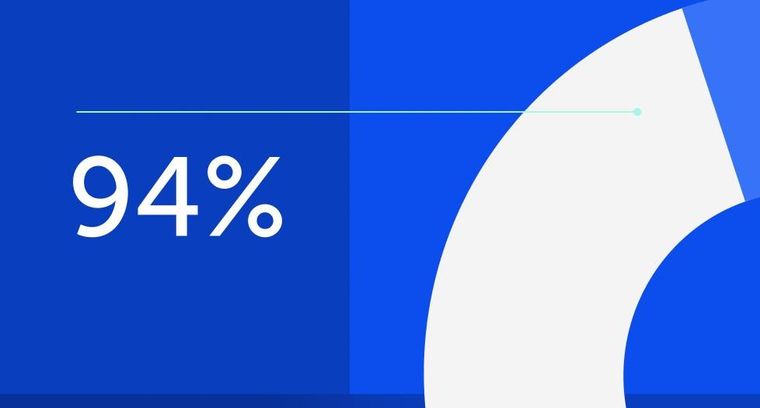
94% of researchers rate our articles as excellent or good
Learn more about the work of our research integrity team to safeguard the quality of each article we publish.
Find out more
ORIGINAL RESEARCH article
Front. Med., 27 September 2022
Sec. Nuclear Medicine
Volume 9 - 2022 | https://doi.org/10.3389/fmed.2022.1008200
This article is part of the Research TopicDevelopment of Selective Carbon-11 Radioligands for Target-Based PET Molecular Imaging in Oncology, Cardiology and NeurologyView all 8 articles
Background: De novo lipogenesis is upregulated in many cancers, and targeting it represents a metabolic approach to cancer treatment. However, the treatment response is unpredictable because lipogenic activity varies greatly among individual tumors, thereby necessitating the assessment of lipogenic activity before treatment. Here, we proposed an imaging probe, positron emission tomography/computed tomography (PET/CT) with dual tracers combining 11C-acetate and 18F-fluorodeoxyglucose (18F-FDG), to assess the lipogenic activity of hepatocellular carcinoma (HCC) and predict the response to lipogenesis-targeted therapy.
Methods: We investigated the association between 11C-acetate/18F-FDG uptake and de novo lipogenesis in three HCC cell lines (from well-differentiated to poorly differentiated: HepG2, Hep3B, and SkHep1) by examining the expression of lipogenic enzymes: acetyl-CoA synthetase 2 (ACSS2), fatty acid synthase (FASN), and ATP citrate lyase (ACLY). The glycolysis level was determined through glycolytic enzymes: pyruvate dehydrogenase expression (PDH). On the basis of the findings of dual-tracer PET/CT, we evaluated the treatment response to a lipase inhibitor (orlistat) in cell culture experiments and xenograft mice.
Results: Dual-tracer PET/CT revealed the lipogenic activity of various HCC cells, which was positively associated with 11C-acetate uptake and negatively associated with 18F-FDG uptake. This finding represents the negative association between 11C-acetate and 18F-FDG uptake. Because these two tracers revealed the lipogenic and glycolytic activity, respectively, which implies an antagonism between lipogenic metabolism and glucose metabolism in HCC. In addition, dual-tracer PET/CT not only revealed the lipogenic activity but also predicted the treatment response to lipogenesis-targeted therapy. For example, HepG2 xenografts with high 11C-acetate but low 18F-FDG uptake exhibited high lipogenic activity and responded well to orlistat treatment, whereas SkHep1 xenografts with low 11C-acetate but high 18F-FDG uptake exhibited lower lipogenic activity and poor response to orlistat.
Conclusion: The proposed non-invasive dual-tracer PET/CT imaging can reveal the lipogenesis and glycolysis status of HCC, thus providing an ideal imaging probe for predicting the therapeutic response of HCC to lipogenesis-targeted therapy.
Targeting de novo lipogenesis represents a metabolic approach to cancer treatment (1–3) because lipogenesis is upregulated in various cancers (4, 5). This is achieved by inhibiting the lipogenic enzymes, such as fatty acid synthase (FASN) (5–7). However, the treatment response to lipogenesis inhibitors is highly variable (8) because of considerable intertumoral variation in lipogenic activity (9, 10). Therefore, non-invasive determination of the lipogenic activity of tumor cells before lipogenesis-targeted therapy is essential.
Imaging with 11C-acetate positron emission tomography/computed tomography (PET/CT) can assess the lipogenic activity and may thus help predict the response to lipogenesis-targeted therapy. 11C-acetate PET/CT has been used to evaluate the lipogenic metabolism in prostate cancer because 11C-acetate is an analog of lipogenic precursors and participates in the lipogenic pathway (11, 12). In addition, 11C-acetate PET imaging has a sensitivity of 87.3% in diagnosing hepatocellular carcinoma (HCC) (13), which can be increased to nearly 100% by using 11C-acetate in combination with 18F-fluorodeoxyglucose (18F-FDG). Although 18F-FDG PET/CT demonstrates glycolytic activity (14, 15), it is unclear whether the dual-tracer PET/CT combining 11C-acetate in combination with 18F-FDG is a better imaging probe for determining the lipogenic activity of HCC and predicting the therapeutic response to targeting lipogenesis.
The value of 11C-acetate and 18F-FDG PET imaging before and after molecular therapy was used to evaluate the prognosis of HCC patients in a recent study (16). The novelty of our study provides new insights of using dual-tracer PET/CT imaging to assess the lipogenic activity of various HCC cell lines with different differentiation states and to evaluate the therapeutic response of lipogenic inhibitor. Our findings revealed how 11C-acetate and 18F-FDG uptake varied with the expression of lipogenic and glycolytic enzymes, respectively, and demonstrated the association between lipogenesis and glycolysis in HCC. Dual-tracer PET/CT can not only provide an immediate metabolic status of HCC but may also serve as a more accurate imaging probe for de novo lipogenesis. By using the non-invasive dual-tracer imaging, we successfully predicted the therapeutic response to a lipogenesis inhibitor (orlistat) in HCC xenograft mice.
