggoner PL, Fischer TD, Rogers B, Galik PK, et al. Real-time detection of a virus using detection dogs. Front Vet Sci. (2016) 2:79. doi: 10.3389/fvets.2015.00079
9. Kamal F, Kumar S, Edwards MR, Veselkov K, Belluomo I, Kebadze T, et al. Virus-induced volatile organic compounds are detectable in exhaled breath during pulmonary infection. Am J Respir Crit Care Med. (2021) 204:1075–85. doi: 10.1164/rccm.202103-0660OC
10. Issitt T, Wiggins L, Veysey M, Sweeney ST, Brackenbury WJ, Redeker K. Volatile compounds in human breath: critical review and meta-analysis. J Breath Res. (2022) 16:024001. doi: 10.1088/1752-7163/ac5230
11. Pirrone F, Albertini M. Olfactory detection of cancer by trained sniffer dogs: a systematic review of the literature. J Vet Behav. (2017) 19:105–17. doi: 10.1016/j.jveb.2017.03.004
12. Hardin DS, Anderson W, Cattet J. Dogs can be successfully trained to alert to hypoglycemia samples from patients with type 1 diabetes. Diabetes Ther. (2015) 6:509–17. doi: 10.1007/s13300-015-0135-x
13. Catala A, Grandgeorge M, Schaff J-L, Cousillas H, Hausberger M, Cattet J. Dogs demonstrate the existence of an epileptic seizure odour in humans. Sci Rep. (2019) 9:4103. doi: 10.1038/s41598-019-40721-4
14. Maa E, Arnold J, Ninedorf K, Olsen H. Canine detection of volatile organic compounds unique to human epileptic seizure. Epilepsy Behav. (2021) 115:107690. doi: 10.1016/j.yebeh.2020.107690
15. Maurer M, McCulloch M, Willey AM, Hirsch W, Dewey D. Detection of bacteriuria by canine olfaction. Open Forum Infect Dis. (2016) 3:ofw051. doi: 10.1093/ofid/ofw051
16. Taylor MT, McCready J, Broukhanski G, Kirpalaney S, Lutz H, Powis J. Using dog scent detection as a point-of-care tool to identify toxigenic Clostridium difficile in stool. Open Forum Infect Dis. (2018) 5:ofy179. doi: 10.1093/ofid/ofy179
17. Guest C, Pinder M, Doggett M, Squires C, Affara M, Kandeh B, et al. Trained dogs identify people with malaria parasites by their odour. Lancet Infect Dis. (2019) 19:578–80. doi: 10.1016/S1473-3099(19)30220-8
18. Kataoka H, Saito K, Kato H, Masuda K. Noninvasive analysis of volatile biomarkers in human emanations for health and early disease diagnosis. Bioanalysis. (2013) 5:1443–59. doi: 10.4155/bio.13.85
19. Rattray NJW, Hamrang Z, Trivedi DK, Goodacre R, Fowler SJ. Taking your breath away: metabolomics breathes life in to personalized medicine. Trends Biotechnol. (2014) 32:538–48. doi: 10.1016/j.tibtech.2014.08.003
20. Cambau E, Poljak M. Sniffing animals as a diagnostic tool in infectious diseases. Clin Microbiol Infect. (2020) 26:431–5. doi: 10.1016/j.cmi.2019.10.036
21. Bauër P, Leemans M. Remote Medical Scent Detection of Cancer and Infectious Diseases With Dogs and Rats: A Systematic Review. Research Square [Preprint]. (2021). Available online at: https://assets.researchsquare.com/files/rs-753298/v1/3d37a663-6815-403d-ba28-60910f3f14e6.pdf?c=1644333652 (accessed August 7, 2022).
22. Horvath G, Järverud GA, Järverud S, Horváth I. Human ovarian carcinomas detected by specific odor. Integr Cancer Ther. (2008) 7:76–80. doi: 10.1177/1534735408319058
23. Ehmann R, Boedeker E, Friedrich U, Sagert J, Dippon J, Friedel G, et al. Canine scent detection in the diagnosis of lung cancer: revisiting a puzzling phenomenon. Eur Respir J. (2012) 39:669–76. doi: 10.1183/09031936.00051711
24. Kybert N, Prokop-Prigge K, Otto CM, Ramirez L, Joffe E, Tanyi J, et al. Exploring ovarian cancer screening using a combined sensor approach: a pilot study. AIP Adv. (2020) 10:035213. doi: 10.1063/1.5144532
25. Rodríguez-Aguilar M, Díaz de León-Martínez L, Gorocica-Rosete P, Pérez-Padilla R, Domínguez-Reyes CA, Tenorio-Torres JA, et al. Application of chemoresistive gas sensors and chemometric analysis to differentiate the fingerprints of global volatile organic compounds from diseases. Preliminary results of COPD, lung cancer and breast cancer. Clin Chim Acta. (2021) 518:83–92. doi: 10.1016/j.cca.2021.03.016
26. van de Kant KDG, van der Sande LJTM, Jöbsis Q, van Schayck OCP, Dompeling E. Clinical use of exhaled volatile organic compounds in pulmonary diseases: a systematic review. Respir Res. (2012) 13:117. doi: 10.1186/1465-9921-13-117
27. Sethi S, Nanda R, Chakraborty T. Clinical application of volatile organic compound analysis for detecting infectious diseases. Clin Microbiol Rev. (2013) 26:462–75. doi: 10.1128/CMR.00020-13
28. Traxler S, Bischoff A-C, Saß R, Trefz P, Gierschner P, Brock B, et al. VOC breath profile in spontaneously breathing awake swine during influenza A infection. Sci Rep. (2018) 8:14857. doi: 10.1038/s41598-018-33061-2
29. Aksenov AA, Sandrock CE, Zhao W, Sankaran S, Schivo M, Harper R, et al. Cellular scent of influenza virus infection. Chembiochem. (2014) 15:1040–8. doi: 10.1002/cbic.201300695
30. Gould O, Ratcliffe N, Król E, de Lacy Costello B. Breath analysis for detection of viral infection, the current position of the field. J Breath Res. (2020) 14:041001. doi: 10.1088/1752-7163/ab9c32
31. Shen B, Yi X, Sun Y, Bi X, Du J, Zhang C, et al. Proteomic and metabolomic characterization of COVID-19 patient sera. Cell. (2020) 182:59–72. doi: 10.1016/j.cell.2020.05.032
32. Lamote K, Janssens E, Schillebeeckx E, Lapperre TS, De Winter BY, van Meerbeeck JP. The scent of COVID-19: viral (semi-)volatiles as fast diagnostic biomarkers? J Breath Res. (2020) 14:042001. doi: 10.1088/1752-7163/aba105
33. Ruszkiewicz DM, Sanders D, O’Brien R, Hempel F, Reed MJ, Riepe AC, et al. Diagnosis of COVID-19 by analysis of breath with gas chromatography-ion mobility spectrometry – A feasibility study. EClinicalMedicine. (2020) 2:100609. doi: 10.1016/j.eclinm.2020.100609
34. Berna AZ, Akaho EH, Harris RM, Congdon M, Korn E, Neher S, et al. Reproducible breath metabolite changes in children with SARS-CoV-2 infection. ACS Infect Dis. (2021) 7:2596–603. doi: 10.1021/acsinfecdis.1c00248
35. Chen H, Qi X, Zhang L, Li X, Ma J, Zhang C, et al. COVID-19 screening using breath-borne volatile organic compounds. J Breath Res. (2021) 15:047104. doi: 10.1088/1752-7163/ac2e57
36. Grassin-Delyle S, Roquencourt C, Moine P, Saffroy G, Carn S, Heming N, et al. Metabolomics of exhaled breath in critically ill COVID-19 patients: a pilot study. EBioMedicine. (2021) 63:103154. doi: 10.1016/j.ebiom.2020.103154
37. Ibrahim W, Cordell RL, Wilde MJ, Richardson M, Carr L, Sundari Devi Dasi A, et al. Diagnosis of COVID-19 by exhaled breath analysis using gas chromatography–mass spectrometry. ERJ Open Res. (2021) 7:00139–02021. doi: 10.1183/23120541.00139-2021
38. Marder D, Tzanani N, Baratz A, Drug E, Prihed H, Weiss S, et al. A multiple-method comparative study using GC–MS, AMDIS and in-house-built software for the detection and identification of “unknown” volatile organic compounds in breath. J Mass Spectrom. (2021) 56:e4782. doi: 10.1002/jms.4782
39. Feuerherd M, Sippel A-K, Erber J, Baumbach JI, Schmid RM, Protzer U, et al. A proof of concept study for the differentiation of SARS-CoV-2, hCoV-NL63, and IAV-H1N1 in vitro cultures using ion mobility spectrometry. Sci Rep. (2021) 11:20143. doi: 10.1038/s41598-021-99742-7
40. Steppert C, Steppert I, Sterlacci W, Bollinger T. Rapid detection of SARS-CoV-2 infection by multicapillary column coupled ion mobility spectrometry (MCC-IMS) of breath. A proof of concept study. J Breath Res. (2021) 15:027105. doi: 10.1088/1752-7163/abe5ca
41. ten Hagen NA, Twele F, Meller S, Jendrny P, Schulz C, von Köckritz-Blickwede M, et al. Discrimination of SARS-CoV-2 infections from other viral respiratory infections by scent detection dogs. Front Med. (2021) 8:749588. doi: 10.3389/fmed.2021.749588
42. Harper WJ. The strengths and weaknesses of the electronic nose. In: Rouseff R, Cadwallader K editors. Headspace Analysis of Foods and Flavors. Advances in Experimental Medicine and Biology. Boston, MA: Springer (2001). p. 59–71. doi: 10.1007/978-1-4615-1247-9_5
43. Zohora SE, Khan AM, Hundewale N. Chemical sensors employed in electronic noses: a review. In: Meghanathan N, Nagamalai D, Chaki N editors. Advances in Computing and Information Technology. Advances in Intelligent Systems and Computing. Berlin: Springer (2013). p. 177–84. doi: 10.1007/978-3-642-31600-5_18
44. Hu W, Wan L, Jian Y, Ren C, Jin K, Su X, et al. Electronic noses: from advanced materials to sensors aided with data processing. Adv Mater Technol. (2019) 4:1800488. doi: 10.1002/admt.201800488
45. Rodríguez-Aguilar M, Díaz de León-Martínez L, Zamora-Mendoza BN, Comas-García A, Guerra Palomares SE, García-Sepúlveda CA, et al. Comparative analysis of chemical breath-prints through olfactory technology for the discrimination between SARS-CoV-2 infected patients and controls. Clin Chim Acta. (2021) 519:126–32. doi: 10.1016/j.cca.2021.04.015
46. Wintjens AGWE, Hintzen KFH, Engelen SME, Lubbers T, Savelkoul PHM, Wesseling G, et al. Applying the electronic nose for pre-operative SARS-CoV-2 screening. Surg Endosc. (2021) 35:6671–8. doi: 10.1007/s00464-020-08169-0
47. Guest C, Dewhirst SY, Lindsay SW, Allen DJ, Aziz S, Baerenbold O, et al. Using trained dogs and organic semi-conducting sensors to identify asymptomatic and mild SARS-CoV-2 infections: an observational study. J Travel Med. (2022) 29:taac043. doi: 10.1093/jtm/taac043
48. Shan B, Broza YY, Li W, Wang Y, Wu S, Liu Z, et al. Multiplexed nanomaterial-based sensor array for detection of COVID-19 in exhaled breath. ACS Nano. (2020) 14:12125–32. doi: 10.1021/acsnano.0c05657
49. Zamora-Mendoza BN, Díaz de León-Martínez L, Rodríguez-Aguilar M, Mizaikoff B, Flores-Ramírez R. Chemometric analysis of the global pattern of volatile organic compounds in the exhaled breath of patients with COVID-19, post-COVID and healthy subjects. Proof of concept for post-COVID assessment. Talanta. (2022) 236:122832. doi: 10.1016/j.talanta.2021.122832
50. Snitz K, Andelman-Gur M, Pinchover L, Weissgross R, Weissbrod A, Mishor E, et al. Proof of concept for real-time detection of SARS CoV-2 infection with an electronic nose. PLoS One. (2021) 16:e0252121. doi: 10.1371/journal.pone.0252121
51. Grandjean D, Salmon D, Slama D, Gallet C, Julien C, Seyrat E, et al. Screening for SARS-CoV-2 persistence in long COVID patients using sniffer dogs and scents from axillary sweats samples. J Clin Trials. (2022) 12:1000002.
