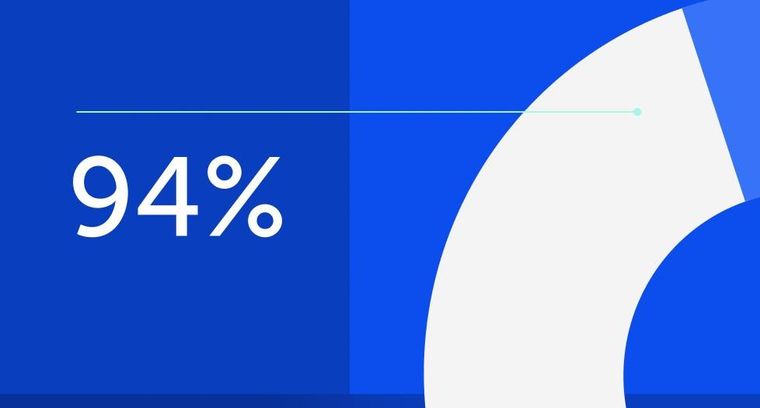
94% of researchers rate our articles as excellent or good
Learn more about the work of our research integrity team to safeguard the quality of each article we publish.
Find out more
REVIEW article
Front. Med., 17 January 2022
Sec. Nephrology
Volume 8 - 2021 | https://doi.org/10.3389/fmed.2021.790783
This article is part of the Research TopicTissue Microenvironment in Kidney DiseasesView all 7 articles
Chronic kidney disease (CKD) is an increasing global health burden. Current treatments for CKD include therapeutics to target factors that contribute to CKD progression, including renin–angiotensin–aldosterone system inhibitors, and drugs to control blood pressure and proteinuria control. Recently, associations between chronic disease processes and the human microbiota and its metabolites have been demonstrated. Dysbiosis—a change in the microbial diversity—has been observed in patients with CKD. The relationship between CKD and dysbiosis is bidirectional; gut-derived metabolites and toxins affect the progression of CKD, and the uremic milieu affects the microbiota. The accumulation of microbial metabolites and toxins is linked to the loss of kidney functions and increased mortality risk, yet renoprotective metabolites such as short-chain fatty acids and bile acids help restore kidney functions and increase the survival rate in CKD patients. Specific dietary interventions to alter the gut microbiome could improve clinical outcomes in patients with CKD. Low-protein and high-fiber diets increase the abundance of bacteria that produce short-chain fatty acids and anti-inflammatory bacteria. Fluctuations in the urinary microbiome are linked to increased susceptibility to infection and antibiotic resistance. In this review, we describe the potential role of the gut, urinary and blood microbiome in CKD pathophysiology and assess the feasibility of modulating the gut microbiota as a therapeutic tool for treating CKD.
Chronic kidney disease (CKD) is a growing healthcare burden affecting about 13.4% of the population worldwide (1). In the last few decades, the number of CKD patients has steadily increased (2). In adults, hypertension and diabetes are the leading causes of CKD, while congenital anomalies of the kidney and urogenital track account for the majority of CKD etiologies in children. Factors that contribute to the progression of CKD include activation of the renin–angiotensin–aldosterone system, proteinuria, a state of chronic inflammation and repetitive acute kidney injury (3–7). CKD is associated with the development of severe health conditions like cardiovascular diseases, neurological complications, adverse pregnancy outcomes, and hyperkalemia (8–12). In children, CKD affects neurocognitive abilities, school performance, growth, quality of life and the cost of medical care (6, 13–15).
Current treatments for CKD include renin–angiotensin–aldosterone system inhibitors and drugs to control blood pressure and proteinuria. An increasing number of studies suggest that the composition of the microbiome has a key role in maintaining health. The human microbiome is the collection of all microbial DNA in the human body, which is distributed in various body parts as; skin, gastrointestinal, urinary tract, respiratory tract, and oral cavity (16). These microbes play crucial roles in the digestion and metabolic processes, stimulation and regulation of the immune response, production of vitamins, and protection against pathogens (17, 18). This microbial community is in a symbiotic relation with the host in the healthy states (19). Dysbiosis refers to a disruption of the microbial balance, and this phenomenon is associated with various diseases and pathological conditions including CKD (20, 21).
The major part of the human microbiome is centralized in the gut (22). The gut microbiota harbors 10-fold more microbial cells than human cells, which regulates nutrient metabolism and produces various metabolites that affect the kidney, heart, vascular system, and liver (23). There is a bi-directional relationship between dysbiosis and the pathogenesis of CKD (20, 24–26). We summarized this relationship in Figure 1. It is well-established that an increase in levels of harmful metabolites including trimethylamine N-oxide (TAMO), indoxyl sulfate, and p-cresyl sulfate are associated with renal fibrosis, endothelial dysfunction, a decline in the estimated glomerular filtration rate (eGFR), cardiovascular complications, and increased mortality and morbidity in CKD (27–30). Moreover, the serum levels of 5-methoxytryptophan and indoxyl sulfate correlate positively with CKD progression (31). On the other hand, renoprotective metabolites including short-chain fatty acids prevent the progression of CKD by suppressing the disruption of the epithelial barrier and regulating the anti-inflammatory response (25, 32). The level of indole propionic acid, derived from the gut flora, negatively correlates with p-cresyl sulfate and indoxyl sulfate concentrations in CKD patients (33).
Figure 1. The relationship between the gut microbiome and chronic kidney disease (CKD) is bi-directional. In one direction, the gut microbiota affect the kidney; the emerging role of gut microbiota in (A) The healthy gut, (B) The leaky gut due to microbial dysbiosis and disruption of the mucosal layer, (C) Release of pro-inflammatory factors in the bloodstream and initiation of the inflammatory cascade, accumulation of uremic toxins, (D) A decline in the estimated glomerular filtration rate (eGFR), the elevation of the albumin creatinine ratio (ACR) and loss of the endocrine functions of the kidney. In the other direction, CKD drives dysbiosis in the gut (indicated by the dotted arrows) and initiates an inflammatory cascade.