We investigated three HCC cell lines (Bioresource Collection and Research Center Hsinchu, Taiwan) with various differentiation states: HepG2 (well-differentiated), Hep3B (moderately differentiated), and SkHep1 (poorly differentiated) (17). HepG2 and Hep3B cell lines were cultured in Dulbecco’s modified Eagle’s medium (Invitrogen/cat. no. 31800-012), and the SkHep1 cell line was cultured in minimum essential medium alpha (Invitrogen/cat. no. 12000-022). In all cell cultures, 10% fetal bovine serum (Hyclone) and 1% penicillin/streptomycin/glutamine cocktail (Invitrogen) were added to the growth media, and the cell lines were cultured at 37°C with 5% CO2 in humid incubators.
The 11C-acetate for in vivo use was synthesized through the carbonation of Grignard reagent. 11C-carbon dioxide was reacted with 0.15 M methylmagnesium bromide and hydrolyzed with 0.4 M hydrochloric acid to be converted to 11C-acetate acid (18). All reaction steps were performed using a robotic system (Scanditronix Anatech RB III) controlled by a computer. The radiochemical purity was greater than 99%. 18F-FDG was synthesized as described in the literature (19). 14C-acetate for in vitro use was obtained from Blossom Biotechnologies.
Aliquots of 1 μCi/500 μl of a radiotracer (18F-FDG or 14C-acetate) were added to each well containing preseeded cells cultured overnight in a 24-well culture plate at a density of 3 × 105 cells/well. After 1-h incubation, the cellular uptake of radiotracers was terminated by rinsing the cells isolated from the supernatant in ice-cold phosphate-buffered saline (PBS). These cells were trypsinized and harvested. Next, the radioactivity of both (18F-FDG and 14C-acetate) harvested cells and the remaining supernatant was determined. Finally, all the cells were lysed, and the cellular proteins were extracted using CytoBuster Protein Extraction Reagent. The cellular uptake of 18F-FDG was determined as (radioactivity of cells)/[(radioactivity of supernatant) × (protein concentration)] by using the Cobra II Auto-Gamma Counter (PerkinElmer). For 14C-acetate uptake, we added scintillation fluid (Ultima Gold, PerkinElmer) to determine the radioactivity by using a Packard Tri-Carb LS β Counter (PerkinElmer). 18F-FDG and 14C-acetate uptake (gamma counter and beta counter, respectively) in cells is normalized by protein weight: counts per minute (CPM)/mg protein.
In the orlistat treatment group, 300 mM orlistat was added to the cell culture medium 2 h before the 14C-acetate uptake experiment. After 2 h, the cell uptake experiments continued, while orlistat remained in the culture medium.
The animal experiments were approved by the animal care and use committee of the institution. For the subcutaneous tumor models, HCC xenografts were established by inoculating approximately 107 tumor cells into the shoulders of 7-week-old NOD/SCID mice (BioLASCO Taiwan). In total, 11 mice were inoculated with HepG2 cells and 10 with SkHep1 cells. When the volume of the inoculated tumor grew to approximately 100 mm3, the mice were injected with 500 μCi 18F-FDG or 11C-acetate through their tail vein and were subjected to whole-body micro-PET/CT imaging (FLEX Triumph preclinical PET/SPECT/CT scanner; TriFoil Imaging). Specifically, the mice were first subjected to CT imaging to acquire images of 256 × 256 pixels, at a current of 0.5 mA and voltage of 80 kVp, and then subjected to PET imaging for 20 min. The cone–beam reconstruction algorithm and 3D-ordered subset expectation maximization algorithm were applied to reconstruct the CT and PET images, respectively. All the reconstructed images were further analyzed using Amide (version 1.0.4; Crump Institute for Molecular Imaging, Los Angeles, CA, USA), a software for analyzing anatomical and functional volumetric medical imaging data in the Digital Imaging and Communications in Medicine standard (20). Specifically, Amide software was applied to represent the imaging with a color-based radiometric scale and to quantify the radioactivity in the regions of interest, including the tumors and thighs of the mice, and to calculate the tumor-to-muscle ratio (T/M ratio) based on percent intensity dose per gram (%ID/g) values (20).
We conducted a quantitative real-time polymerase chain reaction (qPCR) to examine gene expression in HCC cells. First, we prepared the samples for qPCR by using TRIzol reagent (Invitrogen) to extract the total RNA from the tumor tissues. Then, the extracted RNA was reverse-transcribed into first-strand cDNA. By mixing the cDNA (40 ng) with SYBER Green PCR Master Mix (Applied Biosystems) and primers of target genes, qPCR was performed using a StepOnePlus Real-Time System (Applied Biosystems) under the following conditions: activation at 95°C for 20 s, followed by 40 cycles of 95°C for 1 s, and 60°C for 20 s. Finally, the qPCR results were normalized with respect to the expression of the housekeeping gene glyceraldehyde 3-phosphate dehydrogenase.