52. Twele F, ten Hagen NA, Meller S, Schulz C, Osterhaus A, Jendrny P, et al. Detection of post-COVID-19 patients using medical scent detection dogs—a pilot study. Front Med. (2022) 9:877259. doi: 10.3389/fmed.2022.877259
53. Vinaixa M, Schymanski EL, Neumann S, Navarro M, Salek RM, Yanes O. Mass spectral databases for LC/MS- and GC/MS-based metabolomics: state of the field and future prospects. Trends Anal Chem. (2016) 78:23–35. doi: 10.1016/j.trac.2015.09.005
54. Ligor M, Ligor T, Bajtarevic A, Ager C, Pienz M, Klieber M, et al. Determination of volatile organic compounds in exhaled breath of patients with lung cancer using solid phase microextraction and gas chromatography mass spectrometry. Clin Chem Lab Med. (2009) 47:550–60. doi: 10.1515/CCLM.2009.133
55. Ulanowska A, Kowalkowski T, Trawińska E, Buszewski B. The application of statistical methods using VOCs to identify patients with lung cancer. J Breath Res. (2011) 5:046008. doi: 10.1088/1752-7155/5/4/046008
56. van Oort P, de Bruin S, Weda H, Knobel H, Schultz M, Bos L. Exhaled breath metabolomics for the diagnosis of pneumonia in intubated and mechanically-ventilated intensive care unit (ICU)-patients. Int J Mol Sci. (2017) 18:449. doi: 10.3390/ijms18020449
57. Schleich FN, Zanella D, Stefanuto P-H, Bessonov K, Smolinska A, Dallinga JW, et al. Exhaled volatile organic compounds are able to discriminate between neutrophilic and eosinophilic asthma. Am J Respir Crit Care Med. (2019) 200:444–53. doi: 10.1164/rccm.201811-2210OC
58. James D, Scott SM, Ali Z, O’Hare WT. Chemical sensors for electronic nose systems. Microchim Acta. (2005) 149:1–17. doi: 10.1007/s00604-004-0291-6
59. Jalal AH, Alam F, Roychoudhury S, Umasankar Y, Pala N, Bhansali S. Prospects and challenges of volatile organic compound sensors in human healthcare. ACS Sens. (2018) 3:1246–63. doi: 10.1021/acssensors.8b00400
60. Phillips M, Herrera J, Krishnan S, Zain M, Greenberg J, Cataneo RN. Variation in volatile organic compounds in the breath of normal humans. J Chromatogr B Biomed Sci Appl. (1999) 729:75–88. doi: 10.1016/S0378-4347(99)00127-9
61. Walker DB, Walker JC, Cavnar PJ, Taylor JL, Pickel DH, Hall SB, et al. Naturalistic quantification of canine olfactory sensitivity. Appl Anim Behav Sci. (2006) 97:241–54. doi: 10.1016/j.applanim.2005.07.009
62. Craven BA, Paterson EG, Settles GS. The fluid dynamics of canine olfaction: unique nasal airflow patterns as an explanation of macrosmia. J R Soc Interface. (2010) 7:933–43. doi: 10.1098/rsif.2009.0490
63. Concha AR, Guest CM, Harris R, Pike TW, Feugier A, Zulch H, et al. Canine olfactory thresholds to amyl acetate in a biomedical detection scenario. Front Vet Sci. (2019) 5:345. doi: 10.3389/fvets.2018.00345
64. Schmidt K, Podmore I. Current challenges in volatile organic compounds analysis as potential biomarkers of cancer. J Biomark. (2015) 2015:981458. doi: 10.1155/2015/981458
65. Wojnowski W, Kalinowska K. Machine learning and electronic noses for medical diagnostics. In: Lidströmer N, Ashrafian H editors. Artificial Intelligence in Medicine. Cham: Springer International Publishing (2021). p. 1–17. doi: 10.1007/978-3-030-58080-3_329-1
66. D’Aniello B, Pinelli C, Varcamonti M, Rendine M, Lombardi P, Scandurra A. COVID sniffer dogs: technical and ethical concerns. Front Vet Sci. (2021) 8:669712. doi: 10.3389/fvets.2021.669712
67. Hackner K, Pleil J. Canine olfaction as an alternative to analytical instruments for disease diagnosis: understanding ‘dog personality’ to achieve reproducible results. J Breath Res. (2017) 11:012001. doi: 10.1088/1752-7163/aa5524
68. McGowan RTS, Rehn T, Norling Y, Keeling LJ. Positive affect and learning: exploring the “Eureka Effect” in dogs. Anim Cogn. (2014) 17:577–87. doi: 10.1007/s10071-013-0688-x
69. Kantele A, Paajanen J, Turunen S, Pakkanen SH, Patjas A, Itkonen L, et al. Scent dogs in detection of COVID-19: triple-blinded randomised trial and operational real-life screening in airport setting. BMJ Glob Health. (2022) 7:e008024. doi: 10.1136/bmjgh-2021-008024
70. Maurer M, Seto T, Guest C, Somal A, Julian C. Detection of SARS-CoV-2 by canine olfaction: a pilot study. Open Forum Infect Dis. (2022) 9:ofac226. doi: 10.1093/ofid/ofac226
71. ten Hagen NA, Twele F, Meller S, Wijnen L, Schulz C, Schoneberg C, et al. Canine real-time detection of SARS-CoV-2 infections in the context of a mass screening event. BMJ Glob Health. (2022) 7:e010276. doi: 10.1136/bmjgh-2022-010276
72. Bremhorst A, Bütler S, Würbel H, Riemer S. Incentive motivation in pet dogs – Preference for constant vs varied food rewards. Sci Rep. (2018) 8:9756. doi: 10.1038/s41598-018-28079-5
73. Gazit I, Terkel J. Explosives detection by sniffer dogs following strenuous physical activity. Appl Anim Behav Sci. (2003) 81:149–61. doi: 10.1016/S0168-1591(02)00274-5
74. Slensky KA, Drobatz KJ, Downend AB, Otto CM. Deployment morbidity among search-and-rescue dogs used after the September 11, 2001, terrorist attacks. J Am Vet Med Assoc. (2004) 225:868–73. doi: 10.2460/javma.2004.225.868
75. Wackermannová M, Pinc L, Jebavý L. Olfactory sensitivity in mammalian species. Physiol Res. (2016) 65:369–90. doi: 10.33549/physiolres.932955
76. Jamieson LTJ, Baxter GS, Murray PJ. Identifying suitable detection dogs. Appl Anim Behav Sci. (2017) 195:1–7. doi: 10.1016/j.applanim.2017.06.010
77. Kokocińska-Kusiak A, Woszczyło M, Zybala M, Maciocha J, Barłowska K, Dziêcioł M. Canine olfaction: physiology, behavior, and possibilities for practical applications. Animals. (2021) 11:2463. doi: 10.3390/ani11082463
78. Fleischer J, Breer H, Strotmann J. Mammalian olfactory receptors. Front Cell Neurosci. (2009) 3:9. doi: 10.3389/neuro.03.009.2009
79. Issel-Tarver L, Rine J. Organization and expression of canine olfactory receptor genes. Proc Natl Acad Sci U.S.A. (1996) 93:10897–902. doi: 10.1073/pnas.93.20.10897
80. Tacher S, Quignon P, Rimbault M, Dreano S, Andre C, Galibert F. Olfactory receptor sequence polymorphism within and between breeds of dogs. J Hered. (2005) 96:812–6. doi: 10.1093/jhered/esi113
81. Lesniak A, Walczak M, Jezierski T, Sacharczuk M, Gawkowski M, Jaszczak K. Canine olfactory receptor gene polymorphism and its relation to odor detection performance by sniffer dogs. J Hered. (2008) 99:518–27. doi: 10.1093/jhered/esn057
82. Robin S, Tacher S, Rimbault M, Vaysse A, Dréano S, André C, et al. Genetic diversity of canine olfactory receptors. BMC Genomics. (2009) 10:21. doi: 10.1186/1471-2164-10-21
83. Chen R, Irwin DM, Zhang Y-P. Differences in selection drive olfactory receptor genes in different directions in dogs and wolf. Mol Biol Evol. (2012) 29:3475–84. doi: 10.1093/molbev/mss153