The composition of the gut microbiota can be altered by therapeutic dietary interventions and intake of probiotics, and so dietary interventions and probiotics intake can be used to improve CKD outcomes (27, 34). A very low-protein diet decreases plasma levels of indoxyl sulfate, and p-cresyl sulfate and increases the diversity of both butyrate-forming bacteria such as Coprococcus and Roseburia, and the levels of anti-inflammatory bacteria like Blautia and Faecalibacterium (27). A high-fiber diet improves kidney function by lowering the harmful uremic metabolite levels and decreasing microbial diversity (35). Conversely, a high-fat diet increases the plasma level of gut microbiota-derived TAMO metabolites in a mouse model (36).
While the gut is the main repertoire of microbes and the most studied site to date, urine and blood also harbor different types of microbes and microbial signatures in both healthy and disease conditions (22, 37, 38). Several studies have examined the role of gut-derived metabolites in CKD, but little is known about the role of the composition of the urinary and blood microbiomes in the progression of CKD (20, 28, 39).
Our manuscript will focus on the relationship between the human microbiome and its products in the CKD pathophysiology and modulating the gut microbiota as a therapeutic strategy for CKD treatment.
A medical literature search was conducted using PubMed for articles published until May 24th, 2021. The initial search was done using the general search terms: “microbiome,” “chronic kidney disease,” and “metabolites.” Only articles published in English were included. The search resulted in a total of 143 articles. After excluding review articles, we focused on 57 studies summarized in Tables 1, 2, 3, we classified the studies based on the CKD model into clinical studies, animal studies, and dietary interventions studies. References of the included articles were also reviewed for additional relevant articles.
Table 1. Role of gut microbiota and microbial related metabolites in the pathogenesis of CKD (Clinical studies).
Table 2. Role of gut microbiota and microbial related metabolites in the pathogenesis of CKD (Animal models studies).
To sum up three studies were included in the clinical (Table 1) and animal (Table 2) studies, and one study was included in Tables 1, 3 as different CKD models have been used in these four studies. In the tables, we focused on the techniques used to assess gut microbiota and microbial related metabolites not all the techniques used in each study. Of note, gut microbiota and its related metabolites have a significant role in CKD progression and are associated with different circumstances. The exact mechanism of the gut-kidney axis is not clearly identified and both mechanisms of the role of gut microbiota in CKD (causative or consequence) are applicable. Furthermore, dietary intervention studies using animal models showed a change in the disease outcomes, but in humans did not show a big difference. This may be due to most of the participants were in a late CKD stage (ESRD or CKD on hemodialysis) in which most kidney functions are compromised.
CKD encompasses a spectrum of pathophysiologic processes associated with abnormal kidney function and a progressive decline in the glomerular filtration rate (GFR) (29). The underlying etiology varies by age, presence of co-morbid conditions, repeated occurrences of acute kidney injury and level of proteinuria (5, 6). The decline in kidney function and micro-structural changes are considered chronic when they last more than 3 months (85). Irrespective of the underlying etiology (which is considered the initiating mechanism), hyperfiltration and hypertrophy of the remaining nephrons, tubulointerstitial fibrosis, activation of the renin–angiotensin–aldosterone system, and disruption of endothelial barriers disruption are common and lead to a reduction in the renal excretion efficacy and decline in the eGFR (3, 86). The eGFR is used to grade disease severity in CKD patients, a higher grade is associated with a lower filtration rate and more advanced disease (85). The transition from one grade to the next grade is usually accompanied by a loss in the endocrine function of the kidney (86). In particular, CKD patients suffering from cardiovascular events show deterioration in renal functions and severe inflammation (87). Infiltration of immune cells in the tubulointerstitial space and accumulation of immune-derived components contribute to CKD progression (88). A key goal of CKD therapies is to prevent patients progressing to the next stage of the disease.
The dominant bacterial phyla in the gut are Firmicutes, Bacteroidetes, Actinobacteria, and Proteobacteria (89). The interplay between bacteria present in the gut (and their metabolites) and kidney function is occasionally referred to as the gut–kidney axis (90). Recent studies indicate that aberrant gut microbiota has a key role in the pathophysiology of CKD with severe CKD outcomes (24, 61). Bifidobacterium and Lactobacilli are negatively correlated with CKD progression and long-term survival (27, 34, 39). A study of 223 patients with end-stage renal disease revealed that Eggerthella lenta, Fusobacterium nucleatum, and Alistipes shahii are positively correlated with increased levels of secondary bile acids and uremic toxins in CKD patients compared with the control group (39). In this study, the authors showed that the presence of Faecalibacterium prausnitzii, Roseburia, and Prevotella (which produce short-chain fatty acids) was negatively correlated with disease progression and uremic toxin accumulation (39). Another study of 92 patients with CKD reported an increased abundance of Paraprevotella, Pseudobutyrivibrio, and Collinsella stercoris in the CKD cohort; this finding led the authors to suggest that this signature can be used to discriminate between patients with CKD (even those in the early stages of the disease) and healthy individuals (20).
Dysbiosis in the gut has an emerging role in many inflammatory-related diseases and is thought to contribute to the inflammatory component of both acute and chronic kidney disease (91, 92). Microbial alterations in the gut affects the permeability of the intestinal mucosal barrier and releases pro-inflammatory factors and endotoxins in the bloodstream, which initiate the inflammatory cascade (93).
Another mechanism by which gut dysbiosis may contribute to CKD progression is via the role of gut dysbiosis in endothelial dysfunction, the vasoconstrictor response, and the subsequent development of hypertension; a well-known risk factor for CKD (94, 95). Mice fed a high-salt diet had aberrant microbiota compared with mice fed a normal diet; these changes were associated with activation of T-lymphocytes and an elevation in blood pressure (96). A lower abundance of Lactobacillus species in the gut is associated with the development of hypertension and kidney diseases (97). Changes in the gut microbiota could be the starting point for CKD progression through a series of immune response modifications, blood pressure alterations, metabolic changes, and prolonged inflammation.