Western blotting was used to extract lipogenic enzymes from the tumor cells by using the Bradford Protein Assay Kit (Bio-Rad). Specifically, 20 μg of bulk protein extracted from tumor cells with sample buffer (4 × SDS loading buffer: 10% glycerol, 62.5 mM Tris–HCl, pH: 6.8, 2% SDS, 65 mM DTT, and 0.05% bromophenol blue) was incubated in boiling water for 10 min. Through sodium dodecyl sulfate-polyacrylamide gel electrophoresis, the extracted protein was first transferred to polyvinylidene difluoride (PVDF) membranes, which were blocked with 5% (w/v) non-fat milk in PBS. Next, the transferred proteins on the PVDF membrane were reacted with primary antibodies, including anti-FASN (1:1,000, Genetex GTX109833), anti-ACSS2 (1:1,000, Genetex GTX30020), and anti-β-actin (1:1,000, Genetax GTX109639, as the internal control) at 4°C overnight. Next, the PVDF membranes were gently washed with TBST (50 mM Tris–HCl, pH: 7.4, 200 mM NaCl, 1% Tween-20) and incubated with HRPase-conjugated goat anti-rabbit IgG secondary antibodies (1:5,000; LI-COR Biosciences) at room temperature for 1 h. Finally, the chemiluminescent signals of the extracted proteins were examined using Western Lightning Plus-ECL and detected using the LAS 4000 mini-imaging system (Fujifilm).
The activity of de novo lipogenesis in cultured HCC cells was assessed based on the proportion of cellular lipids derived from exogenous 14C-labeled acetate. First, preseeded tumor cells were incubated overnight in a 24-well culture plate at 6 × 104 cells/well. Next, aliquots of 1 μCi/500 μl of 14C-acetate were added to each well and incubated for 1 h after the medium was removed and the cells were rinsed with PBS. Next, the cellular uptake of 14C-acetate was terminated by isolating the cells from the supernatant and rinsing them with ice-cold PBS. Subsequently, cellular lipids were extracted through centrifugation (1,000 rpm for 5 min) of the cells in the solution containing a mixture of 0.6 ml of MeOH (MeOH: H2O = 2.5:1) and 0.4 ml of CHCl3. Finally, 14C-acetate incorporated into the extracted lipids was determined using a Packard Tri-Carb LS β Counter (PerkinElmer) in scintillation solutions (PerkinElmer). 14C-acetate uptake in cells was normalized by protein weight (CPM/mg protein). In the orlistat treatment group, 300 mM orlistat was added to the cell culture medium 2 h before 14C-acetate incorporation into lipid experiments. After 2 h, the experiments were continued, with orlistat still in the culture medium.
The response of the HCC cell cycle to lipogenesis-targeted treatment was examined. For this purpose, preseeded cells (6-well plates at 2 × 105 cells/well) were treated with 150 μM orlistat dissolved in ethanol for 24 h and compared with the mock control treated with ethanol alone. Next, the orlistat treatment was terminated through addition of ice-cold 70% ethanol at −20°C for 1 h and washing of the cells with ice-cold PBS. Finally, the cells were stained with 40 μg/ml of propidium iodide reagent (BioVision) for 1 h at room temperature in the dark. Subsequently, the cell cycle phases were analyzed using a flow cytometer (FACS Calibur; BD Bioscience), which differentiated G0/G1, S, and G2/M phases according to the proportion of fluorescent areas in the cells (21).
To investigate the inhibitory effects of orlistat on HCC cells in vivo, we first established SkHep1 and HepG2 xenografts by inoculating 107 tumor cells subcutaneously into the shoulder of ten 7-week-old NOD/SCID mice (five in the experimental group and five in the control group; BioLASCO Taiwan). The length and width of the tumors were measured every 2 days by using a caliper, and the tumor volume (mm3) was calculated as length × width2 × 0.52. When the length of the tumor reached 5 mm, orlistat was injected intraperitoneally into the mice at 240 mg/kg/day for 14 days. At the end of the study period, all mice were killed using carbon dioxide asphyxiation. All animal experiments were approved by the animal care and use committee of the institution.
The statistical significance of the various experimental treatments was tested. Permutation tests were conducted for the treatments of orlistat (orlistat vs. control), and the Kruskal–Wallis test (non-parametric ANOVA) was performed to compare the three (or more)-level treatments (e.g., to evaluate the statistical differences between the three HCC cell lines).
The well-differentiated HepG2 cells exhibited high lipogenic activity, whereas the poorly differentiated SkHep1 cells exhibited low lipogenic activity. PCR and Western blotting indicated that HepG2 cells overexpressed three lipogenic enzymes: acetyl-CoA synthetase 2 (ACSS2), FASN, and ATP citrate lyase (ACLY) (Figure 1 and Supplementary Figure 1). By contrast, SkHep1 cells underexpressed the three lipogenic enzymes. In the moderately differentiated Hep3B cell line, lipogenesis was partially inhibited, and the expression of only ACSS2 was decreased. Thus, the results revealed a clear association between lipogenic activity and the differentiation status of HCC cell lines. Notably, the well-differentiated HepG2 cells exhibited not only high lipogenesis but also low glycolysis, as indicated by the underexpression of the glycolysis-associated enzyme pyruvate dehydrogenase (PDH) (Figure 1D). By contrast, SkHep1 cells exhibited not only low lipogenesis but also high glycolysis, as indicated by PDH overexpression.
Figure 1. Gene expression of lipogenic enzymes and glycolysis-associated enzymes in three HCC cell lines. Gene expression analysis in three lipogenic enzymes through real-time PCR revealed that (A) FASN expression is significantly lower in SkHep1 cells than in Hep3B and HepG2 cells (Kruskal–Wallis test ***p < 0.001; n = 24 for each cell line), (B) ACSS2 expression is significantly higher in HepG2 cells than in Hep3B and SkHep1 cells (Kruskal–Wallis test ***p < 0.001; n = 12 for each cell line), and (C) ACLY expression (Kruskal–Wallis test *p = 0.016; n = 6 for each cell line) is significantly lower in SkHep1 cells than in Hep3B and HepG2 cells. (D) The expression of glycolysis-associated enzyme PDH in SkHep1 cells is significantly higher than that in Hep3B and HepG2 cells (Kruskal–Wallis test **p = 0.002; n = 15 for each cell line). We summarized all the measurements as box-plots that label 25, 50, and 75% quantiles in the boxes with whiskers presenting at most 1.5 × interquartile range.