84. Rauth-Widmann B. Your Dog’s Senses: Understand how he Perceives his World. München: Cadmos (2006). p. 111.
85. Polgár Z, Kinnunen M, Újváry D, Miklósi Á, Gácsi M. A test of canine olfactory capacity: comparing various dog breeds and wolves in a natural detection task. PLoS One. (2016) 11:e0154087. doi: 10.1371/journal.pone.0154087
86. Packer R, Hendricks A, Burn C. Do dog owners perceive the clinical signs related to conformational inherited disorders as “normal” for the breed? A potential constraint to improving canine welfare. Anim Welf. (2012) 21:81–93. doi: 10.7120/096272812X13345905673809
87. Bartels A, Martin V, Bidoli E, Steigmeier-Raith S, Brühschwein A, Reese S, et al. Brachycephalic problems of pugs relevant to animal welfare. Anim Welf. (2015) 24:327–33. doi: 10.7120/09627286.24.3.327
88. Oechtering TH, Oechtering GU, Noeller C. Computed tomographic imaging of the nose in brachycephalic dog breeds. Tierärztl Prax. (2007) 35:177–87.
89. Hall NJ, Glenn K, Smith DW, Wynne CDL. Performance of pugs, german shepherds, and greyhounds (Canis lupus familiaris) on an odor-discrimination task. J Comp Psychol. (2015) 129:237–46. doi: 10.1037/a0039271
90. Craven BA, Neuberger T, Paterson EG, Webb AG, Josephson EM, Morrison EE, et al. Reconstruction and morphometric analysis of the nasal airway of the dog (Canis familiaris) and implications regarding olfactory airflow. Anat Rec. (2007) 290:1325–40. doi: 10.1002/ar.20592
91. Negus VE. The Comparative Anatomy and Physiology of the Nose and Paranasal Sinuses. Edinburgh: Livingstone (1958). p. 402.
92. Wagner F, Ruf I. Who nose the borzoi? Turbinal skeleton in a dolichocephalic dog breed (Canis lupus familiaris). Mamm Biol. (2019) 94:106–19. doi: 10.1016/j.mambio.2018.06.005
93. Beebe SC, Howell TJ, Bennett PC. Using scent detection dogs in conservation settings: a review of scientific literature regarding their selection. Front Vet Sci. (2016) 3:96. doi: 10.3389/fvets.2016.00096
94. Cablk ME, Heaton JS. Accuracy and reliability of dogs in surveying for desert tortoise (Gopherus agassizii). Ecol Appl. (2006) 16:1926–35. doi: 10.1890/1051-0761(2006)016[1926:aarodi]2.0.co;2
95. Hurt A, Smith DA. Conservation dogs. In: Helton WS, editor. Canine Ergonomics: The Science of Working Dogs. Boca Raton, FL: CRC Press (2009). p. 175–94.
96. McGarrity ME, Sinn DL, Thomas SG, Marti CN, Gosling SD. Comparing the predictive validity of behavioral codings and behavioral ratings in a working-dog breeding program. Appl Anim Behav Sci. (2016) 179:82–94. doi: 10.1016/j.applanim.2016.03.013
97. Wilsson E, Sundgren P-E. The use of a behaviour test for the selection of dogs for service and breeding, I: method of testing and evaluating test results in the adult dog, demands on different kinds of service dogs, sex and breed differences. Appl Anim Behav Sci. (1997) 53:279–95. doi: 10.1016/S0168-1591(96)01174-4
98. Rebmann A, David E, Sorg MHH. Cadaver Dog Handbook: Forensic Training and Tactics for the Recovery of Human Remains. Boca Raton, FL: CRC Press (2000).
99. Rooney NJ, Bradshaw JWS. Breed and sex differences in the behavioural attributes of specialist search dogs—a questionnaire survey of trainers and handlers. Appl Anim Behav Sci. (2004) 86:123–35. doi: 10.1016/j.applanim.2003.12.007
100. Wei Q, Zhang H, Ma S, Guo D. Sex- and age-related differences in c-fos expression in dog olfactory bulbs. Acta Zool. (2017) 98:370–6. doi: 10.1111/azo.12178
101. Hart BL, Eckstein RA. The role of gonadal hormones in the occurrence of objectionable behaviours in dogs and cats. Appl Anim Behav Sci. (1997) 52:331–44. doi: 10.1016/S0168-1591(96)01133-1
102. Maejima M, Inoue-Murayama M, Tonosaki K, Matsuura N, Kato S, Saito Y, et al. Traits and genotypes may predict the successful training of drug detection dogs. Appl Anim Behav Sci. (2007) 107:287–98. doi: 10.1016/j.applanim.2006.10.005
103. Wells DL, Hepper PG. Directional tracking in the domestic dog, Canis familiaris. Appl Anim Behav Sci. (2003) 84:297–305. doi: 10.1016/j.applanim.2003.08.009
104. Myers LJ, Nusbaum KE, Swango LJ, Hanrahan LN, Sartin E. Dysfunction of sense of smell caused by canine parainfluenza virus infection in dogs. Am J Vet Res. (1988) 49:188–90.
105. Myers LJ. Dysosmia of the dog in clinical veterinary medicine. Prog Vet Neurol. (1990) 1:171–9.
106. Butowt R, Bilinska K. SARS-CoV-2: olfaction, brain infection, and the urgent need for clinical samples allowing earlier virus detection. ACS Chem Neurosci. (2020) 11:1200–3. doi: 10.1021/acschemneuro.0c00172
107. Bryche B, St Albin A, Murri S, Lacôte S, Pulido C, Ar Gouilh M, et al. Massive transient damage of the olfactory epithelium associated with infection of sustentacular cells by SARS-CoV-2 in golden Syrian hamsters. Brain Behav Immun. (2020) 89:579–86. doi: 10.1016/j.bbi.2020.06.032
108. Ye Q, Zhou J, He Q, Li R-T, Yang G, Zhang Y, et al. SARS-CoV-2 infection in the mouse olfactory system. Cell Discov. (2021) 7:49. doi: 10.1038/s41421-021-00290-1
109. Calvet GA, Pereira SA, Ogrzewalska M, Pauvolid-Corrêa A, Resende PC, de Tassinari WS, et al. Investigation of SARS-CoV-2 infection in dogs and cats of humans diagnosed with COVID-19 in Rio de Janeiro, Brazil. PLoS One. (2021) 16:e0250853. doi: 10.1371/journal.pone.0250853
110. Hamer SA, Pauvolid-Corrêa A, Zecca IB, Davila E, Auckland LD, Roundy CM, et al. SARS-CoV-2 infections and viral isolations among serially tested cats and dogs in households with infected owners in Texas, USA. Viruses. (2021) 13:938. doi: 10.3390/v13050938
111. Shi J, Wen Z, Zhong G, Yang H, Wang C, Huang B, et al. Susceptibility of ferrets, cats, dogs, and other domesticated animals to SARS–coronavirus 2. Science. (2020) 368:1016–20. doi: 10.1126/science.abb7015
112. Hirai T, Kojima S, Shimada A, Umemura T, Sakai M, Itakurat C. Age-related changes in the olfactory system of dogs. Neuropathol Appl Neurobiol. (1996) 22:531–9. doi: 10.1111/j.1365-2990.1996.tb01132.x
113. Salvin HE, McGreevy PD, Sachdev PS, Valenzuela MJ. The canine cognitive dysfunction rating scale (CCDR): a data-driven and ecologically relevant assessment tool. Vet J. (2011) 188:331–6. doi: 10.1016/j.tvjl.2010.05.014
114. Schütt T, Toft N, Berendt M. Cognitive function, progression of age-related behavioral changes, biomarkers, and survival in dogs more than 8 years old. J Vet Intern Med. (2015) 29:1569–77. doi: 10.1111/jvim.13633
115. Ozawa M, Inoue M, Uchida K, Chambers JK, Takeuch Y, Nakayama H. Physical signs of canine cognitive dysfunction. J Vet Med Sci. (2019) 81:1829–34. doi: 10.1292/jvms.19-0458
116. Kjelvik G, Saltvedt I, White LR, Stenumgård P, Sletvold O, Engedal K, et al. The brain structural and cognitive basis of odor identification deficits in mild cognitive impairment and Alzheimer’s disease. BMC Neurol. (2014) 14:168. doi: 10.1186/s12883-014-0168-1