In general, microbial metabolites associated with CKD are classified into two groups; harmful and renoprotective metabolites. This bi-direction relationship of microbial-derived metabolites is illustrated in Figure 2. Several human and animal studies have demonstrated the deleterious effects of TAMO on the kidney, manifested as kidney interstitial fibrosis, eGFR decline, endothelial dysfunction, and an increased risk of cardiovascular disease risk (34, 36, 47, 81). The increased risk of mortality and morbidity in patients with CKD has been attributed to the accumulation of indoxyl sulfate and p-cresyl sulfate (27, 28, 33, 39). These toxins bind with high affinity to plasma proteins, which mitigates their removal through the dialysis membrane (28). TAMO, indoxyl sulfate, and p-cresyl sulfate are involved in SMAD signaling, tryptophan metabolism, and tyrosine pathways, respectively (20, 46, 54).
Figure 2. The bidirectional role of gut-derived metabolites in the pathophysiology of CKD; (A) Beneficial bacteria produce renoprotective metabolites that inhibit kidney damage, (B) Unfavorable bacteria produce harmful metabolites which promote kidney damage and CKD progression. Trimethylamine N-oxide (TMAO), indoxyl sulfate (IS), p-cresyl sulfate (PSC), short-chain fatty acids (SCFAs), bile acids (Bas), and Indole derivatives (IDs).
A wide array of uremic toxins and other microbial metabolites accumulate in biological samples of patients with CKD, including those in common biological samples such as plasma, stool, and urine, but also volatile metabolites in exhaled breath and gases collected from fecal cultures. For example, accumulation of gaseous metabolites including isoprene, aldehyde, dimethyldisulfide, dimethyltrisulfide, and thioesters occurs in patients with CKD (65).
Microbial dysbiosis and accumulation of gut-derived metabolites have been reported in CKD patients (20, 29, 53). Randomized controlled clinical trials in patients with CKD indicate that changes in the composition of the gut microbiota after treatment with prebiotics and probiotics improved disease outcomes and reduced uremic toxin levels (98–100). Patients with a high abundance of Bifidobacterium and Lactobacillus had lower serum levels of uremic toxins, a reduced inflammatory milieu, and improved renal function (98, 101).
The consumption of food items rich in choline and L-claritin—which are precursors of TAMO—such as egg yolk, kidney, liver, meat and milk, correlates with a high accumulation of uremic toxin and a decline in the glomerular filtration rate (102). A prospective, crossover clinical trial randomized 60 patients with CKD to different dietary interventions; the group on the very low-protein diet had an increase in the gut abundance of Actinobacteria and a decrease in the inflammatory Proteobacteria compared with the group on a regular diet (27).
Prebiotics are non-digestible dietary components such as dietary fiber and digestion-resistant starch. They are present in cereals, fruits, milk, honey, and vegetables or can be given as a dietary supplement (103). Fermentation of prebiotics beneficially modifies gut bacteria by increasing the abundance of Bifidobacterium spp and lactobacillus and reducing the levels of Bacteroides, Clostridia, and Enterobacteria (104). In patients with CKD, dietary fiber intake decreases the levels of circulating pro-inflammatory cytokines, slows the decline in eGFR, lowers the plasma levels of uremic toxins, and minimizes CKD-related cardiovascular risk (105, 106). Esgalhado and colleagues studied the effect of digestion-resistant starch supplementation (16 g/day) in patients with CKD; they observed a reduction in the plasma levels of uremic toxins (indoxyl sulfate, and p-cresyl sulfate), interleukin (IL)-6, and thiobarbituric acid-reactive substances (107). These results are consistent with another study of 32 patients with CKD randomized into two groups; the group that received lactulose syrup for 8 weeks had a greater abundance Bifidobacterium and Lactobacillus in the gut microbiome and decreased serum creatinine levels (108). While these studies demonstrated that probiotics and prebiotics have a beneficial effect on CKD, other studies have shown no significant changes in circulating gut-derived metabolites or changes in CKD outcomes (34, 84). It is important to point out that existing studies are heterogeneous; they used different dietary supplements, had varying durations of intervention, and administered to patients with other comorbidities, patients with varying kidney disease severity and varying underlying etiology. This heterogeneity makes it extremely difficult to draw conclusions from these studies. That being said, superior results may be obtained from the study of dietary interventions in children as other cofounding factors are minimal.
Overall, these studies imply that nutrition therapy has the potential to modulate the microbiome composition and its metabolites, and consequently ameliorate CKD complications and the rate of CKD progression. However, further well-designed, prospective studies are needed to definitively demonstrate the benefit of nutrition therapy on CKD.
Most of the attention in the microbiome field is on the gut microbiota and its metabolites; however, the urinary microbiome is receiving more attention. Until recently, urine was considered a sterile fluid that was only rendered unsterile because of infection (109, 110). But the development of next-generation sequencing techniques enabled studies showing that the urinary tract of healthy individuals is dominated by different kinds of microbes, and the distribution pattern of these microbes affects the health of urinary tract health (110, 111). Fluctuation in the urinary microbiome occurs in urinary tract infections and is involved in antibiotic resistance (109, 112). The urinary microbiome undergoes changes after kidney transplantation, and these modifications thought to be responsible for allograft dysfunction and increased susceptibility to infection (113, 114). In addition, the diversity in microbes in the urinary tract of patients with CKD is associated with the eGFR value (115).
The circulatory microbiome in healthy individuals contains diverse bacterial taxa, and the dominated phylum is Proteobacteria (38). Gut-derived endotoxins circulating in the bloodstream were shown to alter the blood microbiome (93). A study investigating the correlation between blood metabolome and α-diversity of gut microbiota on 399 participants indicated that gut-derived metabolites like p-cresyl and TAMO reflect the Shannon diversity of gut bacteria and could be a biomarker reflecting the gut health (116). A case-controlled study using 16S rRNA target sequencing of blood samples showed that compared with control groups, patients with CKD had a higher diversity of Enterobacteriaceae and Pseudomonadaceae, which was also correlated with lower eGFR (117). Hence, we see the gut microbiota has an ultimate effect on CKD outcomes through different routes.