Dual-tracer PET/CT revealed high 11C-acetate uptake but low 18F-FDG uptake in the HepG2 cells, and low 11C-acetate uptake but high 18F-FDG uptake in the SkHep1 cells. In the in vitro culture experiments, acetate uptake was significantly higher but 18F-FDG uptake was significantly lower in HepG2 cells than in SkHep1 cells (Figures 2A,B, respectively). Similarly, in vivo micro-PET/CT imaging revealed significantly higher 11C-acetate accumulation and lower 18F-FDG accumulation in HepG2 xenografts than in SkHep1 xenografts (Figures 2C–F), which represents the negative association between 11C-acetate and 18F-FDG uptake. Considering that acetate uptake was correlated with lipogenic activity in HepG2 and SkHep1 cells, while 18F-FDG uptake was correlated with glycolytic activity (Figure 1 and Supplementary Figure 1), we concluded that there is an antagonism between lipogenic metabolism and glucose metabolism in HCC. The associations between lipogenic activity and acetate uptake were further confirmed with 14C-acetate addition experiments (Figure 3), in which the proportion of 14C-acetate-derived lipids in HepG2 cells was significantly higher than that in Hep3B and SkHep1 cells. Consequently, dual-tracer PET/CT combined with 11C-acetate and 18F-FDG are capable of revealing the lipogenesis and glycolysis of HCC, respectively, both in vitro and in vivo (Figure 2).
Figure 2. Dual-tracer uptake in three HCC cell lines in vitro and in vivo. In vitro, (A) 14C-acetate uptake in HepG2 cells is significantly higher than that in SkHep1 and Hep3B cells (Kruskal–Wallis test ***p < 0.001; n = 7, 10, and 10 for HepG2, Hep3B, and SKHep1, respectively). (B) 18F-FDG uptake in SkHep1 cells is significantly higher than that in HepG2 and Hep3B cells (Kruskal–Wallis test *p = 0.045; n = 6, 6, and 5 for HepG2, Hep3B, and SKHep1, respectively). In vivo, (C) 11C-acetate micro-PET imaging of HCC xenografts in nude mice, represented by a color-based radiometric scale, revealed that (D) 11C-acetate uptake assessed using the tumor-to-muscle ratio is significantly higher in HepG2 cells than in SkHep1 cells (D; permutation test ***p < 0.001; n = 6 and 5 for HepG2 and SkHep1, respectively); whereas (E) 18F-FDG micro-PET imaging of HCC xenografts revealed that (F) 18F-FDG uptake is significantly higher in SkHep1 cells than in HepG2 cells (F; permutation test ***p < 0.001; n = 5 for each cell line). The red arrows in dual-tracer micro-PET imaging in C,E indicate the location of the HCC xenografts. We summarized the measurements as box-plots that label 25, 50, and 75% quantiles in the boxes with whiskers presenting at most 1.5 × interquartile range.
Figure 3. 14C-acetate incorporated into cellular lipid fractions in three HCC cell lines. 14C-acetate was incorporated into the cellular lipid fraction in the three HCC cell lines. In the extracted lipid fraction, 14C-acetate uptake in HepG2 was significantly higher than that in Hep3B and SkHep1 (***p < 0.001; permutation test; n = 6, 9, and 9 for HepG2, Hep3B, and SkHep1, respectively), indicating that 14C-acetate was intensively accumulated in HepG2 cells and subsequently incorporated into cellular lipid fractions. 14C-acetate uptake (β counter) in cells normalized by protein weight (CPM/mg protein). We summarized the measurements as box-plots that label 25, 50, and 75% quantiles in the boxes with whiskers presenting at most 1.5 × interquartile range.
Lipogenic activity was used to determine the response of HCC to orlistat treatment in vitro and in vivo. In the in vitro experiments, HepG2 cells with high lipogenic activity (Figure 1 and Supplementary Figure 1) had a good response to orlistat, which was indicated by the significant reduction in the uptake of 14C-acetate and in the production of 14C-acetate-derived lipids (Figure 4). In addition, orlistat inhibited the proliferation of HepG2 cells, which significantly reduced the number of cells in the S/G2/M cell cycle phase (Figure 5). By contrast, the other two cell lines, Hep3B and SkHep1, with decreased lipogenic activity, showed a poor response to orlistat treatment and no significant reduction in proliferation. Similar to in vitro findings, orlistat significantly inhibited tumor growth in HepG2 xenografts, but not in SkHep1 xenografts (Figure 6). That is, cell lines with high lipogenic activity responded well to lipogenesis-targeted treatment with orlistat. As mentioned earlier, lipogenic activity in HCC cells can be indicated by dual-tracer PET/CT imaging combined with 11C-acetate and 18F-FDG; our results verify that dual-tracer PET/CT imaging can not only assess lipogenic activity but also predict the response to lipogenesis-targeted therapy in a non-invasive manner.
Figure 4. Treatment response to orlistat treatment assessed using 14C-acetate uptake and 14C-acetate incorporated lipid fractions in three HCC cell lines. In HepG2 cells, (A) 14C-acetate uptake and (B) 14C-acetate incorporated lipid fractions were significantly inhibited by orlistat treatment (labeled by light gray) compared with mock treatment (labeled by dark gray) (permutation test ***p < 0.001 in both A,B; n = 6 for each bar). However, orlistat-induced inhibition was not significant in Hep3B and SkHep1 (permutation test p = 0.236 and 0.630, respectively; n = 6 for each bar). 14C-acetate uptake (β counter) in cells normalized by protein weight (CPM/mg protein). We summarized these measurements as bar plots that label the averaged values with error bars presenting the standard deviation (±1 SD) computed from six replicates for each treatment (n = 6 for each bar).