117. Chaber A, Hazel S, Matthews B, Withers A, Alvergnat G, Grandjean D, et al. Evaluation of canine detection of COVID-19 infected individuals under controlled settings. Transbound Emerg Dis. (2022) 69:e1951–8. doi: 10.1111/tbed.14529
118. Vesga O, Agudelo M, Valencia-Jaramillo AF, Mira-Montoya A, Ossa-Ospina F, Ocampo E, et al. Highly sensitive scent-detection of COVID-19 patients in vitro by trained dogs. PLoS One. (2021) 16:e0257474. doi: 10.1371/journal.pone.0257474
119. Horowitz A, Hecht J, Dedrick A. Smelling more or less: investigating the olfactory experience of the domestic dog. Learn Motiv. (2013) 44:207–17. doi: 10.1016/j.lmot.2013.02.002
120. Jenkins EK, DeChant MT, Perry EB. When the nose doesn’t know: canine olfactory function associated with health, management, and potential links to microbiota. Front Vet Sci. (2018) 5:56. doi: 10.3389/fvets.2018.00056
121. Bräuer J, Blasi D. Dogs display owner-specific expectations based on olfaction. Sci Rep. (2021) 11:3291. doi: 10.1038/s41598-021-82952-4
122. Otto CM, Hare E, Nord JL, Palermo SM, Kelsey KM, Darling TA, et al. Evaluation of three hydration strategies in detection dogs working in a hot environment. Front Vet Sci. (2017) 4:174. doi: 10.3389/fvets.2017.00174
123. Jezierski T, Adamkiewicz E, Walczak M, Sobczyńska M, Górecka-Bruzda A, Ensminger J, et al. Efficacy of drug detection by fully-trained police dogs varies by breed, training level, type of drug and search environment. Forensic Sci Int. (2014) 237:112–8. doi: 10.1016/j.forsciint.2014.01.013
124. Lazarowski L, Haney PS, Brock J, Fischer T, Rogers B, Angle C, et al. Investigation of the behavioral characteristics of dogs purpose-bred and prepared to perform vapor wake® detection of person-borne explosives. Front Vet Sci. (2018) 5:50. doi: 10.3389/fvets.2018.00050
125. Angle CT, Wakshlag JJ, Gillette RL, Steury T, Haney P, Barrett J, et al. The effects of exercise and diet on olfactory capability in detection dogs. J Nutr Sci. (2014) 3:e44. doi: 10.1017/jns.2014.35
126. Altom EK, Davenport GM, Myers LJ, Cummins KA. Effect of dietary fat source and exercise on odorant-detecting ability of canine athletes. Res Vet Sci. (2003) 75:149–55. doi: 10.1016/S0034-5288(03)00071-7
127. Jenkins EK, Lee-Fowler TM, Angle TC, Behrend EN, Moore GE. Effects of oral administration of metronidazole and doxycycline on olfactory capabilities of explosives detection dogs. Am J Vet Res. (2016) 77:906–12. doi: 10.2460/ajvr.77.8.906
128. Ezeh PI, Myers LJ, Hanrahan LA, Kemppainen RJ, Cummins KA. Effects of steroids on the olfactory function of the dog. Physiol Behav. (1992) 51:1183–7. doi: 10.1016/0031-9384(92)90306-M
129. Moser AY, Bizo L, Brown WY. Olfactory generalization in detector dogs. Animals. (2019) 9:702. doi: 10.3390/ani9090702
130. Reeve C, Koivusalo M. Biomedical scent detection dogs: would they pass as a health technology? Pet Behav Sci. (2018) 6:1–7. doi: 10.21071/pbs.v0i6.10785
131. Waggoner P, Lazarowski L, Hutchings B, Angle C, Porritt F. Effects of learning an increasing number of odors on olfactory learning, memory and generalization in detection dogs. Appl Anim Behav Sci. (2022) 247:105568. doi: 10.1016/j.applanim.2022.105568
132. Lit L, Schweitzer JB, Oberbauer AM. Handler beliefs affect scent detection dog outcomes. Anim Cogn. (2011) 14:387–94. doi: 10.1007/s10071-010-0373-2
133. Jendrny P, Schulz C, Twele F, Meller S, Von Köckritz-Blickwede M, Osterhaus ADME, et al. Scent dog identification of samples from COVID-19 patients – A pilot study. BMC Infect Dis. (2020) 20:536. doi: 10.1186/s12879-020-05281-3
134. Jendrny P, Twele F, Meller S, Schulz C, Von Köckritz-Blickwede M, Osterhaus ADME, et al. Scent dog identification of SARS-CoV-2 infections in different body fluids. BMC Infect Dis. (2021) 21:707. doi: 10.1186/s12879-021-06411-1
135. Aviles-Rosa EO, Gallegos SF, Prada-Tiedemann PA, Hall NJ. An automated canine line-up for detection dog research. Front Vet Sci. (2021) 8:775381. doi: 10.3389/fvets.2021.775381
136. Bosco-Lauth AM, Hartwig AE, Porter SM, Gordy PW, Nehring M, Byas AD, et al. Experimental infection of domestic dogs and cats with SARS-CoV-2: pathogenesis, transmission, and response to reexposure in cats. Proc Natl Acad Sci U.S.A. (2020) 117:26382–8. doi: 10.1073/pnas.2013102117
137. Patterson EI, Elia G, Grassi A, Giordano A, Desario C, Medardo M, et al. Evidence of exposure to SARS-CoV-2 in cats and dogs from households in Italy. Nat Commun. (2020) 11:6231. doi: 10.1038/s41467-020-20097-0
138. Sit THC, Brackman CJ, Ip SM, Tam KWS, Law PYT, To EMW, et al. Infection of dogs with SARS-CoV-2. Nature. (2020) 586:776–8. doi: 10.1038/s41586-020-2334-5
139. Decaro N, Vaccari G, Lorusso A, Lorusso E, De Sabato L, Patterson EI, et al. Possible human-to-dog transmission of SARS-CoV-2. Italy, 2020. Emerg Infect Dis. (2021) 27:1981–4. doi: 10.3201/eid2707.204959
140. Barroso R, Vieira-Pires A, Antunes A, Fidalgo-Carvalho I. Susceptibility of pets to SARS-CoV-2 infection: lessons from a seroepidemiologic survey of cats and dogs in Portugal. Microorganisms. (2022) 10:345. doi: 10.3390/microorganisms10020345
141. Ferasin L, Fritz M, Ferasin H, Becquart P, Corbet S, Ar Gouilh M, et al. Infection with SARS-CoV-2 variant B.1.1.7 detected in a group of dogs and cats with suspected myocarditis. Vet Rec. (2021) 189:e944. doi: 10.1002/vetr.944
142. Schulz C, Martina B, Mirolo M, Müller E, Klein R, Volk H, et al. SARS-CoV-2–specific antibodies in domestic cats during first COVID-19 wave, Europe. Emerg Infect Dis. (2021) 27:3115–8. doi: 10.3201/eid2712.211252
143. Schulz C, Wylezich C, Wernike K, Gründl M, Dangel A, Baechlein C, et al. Prolonged SARS-CoV-2 RNA shedding from therapy cat after cluster outbreak in retirement home. Emerg Infect Dis. (2021) 27:1974–6. doi: 10.3201/eid2707.204670
144. Eskandari E, Ahmadi Marzaleh M, Roudgari H, Hamidi Farahani R, Nezami-Asl A, Laripour R, et al. Sniffer dogs as a screening/diagnostic tool for COVID-19: a proof of concept study. BMC Infect Dis. (2021) 21:243. doi: 10.1186/s12879-021-05939-6
145. Essler JL, Kane SA, Nolan P, Akaho EH, Berna AZ, DeAngelo A, et al. Discrimination of SARS-CoV-2 infected patient samples by detection dogs: a proof of concept study. PLoS One. (2021) 16:e0250158. doi: 10.1371/journal.pone.0250158
146. Mendel J, Frank K, Edlin L, Hall K, Webb D, Mills J, et al. Preliminary accuracy of COVID-19 odor detection by canines and HS-SPME-GC-MS using exhaled breath samples. Forensic Sci Int Synerg. (2021) 3:100155. doi: 10.1016/j.fsisyn.2021.100155
147. Salgirli Y, Kismali G, Saral B, Sareyyüpoğlu B, Habiloğlu AD, Öztürk H, et al. Development of a Safety Protocol for Training and Using SARS-CoV-2 Detection Dogs. J Vet Behav [Preprint]. (2022). Available online at: https://papers.ssrn.com/sol3/papers.cfm?abstract_id=4075240# (accessed July 6, 2022).