Microbial dysbiosis plays an important role in the pathogenesis of various diseases. In this review, we summarized and reviewed the literature examining the dual role of the gut microbiome and its metabolic products in the pathophysiology and progression of CKD. We described how gut dysbiosis can initiate the inflammatory process and cause leaking of gut-derived metabolites into the bloodstream. It is well-established that TAMO, indoxyl sulfate, and p-cresyl sulfate and other harmful microbial metabolites accumulate in patients with CKD, and levels of these metabolites correlate with disease progression. Lower levels of bifidobacterium, lactobacillus, and bile acid composition are linked to adverse outcomes in patients with CKD. Our analysis of the literature suggests that the complex interaction between the gut, urinary tract and blood microbiota and associated metabolites may orchestrate subclinical changes in the pathogenesis of CKD and contribute to disease. Modulating the gut microbiota using dietary interventions could improve the clinical outcomes of patients with CKD. Our recommendations are i. conducting omics-based studies like metagenomics and metatranscriptomics to identify the gut microbiota community, metabolic pathways, and microbial genes associated with CKD. ii. screening gut microbiota at different disease stages, especially at the early disease stages. iii. performing dietary intervention studies for CKD patients in the early stages. iv. assessment of urinary and blood microbiome studies for CKD patients. These directions may give a clue about the disease etiology, metabolic pathways and potential treatment for CKD.
EW wrote the first draft. IS and SA reviewed and finalized the content of the manuscript. All authors read and approved the final version.
This work is funded by Sidra Medicine Internal Research Fund 2019 (No. SDR 200055).
The authors declare that the research was conducted in the absence of any commercial or financial relationships that could be construed as a potential conflict of interest.
All claims expressed in this article are solely those of the authors and do not necessarily represent those of their affiliated organizations, or those of the publisher, the editors and the reviewers. Any product that may be evaluated in this article, or claim that may be made by its manufacturer, is not guaranteed or endorsed by the publisher.
1. Lv J, Zhang L. Prevalence and disease burden of chronic kidney disease. Adv Exp Med Biol. (2019) 1165:3–15. doi: 10.1007/978-981-13-8871-2_1
2. Gorostidi M, Sánchez-Martínez M, Ruilope LM, Graciani A, de la Cruz JJ, Santamaría R, et al. Chronic kidney disease in Spain: prevalence and impact of accumulation of cardiovascular risk factors. Nefrologia. (2018) 38:606–15. doi: 10.1016/j.nefroe.2018.04.010
3. Black LM, Lever JM, Traylor AM, Chen B, Yang Z, Esman SK, et al. Divergent effects of AKI to CKD models on inflammation and fibrosis. Am J Physiol Renal Physiol. (2018) 315:F1107–18. doi: 10.1152/ajprenal.00179.2018
4. Kumar S. Cellular and molecular pathways of renal repair after acute kidney injury. Kidney Int. (2018) 93:27–40. doi: 10.1016/j.kint.2017.07.030
5. Negi S, Koreeda D, Kobayashi S, Yano T, Tatsuta K, Mima T, et al. Acute kidney injury: epidemiology, outcomes, complications, and therapeutic strategies. Semin Dial. (2018) 31:519–27. doi: 10.1111/sdi.12705
6. Tiewsoh K, Soni A, Dawman L, Peters NJ, Malik MA. Chronic peritoneal dialysis in children with chronic kidney disease: An experience from a North Indian teaching institute. J Fam Med Prim Car. (2021) 10:3682–7. doi: 10.4103/jfmpc.jfmpc_250_21
7. Kaur G, Singh J, Kumar J. Vitamin D and cardiovascular disease in chronic kidney disease. Pediatr Nephrol. (2019) 34:2509–22. doi: 10.1007/s00467-018-4088-y
8. Chang C, Fan P, Kuo G, Lin Y, Tsai T, Chang S, et al. Infection in advanced chronic kidney disease and subsequent adverse outcomes after dialysis initiation: a nationwide cohort study. Sci Rep. (2020) 10:2938. doi: 10.1038/s41598-020-59794-7
9. Sárközy M, Kovács ZZA, Kovács MG, Gáspár R, Szucs G, Dux L. Mechanisms and modulation of oxidative/nitrative stress in type 4 cardio-renal syndrome and renal sarcopenia. Front Physiol. (2018) 9:1648. doi: 10.3389/fphys.2018.01648
10. Mazumder MK, Paul R, Bhattacharya P, Borah A. Neurological sequel of chronic kidney disease: from diminished acetylcholinesterase activity to mitochondrial dysfunctions, oxidative stress and inflammation in mice brain. Sci Rep. (2019) 9:3097. doi: 10.1038/s41598-018-37935-3
11. Piccoli GB, Cabiddu G, Attini R, Vigotti FN, Maxia S, Lepori N, et al. Risk of adverse pregnancy outcomes in women with CKD. J Am Soc Nephrol. (2015) 26:2011–22. doi: 10.1681/ASN.2014050459
12. Kovesdy CP. Management of hyperkalaemia in chronic kidney disease. Nat Rev Nephrol. (2014) 10:653–62. doi: 10.1038/nrneph.2014.168
13. Chou H, Lin C, Chiou Y, Tain Y, Wang Y, Wang H, et al. Clinical characteristics and prevalence of complications of chronic kidney disease in children: the Taiwan pediatric renal collaborative study. Pediatr Nephrol. (2016) 31:1113–20. doi: 10.1007/s00467-016-3325-5