Figure 5. Treatment response to orlistat assessed using cell cycle analysis with flow cytometry in three HCC cell lines. HepG2 cells in the S/G2/M cell cycle were obviously reduced after orlistat treatment compared with mock treatment, whereas no apparent change was observed in the cell cycle in Hep3B and SkHep1 cells. The proportion was determined by counting 2 × 105 cells for each treatment (n = 2 × 105).
Figure 6. Treatment response to orlistat in HCC xenografts assessed using tumor growth rates in nude mice. The tumor doubling time was significantly reduced in (A) orlistat-treated HepG2 xenograft mice compared with controls (*p = 0.015; n = 2 and 3 xenografts for control and orlistat treatment, respectively), whereas no significant difference was observed in (B) SkHep1 xenografts (p = 0.561; n = 3 xenografts for each treatment). The open and solid circles are the averaged standardized tumor volumes for control and orlistat treatment, respectively. The averaged tumor volume as well as its error bar presenting the uncertainty (±1 SD) was calculated from the replicates observed at different times.
14C-acetate uptake revealed the activity of de novo lipogenesis involving multiple lipogenic enzymes in HCC, whereas the expression of individual lipogenic enzymes (e.g., FASN) revealed only partial information on lipogenesis. Although 14C-acetate uptake was positively associated with FASN expression in HepG2 and SkHep1 cells (Figures 1, 2), Hep3B cells exhibited a discrepancy between 14C-acetate uptake (low) and FASN expression (high), consistent with a previous study on HCC (22). Despite Hep3B cells highly expressing FASN, the reduction in 14C-acetate uptake more accurately revealed the reduction in lipogenesis (Figures 2, 3) and better predicted its poor response to antilipogenic therapy with orlistat (Figure 4). 14C-acetate can reveal lipogenic activity because it is involved in multiple steps of the lipogenic pathway (11, 12), whereas FASN is involved in only one step of the lipogenic pathway. Consequently, 14C-acetate uptake level provides a more comprehensive assessment of lipogenic activity than individual lipogenic enzymes do, whereas more detailed analysis is still required for examining the incorporation of 14C-acetate in the metabolism of various lipid classes.
Compared with the single tracer of 14C-acetate, dual tracers combining 14C-acetate with 18F-FDG more accurately assess lipogenic activities across various HCC cells. For instance, in the absence of 18F-FDG, only 14C-acetate uptake failed to distinguish the differences in lipogenic activity between Hep3B cells and SkHep1 (Figure 2A) because they had similar acetate uptakes. Nonetheless, the addition of 18F-FDG imaging can help distinguish the lipogenic activity between Hep3B and SkHep1 cells because SkHep1 cells have high glycolytic activity coupled with low lipogenic activity (Figures 1, 2B); a biochemical connection between glycolysis and lipogenic metabolism in HCC has been reported (23). Therefore, to better distinguish lipogenic activity among the various HCC cell lines, use of dual tracers is essential. Moreover, a comprehensive in vivo imaging analyses before and after lipogenesis-targeted therapy, including more types of HCC cell lines, are warranted to corroborate our findings. In addition, with the technical progress of new generation PET/CT instruments, the effective radiation dose of dual-tracer PET/CT has been substantially reduced to 14.3 mSv to avoid the radiation burden of HCC patients, compared with diagnostic abdominal CT with the effective radiation dose of 20 mSv (24–26). We suggest that the proposed dual-tracer PET imaging can track cancer metabolic changes and provide a clearer picture for designing suitable and individualized treatment strategies.
In addition to lipogenic and glycolytic metabolism, dual-tracer PET/CT imaging can reveal other metabolic pathways of HCC cells, which can be used to predict the prognosis of patients with HCC. In our study, dual PET/CT imaging in well-differentiated HepG2 cells exhibited high 11C-acetate accumulation and low 18F-FDG. Increased 11C-acetate uptake might indicate not only increased lipogenesis (Figures 1, 2) but also increased oxidative phosphorylation (27), in which catalyzed 11C-acetate (e.g., for producing acetyl-CoA) plays critical roles in both lipogenesis and oxidative phosphorylation metabolic pathways. However, when 11C-acetate uptake is reduced (e.g., in poorly differentiated SkHep1 in Figures 1, 2), metabolic status may shift from active lipogenesis and oxidative phosphorylation, respectively, to increased demand for exogenous lipids (overexpression of lipid transporters such as lipoprotein lipase, LPL) (28, 29) and exogenous glucose (overexpression of glucose transporter, e.g., glucose transporter 1, GLUT1 for anaerobic glycolysis) (30, 31). Because both LPL and GLUT1 are considered poor prognosis factors of HCC (29, 31, 32), dual-tracer PET/CT imaging might be used to unveil the metabolic status of HCC to predict the prognosis of patients with HCC. Nevertheless, the application of dual-tracer imaging to explore cancer metabolism and associated treatments requires more comprehensive in vivo imaging and in vitro experimental data that target gene and protein expression of various enzymes involved in different stages of metabolic pathways in future works.
Cholesterol is a major lipid component of cell membrane and lipoproteins. Cholesterol metabolism provides the prognostic signature for HCC (33). There is a functional crosstalk in hepatocarcinogenesis between cholesterol biosynthesis and de novo lipogenesis, sharing the same precursor of acetyl-CoA (34, 35). Thus, 11C-acetate tracer can be also involved in cholesterol metabolism. However, the relative proportions of acetyl-CoA involved in cholesterol metabolism and de novo lipogenesis in HCC remain unclear (36) and require more detailed investigations in the future.