148. Hong KH, Lee SW, Kim TS, Huh HJ, Lee J, Kim SY, et al. Guidelines for laboratory diagnosis of coronavirus disease 2019 (COVID-19) in Korea. Ann Lab Med. (2020) 40:351–60. doi: 10.3343/alm.2020.40.5.351
149. Hsieh W-Y, Lin C-H, Lin T-C, Lin C-H, Chang H-F, Tsai C-H, et al. Development and efficacy of lateral flow point-of-care testing devices for rapid and mass COVID-19 diagnosis by the detections of SARS-CoV-2 antigen and anti-SARS-CoV-2 antibodies. Diagnostics. (2021) 11:1760. doi: 10.3390/diagnostics11101760
150. Mohammadi A, Esmaeilzadeh E, Li Y, Bosch RJ, Li JZ. SARS-CoV-2 detection in different respiratory sites: a systematic review and meta-analysis. EBioMedicine. (2020) 59:102903. doi: 10.1016/j.ebiom.2020.102903
151. Yoon JG, Yoon J, Song JY, Yoon S-Y, Lim CS, Seong H, et al. Clinical significance of a high SARS-CoV-2 viral load in the saliva. J Korean Med Sci. (2020) 35:e195. doi: 10.3346/jkms.2020.35.e195
152. Medeiros da Silva RC, Nogueira Marinho LC, de Araújo Silva DN, Costa de Lima K, Pirih FQ, Luz de Aquino Martins AR. Saliva as a possible tool for the SARS-CoV-2 detection: a review. Travel Med Infect Dis. (2020) 38:101920. doi: 10.1016/j.tmaid.2020.101920
153. Jeong HW, Kim S-M, Kim H-S, Kim Y-I, Kim JH, Cho JY, et al. Viable SARS-CoV-2 in various specimens from COVID-19 patients. Clin Microbiol Infect. (2020) 26:1520–4. doi: 10.1016/j.cmi.2020.07.020
154. Huang N, Pérez P, Kato T, Mikami Y, Okuda K, Gilmore RC, et al. SARS-CoV-2 infection of the oral cavity and saliva. Nat Med. (2021) 27:892–903. doi: 10.1038/s41591-021-01296-8
155. Liu L, Wei Q, Alvarez X, Wang H, Du Y, Zhu H, et al. Epithelial cells lining salivary gland ducts are early target cells of severe acute respiratory syndrome coronavirus infection in the upper respiratory tracts of rhesus macaques. J Virol. (2011) 85:4025–30. doi: 10.1128/JVI.02292-10
156. Chen L, Zhao J, Peng J, Li X, Deng X, Geng Z, et al. Detection of SARS-CoV-2 in saliva and characterization of oral symptoms in COVID-19 patients. Cell Prolif. (2020) 53:e12923. doi: 10.1111/cpr.12923
157. Xu R, Cui B, Duan X, Zhang P, Zhou X, Yuan Q. Saliva: potential diagnostic value and transmission of 2019-nCoV. Int J Oral Sci. (2020) 12:11. doi: 10.1038/s41368-020-0080-z
158. Han MS, Seong M-W, Kim N, Shin S, Cho SI, Park H, et al. Viral RNA load in mildly symptomatic and asymptomatic children with COVID-19, Seoul, South Korea. Emerg Infect Dis. (2020) 26:2497–9. doi: 10.3201/eid2610.202449
159. Iwasaki S, Fujisawa S, Nakakubo S, Kamada K, Yamashita Y, Fukumoto T, et al. Comparison of SARS-CoV-2 detection in nasopharyngeal swab and saliva. J Infect. (2020) 81:e145–7. doi: 10.1016/j.jinf.2020.05.071
160. Kim SE, Lee JY, Lee A, Kim S, Park K-H, Jung S-I, et al. Viral load kinetics of SARS-CoV-2 infection in saliva in Korean patients: a prospective multi-center comparative study. J Korean Med Sci. (2020) 35:e287. doi: 10.3346/jkms.2020.35.e287
161. Procop GW, Shrestha NK, Vogel S, Van Sickle K, Harrington S, Rhoads DD, et al. A direct comparison of enhanced saliva to nasopharyngeal swab for the detection of SARS-CoV-2 in symptomatic patients. J Clin Microbiol. (2020) 58:e01946–20. doi: 10.1128/JCM.01946-20
162. To KK, Tsang OT-Y, Leung W, Tam AR, Wu T, Lung DC, et al. Temporal profiles of viral load in posterior oropharyngeal saliva samples and serum antibody responses during infection by SARS-CoV-2: an observational cohort study. Lancet Infect Dis. (2020) 20:565–74. doi: 10.1016/S1473-3099(20)30196-1
163. Williams E, Bond K, Zhang B, Putland M, Williamson DA. Saliva as a noninvasive specimen for detection of SARS-CoV-2. J Clin Microbiol. (2020) 58:e00776–20. doi: 10.1128/JCM.00776-20
164. Savela ES, Viloria Winnett A, Romano AE, Porter MK, Shelby N, Akana R, et al. Quantitative SARS-CoV-2 viral-load curves in paired saliva samples and nasal swabs inform appropriate respiratory sampling site and analytical test sensitivity required for earliest viral detection. J Clin Microbiol. (2022) 60:e0178521. doi: 10.1128/JCM.01785-21
165. Wyllie AL, Fournier J, Casanovas-Massana A, Campbell M, Tokuyama M, Vijayakumar P, et al. Saliva or nasopharyngeal swab specimens for detection of SARS-CoV-2. N Engl J Med. (2020) 383:1283–6. doi: 10.1056/NEJMc2016359
166. Kojima N, Turner F, Slepnev V, Bacelar A, Deming L, Kodeboyina S, et al. Self-collected oral fluid and nasal swabs demonstrate comparable sensitivity to clinician collected nasopharyngeal swabs for coronavirus disease 2019 detection. Clin Infect Dis. (2021) 73:e3106–9. doi: 10.1093/cid/ciaa1589
167. Pasomsub E, Watcharananan SP, Boonyawat K, Janchompoo P, Wongtabtim G, Suksuwan W, et al. Saliva sample as a non-invasive specimen for the diagnosis of coronavirus disease 2019: a cross-sectional study. Clin Microbiol Infect. (2021) 27:285.e1–4. doi: 10.1016/j.cmi.2020.05.001
168. Zhu J, Guo J, Xu Y, Chen X. Viral dynamics of SARS-CoV-2 in saliva from infected patients. J Infect. (2020) 81:e48–50. doi: 10.1016/j.jinf.2020.06.059
169. Penn DJ, Oberzaucher E, Grammer K, Fischer G, Soini HA, Wiesler D, et al. Individual and gender fingerprints in human body odour. J R Soc Interface. (2007) 4:331–40. doi: 10.1098/rsif.2006.0182
170. Soini HA, Klouckova I, Wiesler D, Oberzaucher E, Grammer K, Dixon SJ, et al. Analysis of volatile organic compounds in human saliva by a static sorptive extraction method and gas chromatography-mass spectrometry. J Chem Ecol. (2010) 36:1035–42. doi: 10.1007/s10886-010-9846-7
171. Wong DTW. Salivaomics. J Am Dent Assoc. (2012) 143:19S–24S. doi: 10.14219/jada.archive.2012.0339
172. Propper RE. Is sweat a possible route of transmission of SARS-CoV-2? Exp Biol Med. (2020) 245:997–8. doi: 10.1177/1535370220935409
173. Hamming I, Timens W, Bulthuis M, Lely A, Navis G, van Goor H. Tissue distribution of ACE2 protein, the functional receptor for SARS coronavirus. A first step in understanding SARS pathogenesis. J Pathol. (2004) 203:631–7. doi: 10.1002/path.1570
174. Saponaro F, Rutigliano G, Sestito S, Bandini L, Storti B, Bizzarri R, et al. ACE2 in the era of SARS-CoV-2: controversies and novel perspectives. Front Mol Biosci. (2020) 7:588618. doi: 10.3389/fmolb.2020.588618
175. Liu J, Li Y, Liu L, Hu X, Wang X, Hu H, et al. Infection of human sweat glands by SARS-CoV-2. Cell Discov. (2020) 6:84. doi: 10.1038/s41421-020-00229-y
176. Santonja C, Heras F, Núñez L, Requena L. COVID-19 chilblain-like lesion: immunohistochemical demonstration of SARS-CoV-2 spike protein in blood vessel endothelium and sweat gland epithelium in a polymerase chain reaction-negative patient. Br J Dermatol. (2020) 183:778–80. doi: 10.1111/bjd.19338
177. Bian X-W, Yao X-H, Ping Y-F, Yu S, Shi Y, Luo T, et al. Autopsy of COVID-19 patients in China. Natl Sci Rev. (2020) 7:1414–8. doi: 10.1093/nsr/nwaa123
178. Jamiolkowski D, Mühleisen B, Müller S, Navarini AA, Tzankov A, Roider E. SARS-CoV-2 PCR testing of skin for COVID-19 diagnostics: a case report. Lancet. (2020) 396:598–9. doi: 10.1016/S0140-6736(20)31754-2
179. Recalcati S, Tonolo S, Meroni E, Fantini F. SARS-CoV-2 in the sweat of COVID-19-positive patients: a possible route of transmission? J Eur Acad Dermatology Venereol. (2021) 35:e865–6. doi: 10.1111/jdv.17607
180. Arslan B, Bercin S, Aydogan S, Islamoglu Y, Dinc B. SARS-CoV-2 is not found in the sweat of COVID-19 positive patients. Irish J Med Sci. (2022) 191:27–9. doi: 10.1007/s11845-021-02537-y
181. Fathizadeh H, Taghizadeh S, Safari R, Khiabani SS, Babak B, Hamzavi F, et al. Study presence of COVID-19 (SARS-CoV-2) in the sweat of patients infected with COVID-19. Microb Pathog. (2020) 149:104556. doi: 10.1016/j.micpath.2020.104556
182. Munk S, Münch P, Stahnke L, Adler-Nissen J, Schieberle P. Primary odorants of laundry soiled with sweat/sebum: influence of lipase on the odor profile. J Surfactants Deterg. (2000) 3:505–15. doi: 10.1007/s11743-000-0150-z
183. Jadoon S, Karim S, Akram MR, Kalsoom Khan A, Zia MA, Siddiqi AR, et al. Recent developments in sweat analysis and its applications. Int J Anal Chem. (2015) 2015:164974. doi: 10.1155/2015/164974
184. Mancilla-Tapia JM, Lozano-Esparza V, Orduña A, Osuna-Chávez RF, Robles-Zepeda RE, Maldonado-Cabrera B, et al. Dogs detecting COVID-19 from sweat and saliva of positive people: a field experience in Mexico. Front Med. (2022) 9:837053. doi: 10.3389/fmed.2022.837053
185. Grandjean D, Sarkis R, Lecoq-Julien C, Benard A, Roger V, Levesque E, et al. Can the detection dog alert on COVID-19 positive persons by sniffing axillary sweat samples? A proof-of-concept study. PLoS One. (2020) 15:e0243122. doi: 10.1371/journal.pone.0243122
186. Angeletti S, Travaglino F, Spoto S, Pascarella MC, Mansi G, De Cesaris M, et al. COVID-19 sniffer dog experimental training: which protocol and which implications for reliable sidentification? J Med Virol. (2021) 93:5924–30. doi: 10.1002/jmv.27147
187. Grandjean D, Al Marzooqi DH, Lecoq-Julien C, Muzzin Q, Al Hammadi HK, Alvergnat G, et al. Use of canine olfactory detection for COVID-19 testing study on U.A.E. trained detection dog sensitivity. Open Access J Vet Sci Res. (2021) 6:000210. doi: 10.23880/oajvsr-16000210
188. de Maia RCC, Alves LC, da Silva JES, Czyba FR, Pereira JA, Soistier V, et al. Canine olfactory detection of SARS-COV2-infected patients: a one health approach. Front Public Health. (2021) 9:647903. doi: 10.3389/fpubh.2021.647903