14. Ishikura K, Uemura O, Hamasaki Y, Ito S, Wada N, Hattori M, et al. Progression to end-stage kidney disease in Japanese children with chronic kidney disease: results of a nationwide prospective cohort study. Nephrol Dial Transplant. (2014) 29:878–84. doi: 10.1093/ndt/gfu012
15. Furth SL, Pierce C, Hui WF, White CA, Wong CS, Schaefer F, et al. Estimating time to ESRD in children with CKD. Am J Kidney Dis. (2018) 71:783–92. doi: 10.1053/j.ajkd.2017.12.011
16. Gilbert J, Blaser MJ, Caporaso JG, Jansson J, Lynch SV, Knight R. Current understanding of the human microbiome. Nat Med. (2018) 24:392–400. doi: 10.1038/nm.4517
17. Sommer F, Anderson JM, Bharti R, Raes J, Rosenstiel P. The resilience of the intestinal microbiota influences health and disease. Nat Rev Microbiol. (2017) 15:630–8. doi: 10.1038/nrmicro.2017.58
18. Al Khodor S, Shatat IF. Gut microbiome and kidney disease: a bidirectional relationship. Pediatr Nephrol. (2017) 32:921–31. doi: 10.1007/s00467-016-3392-7
19. Young VB. The role of the microbiome in human health and disease: an introduction for clinicians. BMJ. (2017) 356:j831. doi: 10.1136/bmj.j831
20. Wu I-W, Lin C-Y, Chang L-C, Lee C-C, Chiu C-Y, Hsu H-J, et al. Gut microbiota as diagnostic tools for mirroring disease progression and circulating nephrotoxin levels in chronic kidney disease: discovery and validation study. Int J Biol Sci. (2020) 16:420–34. doi: 10.7150/ijbs.37421
21. Pflughoeft KJ, Versalovic J. Human microbiome in health and disease. Annu Rev Pathol. (2012) 7:99–122. doi: 10.1146/annurev-pathol-011811-132421
22. Lloyd-Price J, Abu-Ali G, Huttenhower C. The healthy human microbiome. Genome Med. (2016) 8:51. doi: 10.1186/s13073-016-0307-y
23. Schugar R, Brown J. Emerging roles of flavin monooxygenase 3 in cholesterol metabolism and atherosclerosis. Curr Opin Lipidol. (2015) 26:426–31. doi: 10.1097/MOL.0000000000000215
24. Hsu C, Chang-Chien G, Lin S, Hou C, Lu P, Tain Y. Association of trimethylamine, trimethylamine n-oxide, and dimethylamine with cardiovascular risk in children with chronic kidney disease. J Clin Med. (2020) 9:336. doi: 10.3390/jcm9020336
25. Kanemitsu Y, Mishima E, Maekawa M, Matsumoto Y, Saigusa D, Yamaguchi H, et al. Comprehensive and semi-quantitative analysis of carboxyl-containing metabolites related to gut microbiota on chronic kidney disease using 2-picolylamine isotopic labeling LC-MS/MS. Sci Rep. (2019) 9:19075. doi: 10.1038/s41598-019-55600-1
26. Mishima E, Fukuda S, Mukawa C, Yuri A, Kanemitsu Y, Matsumoto Y, et al. Evaluation of the impact of gut microbiota on uremic solute accumulation by a CE-TOFMS-based metabolomics approach. Kidney Int. (2017) 92:634–45. doi: 10.1016/j.kint.2017.02.011
27. Di Iorio BR, Rocchetti MT, De Angelis M, Cosola C, Marzocco S, Di Micco L, et al. Nutritional therapy modulates intestinal microbiota and reduces serum levels of total and free indoxyl sulfate and p-cresyl sulfate in chronic kidney disease (medika study). J Clin Med. (2019) 8:1424. doi: 10.3390/jcm8091424
28. Gryp T, De Paepe K, Vanholder R, Kerckhof F, Van Biesen W, Van de Wiele T, et al. Gut microbiota generation of protein-bound uremic toxins and related metabolites is not altered at different stages of chronic kidney disease. Kidney Int. (2020) 97:1230–42. doi: 10.1016/j.kint.2020.01.028
29. Pelletier CC, Croyal M, Ene L, Aguesse A, Billon-Crossouard S, Krempf M, et al. Elevation of trimethylamine-N-oxide in chronic kidney disease: contribution of decreased glomerular filtration rate. Toxins. (2019) 11:635. doi: 10.3390/toxins11110635
30. Querfeld U, Anarat A, Bayazit AK, Bakkaloglu AS, Bilginer Y, Caliskan S, et al. The cardiovascular comorbidity in children with chronic kidney disease (4C) study: objectives, design, and methodology. Clin J Am Soc Nephrol. (2010) 5:1642–8. doi: 10.2215/CJN.08791209
31. Chen D, Cao G, Chen H, Argyopoulos CP, Yu H, Su W, et al. Identification of serum metabolites associating with chronic kidney disease progression and anti-fibrotic effect of 5-methoxytryptophan. Nat Commun. (2019) 10:1476. doi: 10.1038/s41467-019-09329-0
32. Maslowski KM, Vieira AT, Ng A, Kranich J, Sierro F, Yu D, et al. Regulation of inflammatory responses by gut microbiota and chemoattractant receptor GPR43. Nature. (2009) 461:1282–6. doi: 10.1038/nature08530
33. Sun C, Lin C, Pan H, Lee C, Lu S, Hsieh Y, et al. Clinical association between the metabolite of healthy gut microbiota, 3-indolepropionic acid and chronic kidney disease. Clin Nutr. (2019) 38:2945–8. doi: 10.1016/j.clnu.2018.11.029
34. Borges NA, Stenvinkel P, Bergman P, Qureshi AR, Lindholm B, Moraes C, et al. Effects of probiotic supplementation on trimethylamine-N-oxide plasma levels in hemodialysis patients: a pilot study. Probiotics Antimicrob Proteins. (2019) 11:648–54. doi: 10.1007/s12602-018-9411-1
35. Kieffer DA, Piccolo BD, Vaziri ND, Liu S, Lau WL, Khazaeli M, et al. Resistant starch alters gut microbiome and metabolomic profiles concurrent with amelioration of chronic kidney disease in rats. Am J Physiol Renal Physiol. (2016) 310:857. doi: 10.1152/ajprenal.00513.2015