Dual-tracer imaging can serve as a non-invasive method for selecting patients with HCC with high lipogenic activity for lipogenesis-targeted therapy, thereby facilitating the development of commercial drugs (Supplementary Figure 2). Currently, antilipogenic drugs targeting lipogenic enzymes (2, 37, 38) and transcription factors (39–41) are rapidly being developed. However, none of these drugs have completed clinical human trials or entered commercial production. One possible reason is the lack of treatment guidance for selecting patients with HCC who respond well to these drugs. Our dual-tracer PET/CT imaging can reveal the lipid metabolism status of HCC cells to provide image guidance for the selection of patients with HCC with high lipogenic activity. For example, patients with HCC with high 11C-acetate but low 18F-FDG uptake on dual-tracer PET/CT exhibit high lipogenic activity and may respond well to lipogenesis inhibitors (Figures 4, 6). By contrast, patients with low 11C-acetate but high 18F-FDG uptake exhibit low lipogenic activity and may respond poorly to lipogenesis inhibitors, although alternative therapies that target exogenous lipid transporters (e.g., LPL) may elicit a good response (42). This imaging-based treatment guidance must be validated in more detailed analyses and clinical trials. Our proposed dual-tracer PET/CT imaging opens up a promising research direction to reveal the lipogenic metabolism status of HCC in a non-invasive manner. Eventually, the proposed imaging probe might guide the future development of lipogenesis-targeted drugs through appropriate patient selection.
Our proposed non-invasive dual-tracer PET/CT combining 11C-acetate with 18F-FDG is an imaging probe that can effectively reveal the lipogenic activity of HCC cells and predict their response to lipogenesis-targeted therapy.
The original contributions presented in this study are included in the article/Supplementary material, further inquiries can be directed to the corresponding authors.
This study was performed in accordance with a plan that was reviewed and approved by the Ethics Committee for Animal Experiments of National Yang Ming Chiao Tung University.
C-HT and R-SL conceived and designed the study. R-HJ and C-CK performed the experiments. Chi-WC prepared the radiopharmaceuticals. C-HT and Chu-WC analyzed the data. C-HT and R-SL wrote the manuscript, with critical comments from the co-authors. All authors contributed to the article and approved the submitted version.
This manuscript was edited by two professional English editors, Katie Fonseca and Agarwal from Wallace Academic Editing.
The authors declare that the research was conducted in the absence of any commercial or financial relationships that could be construed as a potential conflict of interest.
All claims expressed in this article are solely those of the authors and do not necessarily represent those of their affiliated organizations, or those of the publisher, the editors and the reviewers. Any product that may be evaluated in this article, or claim that may be made by its manufacturer, is not guaranteed or endorsed by the publisher.
The Supplementary Material for this article can be found online at: https://www.frontiersin.org/articles/10.3389/fmed.2022.1008200/full#supplementary-material
Supplementary Figure 1. Protein expression in two lipogenic enzymes, FASN and ACSS2, by Western blotting in three HCC cell lines. Our analysis revealed that (A) FASN expression was lower in SkHep1 cells than in Hep3B and HepG2 cells and (B) ACSS2 expression in HepG2 cells was higher than that in Hep3B and SkHep1 cells. Protein expression level is quantified in a relative sense with respect to protein expression of housekeeping gene, β-actin.
Supplementary Figure 2. Schematic diagram of de novo lipogenesis. The diagram presents biochemical processes that critically affects lipid synthesis via the key enzymes for possible drug targets (red box; FASN, ACLY, and ACSS2 were analyzed in this study). In this study, we examined the treatment response of HCC to Orlistate treatment (FASN inhibitor).
1. Stoiber K, Nagło O, Pernpeintner C, Zhang S, Koeberle A, Ulrich M, et al. Targeting de novo lipogenesis as a novel approach in anti-cancer therapy. Br J Cancer. (2018) 118:43–51. doi: 10.1038/bjc.2017.374
2. Svensson RU, Parker SJ, Eichner LJ, Kolar MJ, Wallace M, Brun SN, et al. Inhibition of acetyl-CoA carboxylase suppresses fatty acid synthesis and tumor growth of non-small-cell lung cancer in preclinical models. Nat Med. (2016) 22:1108–19. doi: 10.1038/nm.4181
3. Flavin R, Peluso S, Nguyen PL, Loda M. Fatty acid synthase as a potential therapeutic target in cancer. Future Oncol. (2010) 6:551–62. doi: 10.2217/fon.10.11
4. Migita T, Ruiz S, Fornari A, Fiorentino M, Priolo C, Zadra G, et al. Fatty acid synthase: a metabolic enzyme and candidate oncogene in prostate cancer. J Natl Cancer Inst. (2009) 101:519–32. doi: 10.1093/jnci/djp030
5. Carvalho MA, Zecchin KG, Seguin F, Bastos DC, Agostini M, Rangel AL, et al. Fatty acid synthase inhibition with Orlistat promotes apoptosis and reduces cell growth and lymph node metastasis in a mouse melanoma model. Int J Cancer. (2008) 123:2557–65. doi: 10.1002/ijc.23835
6. Puig T, Turrado C, Benhamú B, Aguilar H, Relat J, Ortega-Gutiérrez S, et al. Novel inhibitors of fatty acid synthase with anticancer activity. Clin Cancer Res. (2009) 15:7608–15. doi: 10.1158/1078-0432.CCR-09-0856
7. Kridel SJ, Axelrod F, Rozenkrantz N, Smith JW. Orlistat is a novel inhibitor of fatty acid synthase with antitumor activity. Cancer Res. (2004) 64:2070–5. doi: 10.1158/0008-5472.CAN-03-3645
8. Yoshii Y, Furukawa T, Oyama N, Hasegawa Y, Kiyono Y, Nishii R, et al. Fatty acid synthase is a key target in multiple essential tumor functions of prostate cancer: uptake of radiolabeled acetate as a predictor of the targeted therapy outcome. PLoS One. (2013) 8:e64570. doi: 10.1371/journal.pone.0064570
9. Rossi S, Graner E, Febbo P, Weinstein L, Bhattacharya N, Onody T, et al. Fatty acid synthase expression defines distinct molecular signatures in prostate cancer. Mol Cancer Res. (2003) 1:707–15.