189. Vlachová L, Hotový G, Šlechta J, Váňa R, Vokřálová M, Zeman J. Olfactory detection of human odorant signatures in COVID patients by trained dogs. medRxiv [Preprint]. (2021). doi: 10.1101/2021.07.12.21258827
190. Devillier P, Gallet C, Salvator H, Lecoq-Julien C, Naline E, Roisse D, et al. Biomedical detection dogs for the identification of SARS-CoV-2 infections from axillary sweat and breath samples. J Breath Res. (2022) 16:037101. doi: 10.1088/1752-7163/ac5d8c
191. Grandjean D, Elie C, Gallet C, Julien C, Roger V, Desquilbet L, et al. Diagnostic accuracy of non-invasive detection of SARS-CoV-2 infection by canine olfaction. PLoS One. (2022) 17:e0268382. doi: 10.1371/journal.pone.0268382
192. Grandjean D, Gallet C, Julien C, Sarkis R, Muzzin Q, Roger V, et al. Identifying SARS-COV-2 infected patients through canine olfactive detection on axillary sweat samples; study of observed sensitivities and specificities within a group of trained dogs. PLoS One. (2022) 17:e0262631. doi: 10.1371/journal.pone.0262631
193. Gokool VA, Crespo-Cajigas J, Mallikarjun A, Collins A, Kane S, Plymouth V, et al. The use of biological sensors and instrumental analysis to discriminate COVID-19 odor signatures. Biosensors. (2022) 12:1003. doi: 10.3390/bios12111003
194. Hag-Ali M, AlShamsi AS, Boeijen L, Mahmmod Y, Manzoor R, Rutten H, et al. The detection dogs test is more sensitive than real-time PCR in screening for SARS-CoV-2. Commun Biol. (2021) 4:686. doi: 10.1038/s42003-021-02232-9
195. Kim JY, Ko J-H, Kim Y, Kim Y-J, Kim J-M, Chung Y-S, et al. Viral load kinetics of SARS-CoV-2 infection in first two patients in Korea. J Korean Med Sci. (2020) 35:e86. doi: 10.3346/jkms.2020.35.e86
196. Sun J, Zhu A, Li H, Zheng K, Zhuang Z, Chen Z, et al. Isolation of infectious SARS-CoV-2 from urine of a COVID-19 patient. Emerg Microbes Infect. (2020) 9:991–3. doi: 10.1080/22221751.2020.1760144
197. Peng L, Liu J, Xu W, Luo Q, Chen D, Lei Z, et al. SARS-CoV-2 can be detected in urine, blood, anal swabs, and oropharyngeal swabs specimens. J Med Virol. (2020) 92:1676–80. doi: 10.1002/jmv.25936
198. Lo IL, Lio CF, Cheong HH, Lei CI, Cheong TH, Zhong X, et al. Evaluation of SARS-CoV-2 RNA shedding in clinical specimens and clinical characteristics of 10 patients with COVID-19 in Macau. Int J Biol Sci. (2020) 16:1698–707. doi: 10.7150/ijbs.45357
199. Bwire GM, Majigo MV, Njiro BJ, Mawazo A. Detection profile of SARS-CoV-2 using RT-PCR in different types of clinical specimens: a systematic review and meta-analysis. J Med Virol. (2021) 93:719–25. doi: 10.1002/jmv.26349
200. Jones DL, Baluja MQ, Graham DW, Corbishley A, McDonald JE, Malham SK, et al. Shedding of SARS-CoV-2 in feces and urine and its potential role in person-to-person transmission and the environment-based spread of COVID-19. Sci Total Environ. (2020) 749:141364. doi: 10.1016/j.scitotenv.2020.141364
201. Kashi AH, De la Rosette J, Amini E, Abdi H, Fallah-Karkan M, Vaezjalali M. Urinary viral shedding of COVID-19 and its clinical associations: a systematic review and meta-analysis of observational studies. Urol J. (2020) 17:433–41. doi: 10.22037/uj.v16i7.6248
202. Nomoto H, Ishikane M, Katagiri D, Kinoshita N, Nagashima M, Sadamasu K, et al. Cautious handling of urine from moderate to severe COVID-19 patients. Am J Infect Control. (2020) 48:969–71. doi: 10.1016/j.ajic.2020.05.034
203. Roshandel MR, Nateqi M, Lak R, Aavani P, Sari Motlagh R, Shariat SF, et al. Diagnostic and methodological evaluation of studies on the urinary shedding of SARS-CoV-2, compared to stool and serum: a systematic review and meta-analysis. Cell Mol Biol. (2020) 66:148–56. doi: 10.14715/cmb/2020.66.6.26
204. Joukar F, Yaghubi Kalurazi T, Khoshsorour M, Taramian S, Mahfoozi L, Balou HA, et al. Persistence of SARS-CoV-2 RNA in the nasopharyngeal, blood, urine, and stool samples of patients with COVID-19: a hospital-based longitudinal study. Virol J. (2021) 18:134. doi: 10.1186/s12985-021-01599-9
205. Ren X, Wang S, Chen X, Wei X, Li G, Ren S, et al. Multiple expression assessments of ACE2 and TMPRSS2 SARS-CoV-2 entry molecules in the urinary tract and their associations with clinical manifestations of COVID-19. Infect Drug Resist. (2020) 13:3977–90. doi: 10.2147/IDR.S270543
206. Martinez-Rojas MA, Vega-Vega O, Bobadilla NA. Is the kidney a target of SARS-CoV-2? Am J Physiol Physiol. (2020) 318:F1454–62. doi: 10.1152/ajprenal.00160.2020
207. George S, Pal AC, Gagnon J, Timalsina S, Singh P, Vydyam P, et al. Evidence for SARS-CoV-2 spike protein in the urine of COVID-19 patients. Kidney. (2021) 2:924–36. doi: 10.34067/KID.0002172021
208. Amann A, Costello BDL, Miekisch W, Schubert J, Buszewski B, Pleil J, et al. The human volatilome: volatile organic compounds (VOCs) in exhaled breath, skin emanations, urine, feces and saliva. J Breath Res. (2014) 8:034001. doi: 10.1088/1752-7155/8/3/034001
209. Banday KM, Pasikanti KK, Chan ECY, Singla R, Rao KVS, Chauhan VS, et al. Use of urine volatile organic compounds to discriminate tuberculosis patients from healthy subjects. Anal Chem. (2011) 83:5526–34. doi: 10.1021/ac200265g
210. Lim SH, Martino R, Anikst V, Xu Z, Mix S, Benjamin R, et al. Rapid diagnosis of tuberculosis from analysis of urine volatile organic compounds. ACS Sens. (2016) 1:852–6. doi: 10.1021/acssensors.6b00309
211. Janfaza S, Khorsand B, Nikkhah M, Zahiri J. Digging deeper into volatile organic compounds associated with cancer. Biol Methods Protoc. (2019) 4:bz014. doi: 10.1093/biomethods/bpz014
212. Duguid JP. The size and the duration of air-carriage of respiratory droplets and droplet-nuclei. Epidemiol Infect. (1946) 44:471–9. doi: 10.1017/S0022172400019288
213. Fairchild CI, Stampfer JF. Particle concentration in exhaled breath. Am Ind Hyg Assoc J. (1987) 48:948–9. doi: 10.1080/15298668791385868
214. Papineni RS, Rosenthal FS. The size distribution of droplets in the exhaled breath of healthy human subjects. J Aerosol Med. (1997) 10:105–16. doi: 10.1089/jam.1997.10.105
215. Miekisch W, Schubert JK, Noeldge-Schomburg GFE. Diagnostic potential of breath analysis—focus on volatile organic compounds. Clin Chim Acta. (2004) 347:25–39. doi: 10.1016/j.cccn.2004.04.023
216. Bourouiba L, Dehandschoewercker E, Bush JWM. Violent expiratory events: on coughing and sneezing. J Fluid Mech. (2014) 745:537–63. doi: 10.1017/jfm.2014.88
217. Asadi S, Bouvier N, Wexler AS, Ristenpart WD. The coronavirus pandemic and aerosols: does COVID-19 transmit via expiratory particles? Aerosol Sci Technol. (2020) 54:635–8. doi: 10.1080/02786826.2020.1749229
218. Scheuch G. Breathing is enough: for the spread of influenza virus and SARS-CoV-2 by breathing only. J Aerosol Med Pulm Drug Deliv. (2020) 33:230–4. doi: 10.1089/jamp.2020.1616
219. Ghosh C, Singh V, Grandy J, Pawliszyn J. Recent advances in breath analysis to track human health by new enrichment technologies. J Sep Sci. (2020) 43:226–40. doi: 10.1002/jssc.201900769
220. Lomonaco T, Salvo P, Ghimenti S, Biagini D, Vivaldi F, Bonini A, et al. Stability of volatile organic compounds in sorbent tubes following SARS-CoV-2 inactivation procedures. J Breath Res. (2021) 15:037102. doi: 10.1088/1752-7163/abf0b4
221. Kang S, Paul Thomas CL. How long may a breath sample be stored for at –80 °C? A study of the stability of volatile organic compounds trapped onto a mixed Tenax: carbograph trap adsorbent bed from exhaled breath. J Breath Res. (2016) 10:026011. doi: 10.1088/1752-7155/10/2/026011
222. Harshman SW, Mani N, Geier BA, Kwak J, Shepard P, Fan M, et al. Storage stability of exhaled breath on Tenax TA. J Breath Res. (2016) 10:046008. doi: 10.1088/1752-7155/10/4/046008
223. Oakley-Girvan I, Davis SW. Breath based volatile organic compounds in the detection of breast, lung, and colorectal cancers: a systematic review. Cancer Biomark. (2017) 21:29–39. doi: 10.3233/CBM-170177
224. Salman D, Ibrahim W, Kanabar A, Joyce A, Zhao B, Singapuri A, et al. The variability of volatile organic compounds in the indoor air of clinical environments. J Breath Res. (2022) 16:016005. doi: 10.1088/1752-7163/ac3565
225. Amal H, Leja M, Broza YY, Tisch U, Funka K, Liepniece-Karele I, et al. Geographical variation in the exhaled volatile organic compounds. J Breath Res. (2013) 7:047102. doi: 10.1088/1752-7155/7/4/047102
226. Elliker KR, Sommerville BA, Broom DM, Neal DE, Armstrong S, Williams HC. Key considerations for the experimental training and evaluation of cancer odour detection dogs: lessons learnt from a double-blind, controlled trial of prostate cancer detection. BMC Urol. (2014) 14:22. doi: 10.1186/1471-2490-14-22
227. Guest CM, Harris R, Anjum I, Concha AR, Rooney NJ. A lesson in standardization – Subtle aspects of the processing of samples can greatly affect dogs’ learning. Front Vet Sci. (2020) 7:525. doi: 10.3389/fvets.2020.00525
228. DeChant MT, Bunker PC, Hall NJ. Stimulus control of odorant concentration: pilot study of generalization and discrimination of odor concentration in canines. Animals. (2021) 11:326. doi: 10.3390/ani11020326
229. Wilson C, Morant S, Kane S, Pesterfield C, Guest C, Rooney NJ. An owner-independent investigation of diabetes alert dog performance. Front Vet Sci. (2019) 6:91. doi: 10.3389/fvets.2019.00091
230. LoGrippo GA. Investigations of the use of beta-propiolactone in virus inactivation. Ann N Y Acad Sci. (1960) 83:578–94. doi: 10.1111/j.1749-6632.1960.tb40931.x