36. Sun G, Yin Z, Liu N, Bian X, Yu R, Su X, et al. Gut microbial metabolite TMAO contributes to renal dysfunction in a mouse model of diet-induced obesity. Biochem Biophys Res Commun. (2017) 493:964–70. doi: 10.1016/j.bbrc.2017.09.108
37. Modena BD, Milam R, Harrison F, Cheeseman JA, Abecassis MM, Friedewald JJ, et al. Changes in urinary microbiome populations correlate in kidney transplants with interstitial fibrosis and tubular atrophy documented in early surveillance biopsies. Am J Transplant. (2017) 17:712–23. doi: 10.1111/ajt.14038
38. Païssé S, Valle C, Servant F, Courtney M, Burcelin R, Amar J, et al. Comprehensive description of blood microbiome from healthy donors assessed by 16S targeted metagenomic sequencing. Transfusion. (2016) 56:1138–47. doi: 10.1111/trf.13477
39. Wang X, Yang S, Li S, Zhao L, Hao Y, Qin J, et al. Aberrant gut microbiota alters host metabolome and impacts renal failure in humans and rodents. Gut. (2020). doi: 10.1136/gutjnl-2019-319766
40. Ma X, Wang Y, Wu H, Li F, Feng X, Xie Y, et al. Periodontal health related-inflammatory and metabolic profiles of patients with end-stage renal disease: potential strategy for predictive, preventive, and personalized medicine. EPMA. (2021)12:1–12. doi: 10.1007/s13167-021-00239-0
41. Ebert T, Painer J, Bergman P, Qureshi AR, Giroud S, Stalder G, et al. Insights in the regulation of trimetylamine N-oxide production using a comparative biomimetic approach suggest a metabolic switch in hibernating bears. Sci Rep. (2020) 10:20323. doi: 10.1038/s41598-020-76346-1
42. Kim JE, Kim H, Park JI, Cho H, Kwak M, Kim B, et al. The Association between Gut Microbiota and Uremia of Chronic Kidney Disease. Microorganisms. (2020) 8. doi: 10.3390/microorganisms8060907
43. Zhu B, Shen J, Jiang R, Jin L, Zhan G, Liu J, et al. Abnormalities in gut microbiota and serum metabolites in hemodialysis patients with mild cognitive decline: a single-center observational study. Psychopharmacology. (2020) 237:2739–52. doi: 10.1007/s00213-020-05569-x
44. Wu I-W, Gao S-S, Chou H-C, Yang H-Y, Chang L-C, Kuo Y-L, et al. Integrative metagenomic and metabolomic analyses reveal severity-specific signatures of gut microbiota in chronic kidney disease. Theranostics. (2020) 10:5398–411. doi: 10.7150/thno.41725
45. Hsu C, Lu P, Hou C, Tain Y. Blood pressure abnormalities associated with gut microbiota-derived short chain fatty acids in children with congenital anomalies of the kidney and urinary tract. J Clin Med. (2019) 8:1090. doi: 10.3390/jcm8081090
46. El-Deeb OS, Atef MM, Hafez YM. The interplay between microbiota-dependent metabolite trimethylamine N-oxide, transforming growth factor β/SMAD signaling and inflammasome activation in chronic kidney disease patients: a new mechanistic perspective. J Cell Biochem. (2019) 120:14476–85. doi: 10.1002/jcb.28707
47. Hsu C, Lu P, Lo M, Lin I-, Chang-Chien G, Lin S, et al. Gut microbiota-dependent trimethylamine N-Oxide pathway associated with cardiovascular risk in children with early-stage chronic kidney disease. Int J Mol Sci. (2018) 19:3699. doi: 10.3390/ijms19123699
48. Gruppen EG, Garcia E, Connelly MA, Jeyarajah EJ, Otvos JD, Bakker SJL, et al. TMAO is associated with mortality: impact of modestly impaired renal function. Sci Rep. (2017) 7:13781. doi: 10.1038/s41598-017-13739-9
49. Ottiger M, Nickler M, Steuer C, Odermatt J, Huber A, Christ-Crain M, et al. Trimethylamine-N-oxide (TMAO) predicts fatal outcomes in community-acquired pneumonia patients without evident coronary artery disease. Eur J Intern Med. (2016) 36:67–73. doi: 10.1016/j.ejim.2016.08.017
50. Poesen R, Claes K, Evenepoel P, de Loor H, Augustijns P, Kuypers D, et al. Microbiota-derived phenylacetylglutamine associates with overall mortality and cardiovascular disease in patients with CKD. J Am Soc Nephrol. (2016) 27:3479–87. doi: 10.1681/ASN.2015121302
51. Poesen R, Evenepoel P, de Loor H, Bammens B, Claes K, Sprangers B, et al. The influence of renal transplantation on retained microbial-human co-metabolites. Nephrol Dial Transplant. (2016) 31:1721–9. doi: 10.1093/ndt/gfw009
52. Mafune A, Iwamoto T, Tsutsumi Y, Nakashima A, Yamamoto I, Yokoyama K, et al. Associations among serum trimethylamine-N-oxide (TMAO) levels, kidney function and infarcted coronary artery number in patients undergoing cardiovascular surgery: a cross-sectional study. Clin Exp Nephrol. (2016) 20:731–9. doi: 10.1007/s10157-015-1207-y
53. Poesen R, Windey K, Neven E, Kuypers D, De Preter V, Augustijns P, et al. The influence of CKD on colonic microbial metabolism. J Am Soc Nephrol. (2016) 27:1389–99. doi: 10.1681/ASN.2015030279
54. Tang WHW, Wang Z, Kennedy DJ, Wu Y, Buffa JA, Agatisa-Boyle B, et al. Gut microbiota-dependent trimethylamine N-oxide (TMAO) pathway contributes to both development of renal insufficiency and mortality risk in chronic kidney disease. Circ Res. (2015) 116:448–55. doi: 10.1161/CIRCRESAHA.116.305360