10. Vâvere AL, Kridel SJ, Wheeler FB, Lewis JS. 1-11C-acetate as a PET radiopharmaceutical for imaging fatty acid synthase expression in prostate cancer. J Nucl Med. (2008) 49:327–34. doi: 10.2967/jnumed.107.046672
11. Yun M, Bang S-H, Kim JW, Park JY, Kim KS, Lee JD. The importance of acetyl coenzyme A synthetase for 11C-acetate uptake and cell survival in hepatocellular carcinoma. J Nucl Med. (2009) 50:1222–8. doi: 10.2967/jnumed.109.062703
12. Yoshimoto M, Waki A, Yonekura Y, Sadato N, Murata T, Omata N, et al. Characterization of acetate metabolism in tumor cells in relation to cell proliferation: acetate metabolism in tumor cells. Nucl Med Biol. (2001) 28:117–22. doi: 10.1016/S0969-8051(00)00195-5
13. Ho C-L, Yu SCH, Yeung DWC. 11C-Acetate PET imaging in hepatocellular carcinoma and other liver masses. J Nucl Med. (2003) 44:213–21.
14. Huang SC, Phelps ME, Hoffman EJ, Sideris K, Selin CJ, Kuhl DE. Noninvasive determination of local cerebral metabolic rate of glucose in man. Am J Physiol. (1980) 238:E69–82. doi: 10.1152/ajpendo.1980.238.1.E69
15. Phelps ME, Huang S-C, Hoffman EJ, Selin CE, Sokoloff L, Kuhl D. Tomographic measurement of local cerebral glucose metabolic rate in humans with (F-18)2-fluoro-2-deoxy-D glucose: validation of method. Ann Neurol. (1979) 6:371–88. doi: 10.1002/ana.410060502
16. Li S, Peck-Radosavljevic M, Ubl P, Wadsak W, Mitterhauser M, Rainer E, et al. The value of [(11)C]-acetate PET and [(18)F]-FDG PET in hepatocellular carcinoma before and after treatment with transarterial chemoembolization and bevacizumab. Eur J Nucl Med Mol Imaging. (2017) 44:1732–41. doi: 10.1007/s00259-017-3724-2
17. Wang R-C, Huang C-Y, Pan T-L, Chen W-Y, Ho C-T, Liu T-Z, et al. Proteomic characterization of annexin l (ANX1) and heat shock protein 27 (HSP27) as biomarkers for invasive hepatocellular carcinoma cells. PLoS One. (2015) 10:e0139232. doi: 10.1371/journal.pone.0139232
18. Pike VW, Eakins MN, Allan RM, Selwyn AP. Preparation of [1-11C]acetate–an agent for the study of myocardial metabolism by positron emission tomography. Int J Appl Radiat Isot. (1982) 33:505–12. doi: 10.1016/0020-708X(82)90003-5
19. Hamacher K, Coenen HH, Stöcklin G. Efficient stereospecific synthesis of no-carrier-added 2-[18F]-fluoro-2-deoxy-D-glucose using aminopolyether supported nucleophilic substitution. J Nucl Med. (1986) 27:235–8.
20. Loening AM, Gambhir SS. AMIDE: a free software tool for multimodality medical image analysis. Mol Imaging. (2003) 2:131–7. doi: 10.1162/153535003322556877
21. Hsu F-T, Sun C-C, Wu C-H, Lee Y-J, Chiang C-H, Wang W-S. Regorafenib induces apoptosis and inhibits metastatic potential of human bladder carcinoma cells. Anticancer Res. (2017) 37:4919–26. doi: 10.21873/anticanres.11901
22. Li L, Che L, Wang C, Blecha JE, Li X, VanBrocklin HF, et al. [(11)C]acetate PET imaging is not always associated with increased lipogenesis in hepatocellular carcinoma in mice. Mol Imaging Biol. (2016) 18:360–7. doi: 10.1007/s11307-015-0915-8
23. Zhao X, Zhao L, Yang H, Li J, Min X, Yang F, et al. Pyruvate kinase M2 interacts with nuclear sterol regulatory element–binding protein 1a and thereby activates lipogenesis and cell proliferation in hepatocellular carcinoma. J Biol Chem. (2018) 293:6623–34. doi: 10.1074/jbc.RA117.000100
24. Huang B, Law MW, Khong PL. Whole-body PET/CT scanning: estimation of radiation dose and cancer risk. Radiology. (2009) 251:166–74. doi: 10.1148/radiol.2511081300
25. Kwon HW, Kim JP, Lee HJ, Paeng JC, Lee JS, Cheon GJ, et al. Radiation dose from whole-body f-18 fluorodeoxyglucose positron emission tomography/computed tomography: nationwide survey in Korea. J Korean Med Sci. (2016) 31(Suppl. 1):S69–74. doi: 10.3346/jkms.2016.31.S1.S69
26. Alameen S, Tamam N, Awadain S, Sulieman A, Alkhaldi L, Hmed AB. Radiobiological risks in terms of effective dose and organ dose from (18)F-FDG whole-body PET/CT procedures. Saudi J Biol Sci. (2021) 28:5947–51. doi: 10.1016/j.sjbs.2021.06.055
27. Jeon JY, Lee M, Whang SH, Kim JW, Cho A, Yun M. Regulation of acetate utilization by monocarboxylate transporter 1 (MCT1) in hepatocellular carcinoma (HCC). Oncol Res. (2018) 26:71–81. doi: 10.3727/096504017X14902648894463
28. Nakagawa H, Hayata Y, Kawamura S, Yamada T, Fujiwara N, Koike K. Lipid metabolic reprogramming in hepatocellular carcinoma. Cancers. (2018) 10:447. doi: 10.3390/cancers10110447
29. Cao D, Song X, Che L, Li X, Pilo MG, Vidili G, et al. Both de novo synthetized and exogenous fatty acids support the growth of hepatocellular carcinoma cells. Liver Int. (2017) 37:80–9. doi: 10.1111/liv.13183