231. LoGrippo GA, Hartman FW. Antigenicity of beta-propiolactone-inactivated virus vaccines. J Immunol. (1955) 75:123–8.
232. Budowsky E, Friedman E, Zheleznova N, Noskov F. Principles of selective inactivation of viral genome. VI. Inactivation of the infectivity of the influenza virus by the action of β-propiolactone. Vaccine. (1991) 9:398–402. doi: 10.1016/0264-410X(91)90125-P
233. Budowsky EI, Smirnov YA, Shenderovich SF. Principles of selective inactivation of viral genome. VIII. The influence of β-propiolactone on immunogenic and protective activities of influenza virus. Vaccine. (1993) 11:343–8. doi: 10.1016/0264-410X(93)90197-6
234. Chowdhury P, Topno R, Khan SA, Mahanta J. Comparison of β -propiolactone and formalin inactivation on antigenicity and immune response of West Nile virus. Adv Virol. (2015) 2015:616898. doi: 10.1155/2015/616898
235. Gao Q, Bao L, Mao H, Wang L, Xu K, Yang M, et al. Development of an inactivated vaccine candidate for SARS-CoV-2. Science. (2020) 369:77–81. doi: 10.1126/science.abc1932
236. Lei S, Gao X, Sun Y, Yu X, Zhao L. Gas chromatography-mass spectrometry method for determination of β-propiolactone in human inactivated rabies vaccine and its hydrolysis analysis. J Pharm Anal. (2018) 8:373–7. doi: 10.1016/j.jpha.2018.06.003
237. Uittenbogaard JP, Zomer B, Hoogerhout P, Metz B. Reactions of β-propiolactone with nucleobase analogues, nucleosides, and peptides. J Biol Chem. (2011) 286:36198–214. doi: 10.1074/jbc.M111.279232
238. Determann H, Joachim H-U. Zur reaktion von ß-propiolacton mit semmproteinen und aminosäurederivaten. Z klin Chem Klin Biochem. (1971) 9:398–401.
239. Lelie PN, Reesink HW, Lucas CJ. Inactivation of 12 viruses by heating steps applied during manufacture of a hepatitis B vaccine. J Med Virol. (1987) 23:297–301. doi: 10.1002/jmv.1890230313
240. Darnell MER, Subbarao K, Feinstone SM, Taylor DR. Inactivation of the coronavirus that induces severe acute respiratory syndrome, SARS-CoV. J Virol Methods. (2004) 121:85–91. doi: 10.1016/j.jviromet.2004.06.006
241. Saluzzo JF, Leguenno B, Van der Groen G. Use of heat inactivated viral haemorrhagic fever antigens in serological assays. J Virol Methods. (1988) 22:165–72. doi: 10.1016/0166-0934(88)90099-7
242. Edwards S. Survival and inactivation of classical swine fever virus. Vet Microbiol. (2000) 73:175–81. doi: 10.1016/S0378-1135(00)00143-7
243. Leclercq I, Batéjat C, Burguière AM, Manuguerra J. Heat inactivation of the Middle East respiratory syndrome coronavirus. Influenza Other Respir Viruses. (2014) 8:585–6. doi: 10.1111/irv.12261
244. Cutts T, Grolla A, Jones S, Cook BWM, Qiu X, Theriault SS. Inactivation of Zaire ebolavirus variant makona in human serum samples analyzed by enzyme-linked immunosorbent assay. J Infect Dis. (2016) 214:S218–21. doi: 10.1093/infdis/jiw289
245. Park SL, Huang Y-JS, Hsu W-W, Hettenbach SM, Higgs S, Vanlandingham DL. Virus-specific thermostability and heat inactivation profiles of alphaviruses. J Virol Methods. (2016) 234:152–5. doi: 10.1016/j.jviromet.2016.04.004
246. Abraham JP, Plourde BD, Cheng L. Using heat to kill SARS-CoV-2. Rev Med Virol. (2020) 30:e2115. doi: 10.1002/rmv.2115
247. Batéjat C, Grassin Q, Manuguerra J-C, Leclercq I. Heat inactivation of the severe acute respiratory syndrome coronavirus 2. J Biosaf Biosecur. (2021) 3:1–3. doi: 10.1016/j.jobb.2020.12.001
248. Heßling M, Hönes K, Vatter P, Lingenfelder C. Ultraviolet irradiation doses for coronavirus inactivation – Review and analysis of coronavirus photoinactivation studies. GMS Hyg Infect Control. (2020) 15:Doc08. doi: 10.3205/dgkh000343
249. Qiao Y, Yang M, Marabella IA, McGee DAJ, Aboubakr H, Goyal S, et al. Greater than 3-log reduction in viable coronavirus aerosol concentration in ducted ultraviolet-C (UV–C) systems. Environ Sci Technol. (2021) 55:4174–82. doi: 10.1021/acs.est.0c05763
250. Biasin M, Bianco A, Pareschi G, Cavalleri A, Cavatorta C, Fenizia C, et al. UV-C irradiation is highly effective in inactivating SARS-CoV-2 replication. Sci Rep. (2021) 11:6260. doi: 10.1038/s41598-021-85425-w
251. Budowsky EI, Bresler SE, Friedman EA, Zheleznova NV. Principles of selective inactivation of viral genome. Arch Virol. (1981) 68:239–47. doi: 10.1007/BF01314577
252. Perdiz D, Gróf P, Mezzina M, Nikaido O, Moustacchi E, Sage E. Distribution and repair of bipyrimidine photoproducts in solar UV-irradiated mammalian cells. J Biol Chem. (2000) 275:26732–42. doi: 10.1016/S0021-9258(19)61437-7
253. Browne K. Brought to light: how ultraviolet disinfection can prevent the nosocomial transmission of COVID-19 and other infectious diseases. Appl Microbiol. (2021) 1:537–56. doi: 10.3390/applmicrobiol1030035
254. Yao H, Feilberg A. Characterisation of photocatalytic degradation of odorous compounds associated with livestock facilities by means of PTR-MS. Chem Eng J. (2015) 277:341–51. doi: 10.1016/j.cej.2015.04.094
255. Yang X, Koziel JA, Laor Y, Zhu W, van Leeuwen J, Jenks WS, et al. VOC removal from manure gaseous emissions with UV photolysis and UV-TiO2 photocatalysis. Catalysts. (2020) 10:607. doi: 10.3390/catal10060607
256. Lee M, Koziel JA, Murphy W, Jenks WS, Chen B, Li P, et al. Evaluation of TiO2 based photocatalytic treatment of odor and gaseous emissions from swine manure with UV-A and UV-C. Animals. (2021) 11:1289. doi: 10.3390/ani11051289
257. Lee M, Koziel JA, Murphy W, Jenks WS, Chen B, Li P, et al. Mitigation of odor and gaseous emissions from swine barn with UV-A and UV-C photocatalysis. Atmosphere. (2021) 12:585. doi: 10.3390/atmos12050585
258. Fletcher DB. Successful UV/oxidation of VOC-contaminated groundwater. Remediat J. (1991) 1:353–7. doi: 10.1002/rem.3440010310
259. Sekiguchi K, Morinaga W, Sakamoto K, Tamura H, Yasui F, Mehrjouei M, et al. Degradation of VOC gases in liquid phase by photocatalysis at the bubble interface. Appl Catal B Environ. (2010) 97:190–7. doi: 10.1016/j.apcatb.2010.03.039
260. Aboubakr HA, Sharafeldin TA, Goyal SM. Stability of SARS-CoV-2 and other coronaviruses in the environment and on common touch surfaces and the influence of climatic conditions: a review. Transbound Emerg Dis. (2021) 68:296–312. doi: 10.1111/tbed.13707
261. Horowitz B, Lazo A, Grossberg H, Page G, Lippin A, Swan G. Virus inactivation by solvent/detergent treatment and the manufacture of SD-plasma. Vox Sang. (1998) 74:203–6. doi: 10.1111/j.1423-0410.1998.tb05473.x
262. Hellstern P. Solvent/detergent-treated plasma: composition, efficacy, and safety. Curr Opin Hematol. (2004) 11:346–50. doi: 10.1097/01.moh.0000137915.88478.23
263. Scheller C, Krebs F, Minkner R, Astner I, Gil-Moles M, Wätzig H. Physicochemical properties of SARS-CoV-2 for drug targeting, virus inactivation and attenuation, vaccine formulation and quality control. Electrophoresis. (2020) 41:1137–51. doi: 10.1002/elps.202000121
264. Biesert L, Suhartono H. Solvent/detergent treatment of human plasma – A very robust method for virus inactivation. Validated virus safety of OCTAPLAS®. Vox Sang. (1998) 74:207–12. doi: 10.1111/j.1423-0410.1998.tb05474.x
265. Ji H. Lysis of cultured cells for immunoprecipitation. Cold Spring Harb Protoc. (2010) 2010:pdb.prot5466. doi: 10.1101/pdb.prot5466
266. Olsen E, Nielsen F. Predicting vapour pressures of organic compounds from their chemical structure for classification according to the VOCDirective and risk assessment in general. Molecules. (2001) 6:370–89. doi: 10.3390/60400370
267. Smither SJ, Eastaugh LS, Findlay JS, Lever MS. Experimental aerosol survival of SARS-CoV-2 in artificial saliva and tissue culture media at medium and high humidity. Emerg Microbes Infect. (2020) 9:1415–7. doi: 10.1080/22221751.2020.1777906