55. Wikoff WR, Nagle MA, Kouznetsova VL, Tsigelny IF, Nigam SK. Untargeted metabolomics identifies enterobiome metabolites and putative uremic toxins as substrates of organic anion transporter 1 (Oat1). J Proteome Res. (2011) 10:2842–51. doi: 10.1021/pr200093w
56. Sun C, Li J, Wang Y, Lin S, Ou Y, Lin C, et al. Indoxyl sulfate caused behavioral abnormality and neurodegeneration in mice with unilateral nephrectomy. Aging. (2021) 13:6681–701. doi: 10.18632/aging.202523
57. Hu X, Xie Y, Xiao Y, Zeng W, Gong Z, Du J. Longitudinal analysis of fecal microbiome and metabolome during renal fibrotic progression in a unilateral ureteral obstruction animal model. Eur J Pharmacol. (2020) 886:173555. doi: 10.1016/j.ejphar.2020.173555
58. Hsu C, Yang H, Hou C, Chang-Chien G, Lin S, Tain Y. Maternal adenine-induced chronic kidney disease programs hypertension in adult male rat offspring: implications of nitric oxide and gut microbiome derived metabolites. Int J Mol Sci. (2020) 21:7237. doi: 10.3390/ijms21197237
59. Mishima E, Ichijo M, Kawabe T, Kikuchi K, Akiyama Y, Toyohara T, et al. Germ-free conditions modulate host purine metabolism, exacerbating adenine-induced kidney damage. Toxins. (2020) 12:547. doi: 10.3390/toxins12090547
60. Bush KT, Singh P, Nigam SK. Gut-derived uremic toxin handling in vivo requires OAT-mediated tubular secretion in chronic kidney disease. JCI Insight. (2020) 5:e133817. doi: 10.1172/jci.insight.133817
61. Feng Y, Cao G, Chen D, Vaziri ND, Chen L, Zhang J, et al. Microbiome-metabolomics reveals gut microbiota associated with glycine-conjugated metabolites and polyamine metabolism in chronic kidney disease. Cell Mol Life Sci. (2019) 76:4961–78. doi: 10.1007/s00018-019-03155-9
62. Chen L, Chen D, Liu J, Zhang J, Vaziri ND, Zhuang S, et al. Unilateral ureteral obstruction causes gut microbial dysbiosis and metabolome disorders contributing to tubulointerstitial fibrosis. Exp Mol Med. (2019) 51:1–18. doi: 10.1038/s12276-019-0234-2
63. Tao J, Zhao M, Jiang S, Pu X, Wei X. Comparative metabolism of two major compounds in Fructus Corni extracts by gut microflora from normal and chronic nephropathy rats in vitro by UPLC-Q-TOF/MS. J Chromatogr B Analyt Technol Biomed Life Sci. (2018) 1073:170–6. doi: 10.1016/j.jchromb.2017.12.025
64. Du L, Tao J, Jiang S, Qian D, Guo J, Duan J. Metabolic profiles of the flos abelmoschus manihot extract by intestinal bacteria from the normal and CKD model rats based on UPLC-Q-TOF/MS. Biomed Chromatogr. (2017) 31:e3795. doi: 10.1002/bmc.3795
65. Meinardi S, Jin K, Barletta B, Blake DR, Vaziri ND. Exhaled breath and fecal volatile organic biomarkers of chronic kidney disease. Biochim Biophys Acta. (2013) 1830:2531–7. doi: 10.1016/j.bbagen.2012.12.006
66. Chaves LD, Abyad S, Honan AM, Bryniarski MA, McSkimming DI, Stahura CM, et al. Unconjugated p-cresol activates macrophage macropinocytosis leading to increased LDL uptake. JCI Insight. (2021) 6:e144410. doi: 10.1172/jci.insight.144410
67. Zhang Z, Yang L, Wan Y, Liu C, Jiang S, Shang E, et al. Integrated gut microbiota and fecal metabolomics reveal the renoprotective effect of rehmanniae radix preparata and corni fructus on adenine-induced CKD rats. J Chromatogr B Analyt Technol Biomed Life Sci. (2021) 1174:122728. doi: 10.1016/j.jchromb.2021.122728
68. Liu Y, Li YJ, Loh YW, Singer J, Zhu W, Macia L, et al. Fiber derived microbial metabolites prevent acute kidney injury through G-Protein coupled receptors and HDAC inhibition. Front Cell Dev Biol. (2021) 9:648639. doi: 10.3389/fcell.2021.648639
69. de Andrade LS, Sardá FAH, Pereira NBF, Teixeira RR, Rodrigues SD, de Lima JD, et al. Effect of unripe banana flour on gut-derived uremic toxins in individuals undergoing peritoneal dialysis: a randomized, double-blind, placebo-controlled, crossover trial. Nutrients. (2021) 13:646. doi: 10.3390/nu13020646
70. Zhang W, Miikeda A, Zuckerman J, Jia X, Charugundla S, Zhou Z, et al. Inhibition of microbiota-dependent TMAO production attenuates chronic kidney disease in mice. Sci Rep. (2021) 11:518. doi: 10.1038/s41598-020-80063-0
71. Hsu C, Hou C, Chang-Chien G, Lin S, Yang H, Tain Y. Perinatal resveratrol therapy prevents hypertension programmed by maternal chronic kidney disease in adult male offspring: implications of the gut microbiome and their metabolites. Biomedicines. (2020) 8:567. doi: 10.3390/biomedicines8120567
72. Karaduta O, Glazko G, Dvanajscak Z, Arthur J, Mackintosh S, Orr L, et al. Resistant starch slows the progression of CKD in the 5/6 nephrectomy mouse model. Physiol Rep. (2020) 8:e14610. doi: 10.14814/phy2.14610
73. Wu I-W, Lee C-C, Hsu H-J, Sun C-Y, Chen Y-C, Yang K-J, et al. Compositional and functional adaptations of intestinal microbiota and related metabolites in CKD patients receiving dietary protein restriction. Nutrients. (2020) 12:2799. doi: 10.3390/nu12092799
74. Ephraim E, Jewell DE. Effect of added dietary betaine and soluble fiber on metabolites and fecal microbiome in dogs with early renal disease. Metabolites. (2020) 10:370. doi: 10.3390/metabo10090370