30. Busk M, Horsman MR, Kristjansen PEG, van der Kogel AJ, Bussink J, Overgaard J. Aerobic glycolysis in cancers: Implications for the usability of oxygen-responsive genes and fluorodeoxyglucose-PET as markers of tissue hypoxia. Int J Cancer. (2008) 122:2726–34. doi: 10.1002/ijc.23449
31. Zheng J. Energy metabolism of cancer: Glycolysis versus oxidative phosphorylation (Review). Oncol Lett. (2012) 4:1151–7. doi: 10.3892/ol.2012.928
32. Sun H-W, Yu X-J, Wu W-C, Chen J, Shi M, Zheng L, et al. GLUT1 and ASCT2 as predictors for prognosis of hepatocellular carcinoma. PLoS One. (2016) 11:e0168907. doi: 10.1371/journal.pone.0168907
33. Tang L, Wei R, Chen R, Fan G, Zhou J, Qi Z, et al. Establishment and validation of a cholesterol metabolism-related prognostic signature for hepatocellular carcinoma. Comput Struct Biotechnol J. (2022) 20:4402–14. doi: 10.1016/j.csbj.2022.07.030
34. Che L, Chi W, Qiao Y, Zhang J, Song X, Liu Y, et al. Cholesterol biosynthesis supports the growth of hepatocarcinoma lesions depleted of fatty acid synthase in mice and humans. Gut. (2020) 69:177. doi: 10.1136/gutjnl-2018-317581
35. Heravi G, Yazdanpanah O, Podgorski I, Matherly L, Liu W. Lipid metabolism reprogramming in renal cell carcinoma. CancerMetastasis Rev. (2022) 41:17–31. doi: 10.1007/s10555-021-09996-w
36. Zhou F, Sun X. Cholesterol metabolism: a double-edged sword in hepatocellular carcinoma. Front Cell Dev Biol. (2021) 9:762828. doi: 10.3389/fcell.2021.762828
37. Von Roemeling CA, Caulfield TR, Marlow L, Bok I, Wen J, Miller JL, et al. Accelerated bottom-up drug design platform enables the discovery of novel stearoyl-CoA desaturase 1 inhibitors for cancer therapy. Oncotarget. (2017) 9:3–20. doi: 10.18632/oncotarget.21545
38. Lu T, Schubert C, Cummings MD, Bignan G, Connolly PJ, Smans K, et al. Design and synthesis of a series of bioavailable fatty acid synthase (FASN) KR domain inhibitors for cancer therapy. Bioorg Med Chem Lett. (2018) 28:2159–64. doi: 10.1016/j.bmcl.2018.05.014
39. Kim S, Yoon YY, Park YW, Whang W-K, Park S-Y, Hwang KW. Cynandione A from Cynanchum wilfordii inhibits hepatic de novo lipogenesis by activating the LKB1/AMPK pathway in HepG2 cells. J Nat Med. (2020) 74:142–52. doi: 10.1007/s11418-019-01356-x
40. Wang Y, Wan X, Hao Y, Zhao Y, Du L, Huang Y, et al. NR6A1 regulates lipid metabolism through mammalian target of rapamycin complex 1 in HepG2 cells. Cell Commun Signal. (2019) 17:77. doi: 10.1186/s12964-019-0389-4
41. Zare M, Panahi G, Koushki M, Mostafavi-Pour Z, Meshkani R. Metformin reduces lipid accumulation in HepG2 cells via downregulation of miR-33b. Arch Physiol Biochem. (2019) 128:333–40. doi: 10.1080/13813455.2019.1680700
Keywords: de novo lipogenesis, imaging probe, cancer metabolism, PET/CT, cancer therapy, lipogenesis-targeted therapy, hepatocellular carcinoma
Citation: Tsao C-H, Jhou R-H, Ke C-C, Chang C-W, Chang C-W, Yang B-H, Huang W-S, Shih B-F and Liu R-S (2022) Dual-tracer positron emission tomography/computed tomography as an imaging probe of de novo lipogenesis in preclinical models of hepatocellular carcinoma. Front. Med. 9:1008200. doi: 10.3389/fmed.2022.1008200
Received: 31 July 2022; Accepted: 12 September 2022;
Published: 27 September 2022.
Edited by:
Anne Roivainen, University of Turku, FinlandReviewed by:
Sharjeel Usmani, Kuwait Cancer Control Center, KuwaitCopyright © 2022 Tsao, Jhou, Ke, Chang, Chang, Yang, Huang, Shih and Liu. This is an open-access article distributed under the terms of the Creative Commons Attribution License (CC BY). The use, distribution or reproduction in other forums is permitted, provided the original author(s) and the copyright owner(s) are credited and that the original publication in this journal is cited, in accordance with accepted academic practice. No use, distribution or reproduction is permitted which does not comply with these terms.
*Correspondence: Chien-Chih Ke, Y2NrZUBrbXUuZWR1LnR3; Ren-Shyan Liu, cnNsaXV2Z2hAZ21haWwuY29t
Disclaimer: All claims expressed in this article are solely those of the authors and do not necessarily represent those of their affiliated organizations, or those of the publisher, the editors and the reviewers. Any product that may be evaluated in this article or claim that may be made by its manufacturer is not guaranteed or endorsed by the publisher.
Research integrity at Frontiers
Learn more about the work of our research integrity team to safeguard the quality of each article we publish.