268. Kwon T, Gaudreault NN, Richt JA. Seasonal stability of SARS-CoV-2 in biological fluids. Pathogens. (2021) 10:540. doi: 10.3390/pathogens10050540
269. van Doremalen N, Bushmaker T, Morris DH, Holbrook MG, Gamble A, Williamson BN, et al. Aerosol and surface stability of SARS-CoV-2 as compared with SARS-CoV-1. N Engl J Med. (2020) 382:1564–7. doi: 10.1056/NEJMc2004973
270. Kasloff SB, Leung A, Strong JE, Funk D, Cutts T. Stability of SARS-CoV-2 on critical personal protective equipment. Sci Rep. (2021) 11:984. doi: 10.1038/s41598-020-80098-3
271. Perrin TE, Rasmussen LEL, Gunawardena R, Rasmussen RA. A method for collection, long-term storage, and bioassay of labile volatile chemosignals. J Chem Ecol. (1996) 22:207–21. doi: 10.1007/BF02055093
272. Hsieh C-C, Horng S-H, Liao P-N. Stability of trace-level volatile organic compounds stored in canisters and tedlar bags. Aerosol Air Qual Res. (2003) 3:17–28. doi: 10.4209/aaqr.2003.06.0003
273. Miller TC, Morgera SD, Saddow SE, Takshi A, Palm M. Electronic nose with detection method for alcohol, acetone, and carbon monoxide in coronavirus disease 2019 breath simulation model. IEEE Sens J. (2021) 21:15935–43. doi: 10.1109/JSEN.2021.3076102
274. Caprio J. Electrophysiological distinctions between the taste and smell of amino acids in catfish. Nature. (1977) 266:850–1. doi: 10.1038/266850a0
275. Caprio J. Olfaction and taste in the channel catfish: an electrophysiological study of the responses to amino acids and derivatives. J Comp Physiol. (1978) 123:357–71. doi: 10.1007/BF00656970
276. Caprio J, Byrd RP. Electrophysiological evidence for acidic, basic, and neutral amino acid olfactory receptor sites in the catfish. J Gen Physiol. (1984) 84:403–22. doi: 10.1085/jgp.84.3.403
277. Nikonov AA, Caprio J. Highly specific olfactory receptor neurons for types of amino acids in the channel catfish. J Neurophysiol. (2007) 98:1909–18. doi: 10.1152/jn.00548.2007
278. Nikonov AA, Caprio J. Responses of olfactory forebrain units to amino acids in the channel catfish. J Neurophysiol. (2007) 97:2490–8. doi: 10.1152/jn.01198.2006
279. Naim M, Schutz O, Zehavi U, Rouseff RL, Haleva-Toledo E. Effects of orange juice fortification with thiols on p -vinylguaiacol formation, ascorbic-acid degradation, browning, and acceptance during pasteurization and storage under moderate conditions. J Agric Food Chem. (1997) 45:1861–7. doi: 10.1021/jf9608884
280. Laska M. Olfactory perception of 6 amino acids by human subjects. Chem Senses. (2010) 35:279–87. doi: 10.1093/chemse/bjq017
281. Halpern M. The organization and function of the vomeronasal system. Annu Rev Neurosci. (1987) 10:325–62. doi: 10.1146/annurev.ne.10.030187.001545
282. Keverne EB. The vomeronasal organ. Science. (1999) 286:716–20. doi: 10.1126/science.286.5440.716
283. Karim SSA, Karim QA. Omicron SARS-CoV-2 variant: a new chapter in the COVID-19 pandemic. Lancet. (2021) 398:2126–8. doi: 10.1016/S0140-6736(21)02758-6
284. Tao K, Tzou PL, Nouhin J, Gupta RK, de Oliveira T, Kosakovsky Pond SL, et al. The biological and clinical significance of emerging SARS-CoV-2 variants. Nat Rev Genet. (2021) 22:757–73. doi: 10.1038/s41576-021-00408-x
285. Murarka M, Vesley-Gross ZI, Essler JL, Smith PG, Hooda J, Drapkin R, et al. Testing ovarian cancer cell lines to train dogs to detect ovarian cancer from blood plasma: a pilot study. J Vet Behav. (2019) 32:42–8. doi: 10.1016/j.jveb.2019.04.010
286. Ciurkiewicz M, Armando F, Schreiner T, de Buhr N, Pilchová V, Krupp-Buzimikic V, et al. Ferrets are valuable models for SARS-CoV-2 research. Vet Pathol. (2022) 59:661–72. doi: 10.1177/03009858211071012
287. Paul-Ehrlich-Institut. Mindestkriterien für SARS-CoV-2 Antigentests im Sinne von §1 Abs. 1 Satz 1 TestVO: Antigenschnelltests. (2022). Available online at: https://www.pei.de/SharedDocs/Downloads/DE/newsroom/dossiers/mindestkriterien-sars-cov-2-antigentests.pdf?__blob=publicationFile&v=9 (accessed May 1, 2022).
288. FAO, OIE, WHO, UNEP. Joint Tripartite (FAO, OIE, WHO) and UNEP Statement Tripartite and UNEP Support OHHLEP’s Definition of “One Health”. (2022). Available online at: https://wedocs.unep.org/bitstream/handle/20.500.11822/37600/JTFOWU.pdf (accessed May 5, 2022).
289. Aleta A, Martín-Corral D, Pastore y Piontti A, Ajelli M, Litvinova M, Chinazzi M, et al. Modelling the impact of testing, contact tracing and household quarantine on second waves of COVID-19. Nat Hum Behav. (2020) 4:964–71. doi: 10.1038/s41562-020-0931-9
290. WHO. COVID-19 Strategic Preparedness and Response Plan (SPRP 2021). (2022). Available online at: https://www.who.int/publications-detail-redirect/WHO-WHE-2021.02 (accessed April 10, 2022).
291. Dai T, Zaman MH, Padula WV, Davidson PM. Supply chain failures amid COVID-19 signal a new pillar for global health preparedness. J Clin Nurs. (2021) 30:e1–3. doi: 10.1111/jocn.15400
292. Jayamohan H, Lambert CJ, Sant HJ, Jafek A, Patel D, Feng H, et al. SARS-CoV-2 pandemic: a review of molecular diagnostic tools including sample collection and commercial response with associated advantages and limitations. Anal Bioanal Chem. (2021) 413:49–71. doi: 10.1007/s00216-020-02958-1
293. Vandenberg O, Martiny D, Rochas O, van Belkum A, Kozlakidis Z. Considerations for diagnostic COVID-19 tests. Nat Rev Microbiol. (2021) 19:171–83. doi: 10.1038/s41579-020-00461-z
294. EC. EU Guidance on Operating Procedures for Explosive Detection Dogs in Public Spaces. (2022). Available online at: https://ec.europa.eu/newsroom/pps/items/696384 (accessed May 1, 2022).
Keywords: canine detection, SARS-CoV-2, COVID-19, test system, pandemic control
Citation: Meller S, Al Khatri MSA, Alhammadi HK, Álvarez G, Alvergnat G, Alves LC, Callewaert C, Caraguel CGB, Carancci P, Chaber A-L, Charalambous M, Desquilbet L, Ebbers H, Ebbers J, Grandjean D, Guest C, Guyot H, Hielm-Björkman A, Hopkins A, Kreienbrock L, Logan JG, Lorenzo H, Maia RdCC, Mancilla-Tapia JM, Mardones FO, Mutesa L, Nsanzimana S, Otto CM, Salgado-Caxito M, de los Santos F, da Silva JES, Schalke E, Schoneberg C, Soares AF, Twele F, Vidal-Martínez VM, Zapata A, Zimin-Veselkoff N and Volk HA (2022) Expert considerations and consensus for using dogs to detect human SARS-CoV-2-infections. Front. Med. 9:1015620. doi: 10.3389/fmed.2022.1015620
Received: 09 August 2022; Accepted: 17 November 2022;
Published: 08 December 2022.
Edited by:
Olivier Vandenberg, Laboratoire Hospitalier Universitaire de Bruxelles (LHUB-ULB), BelgiumReviewed by:
Thomas Passler, Auburn University, United StatesCopyright © 2022 Meller, Al Khatri, Alhammadi, Álvarez, Alvergnat, Alves, Callewaert, Caraguel, Carancci, Chaber, Charalambous, Desquilbet, Ebbers, Ebbers, Grandjean, Guest, Guyot, Hielm-Björkman, Hopkins, Kreienbrock, Logan, Lorenzo, Maia, Mancilla-Tapia, Mardones, Mutesa, Nsanzimana, Otto, Salgado-Caxito, de los Santos, da Silva, Schalke, Schoneberg, Soares, Twele, Vidal-Martínez, Zapata, Zimin-Veselkoff and Volk. This is an open-access article distributed under the terms of the Creative Commons Attribution License (CC BY). The use, distribution or reproduction in other forums is permitted, provided the original author(s) and the copyright owner(s) are credited and that the original publication in this journal is cited, in accordance with accepted academic practice. No use, distribution or reproduction is permitted which does not comply with these terms.
*Correspondence: Sebastian Meller, c2ViYXN0aWFuLm1lbGxlckB0aWhvLWhhbm5vdmVyLmRl
†These authors share senior authorship