75. Nagayama Y, Isoo N, Nakashima A, Suzuki K, Yamano M, Nariyama T, et al. Renoprotective effects of paramylon, a β-1,3-D-Glucan isolated from Euglena gracilis Z in a rodent model of chronic kidney disease. PLoS ONE. (2020) 15:e0237086. doi: 10.1371/journal.pone.0237086
76. Hall JA, Jackson MI, Jewell DE, Ephraim E. Chronic kidney disease in cats alters response of the plasma metabolome and fecal microbiome to dietary fiber. PLoS ONE. (2020) 15:e0235480. doi: 10.1371/journal.pone.0235480
77. Hsu C-N, Lin I-C, Yu H-R, Huang L-T, Tiao M-M, Tain Y-L. Maternal tryptophan supplementation protects adult rat offspring against hypertension programmed by maternal chronic kidney disease: implication of tryptophan-metabolizing microbiome and aryl hydrocarbon receptor. Int J Mol Sci. (2020) 21:4552. doi: 10.3390/ijms21124552
78. Sato E, Hosomi K, Sekimoto A, Mishima E, Oe Y, Saigusa D, et al. Effects of the oral adsorbent AST-120 on fecal p-cresol and indole levels and on the gut microbiota composition. Biochem Biophys Res Commun. (2020) 525:773–9. doi: 10.1016/j.bbrc.2020.02.141
79. Gupta N, Buffa JA, Roberts AB, Sangwan N, Skye SM, Li L, et al. Targeted inhibition of gut microbial trimethylamine N-Oxide production reduces renal tubulointerstitial fibrosis and functional impairment in a murine model of chronic kidney disease. Arterioscler Thromb Vasc Biol. (2020) 40:1239–55. doi: 10.1161/ATVBAHA.120.314139
80. Cai H, Su S, Li Y, Zhu Z, Guo J, Zhu Y, et al. Danshen can interact with intestinal bacteria from normal and chronic renal failure rats. Biomed Pharmacother. (2019) 109:1758–71. doi: 10.1016/j.biopha.2018.11.047
81. Li T, Gua C, Wu B, Chen Y. Increased circulating trimethylamine N-oxide contributes to endothelial dysfunction in a rat model of chronic kidney disease. Biochem Biophys Res Commun. (2018) 495:2071–7. doi: 10.1016/j.bbrc.2017.12.069
82. Kikuchi M, Ueno M, Itoh Y, Suda W, Hattori M. Uremic toxin-producing gut microbiota in rats with chronic kidney disease. Nephron. (2017) 135:51–60. doi: 10.1159/000450619
83. Wu B, Jiang H, He Q, Wang M, Xue J, Liu H, et al. Liquid chromatography/mass spectrometry reveals the effect of lactobacillus treatment on the faecal metabolite profile of rats with chronic renal failure. Nephron. (2017) 135:156–66. doi: 10.1159/000452453
84. Poesen R, Evenepoel P, de Loor H, Delcour JA, Courtin CM, Kuypers D, et al. The influence of prebiotic arabinoxylan oligosaccharides on microbiota derived uremic retention solutes in patients with chronic kidney disease: a randomized controlled trial. PLoS ONE. (2016) 11:e0153893. doi: 10.1371/journal.pone.0153893
85. Stevens PE, Levin A. Evaluation and management of chronic kidney disease: synopsis of the kidney disease: improving global outcomes 2012 clinical practice guideline. Ann Intern Med. (2013) 158:825–30. doi: 10.7326/0003-4819-158-11-201306040-00007
86. Hodgkins KS, Schnaper HW. Tubulointerstitial injury and the progression of chronic kidney disease. Pediatr Nephrol. (2012) 27:901–9. doi: 10.1007/s00467-011-1992-9
87. Vanholder R, Van Laecke S, Glorieux G, Verbeke F, Castillo-Rodriguez E, Ortiz A. Deleting death and dialysis: conservative care of cardio-vascular risk and kidney function loss in chronic kidney disease (CKD). Toxins. (2018) 10:237. doi: 10.3390/toxins10060237
88. Rapa SF, Di Iorio BR, Campiglia P, Heidland A, Marzocco S. Inflammation and oxidative stress in chronic kidney disease-potential therapeutic role of minerals, vitamins and plant-derived metabolites. Int J Mol Sci. (2019) 21:263. doi: 10.3390/ijms21010263
89. Crespo-Salgado J, Vehaskari VM, Stewart T, Ferris M, Zhang Q, Wang G, et al. Intestinal microbiota in pediatric patients with end stage renal disease: a midwest pediatric nephrology consortium study. Microbiome. (2016) 4:50. doi: 10.1186/s40168-016-0195-9
90. Yang T, Richards EM, Pepine CJ, Raizada MK. The gut microbiota and the brain-gut-kidney axis in hypertension and chronic kidney disease. Nat Rev Nephrol. (2018) 14:442–56. doi: 10.1038/s41581-018-0018-2
91. Andrade-Oliveira V, Foresto-Neto O, Watanabe IKM, Zatz R, Câmara NOS. Inflammation in renal diseases: new and old players. Front Pharmacol. (2019) 10:1192. doi: 10.3389/fphar.2019.01192
92. Mihai S, Codrici E, Popescu ID, Enciu A, Albulescu L, Necula LG, et al. Inflammation-related mechanisms in chronic kidney disease prediction, progression, and outcome. J Immunol Res. (2018) 2018:2180373. doi: 10.1155/2018/2180373
93. Velmurugan G, Dinakaran V, Rajendhran J, Swaminathan K. Blood microbiota and circulating microbial metabolites in diabetes and cardiovascular disease. Trends Endocrinol Metab. (2020) 31:835–47. doi: 10.1016/j.tem.2020.01.013
94. Schiffrin EL. How structure, mechanics, and function of the vasculature contribute to blood pressure elevation in hypertension. Can J Cardiol. (2020) 36:648–58. doi: 10.1016/j.cjca.2020.02.003
95. Relman DA. Microbiota: a high-pressure situation for bacteria. Nature. (2017) 551:571–2. doi: 10.1038/nature24760
96. Wilck N, Matus MG, Kearney SM, Olesen SW, Forslund K, Bartolomaeus H, et al. Salt-responsive gut commensal modulates TH17 axis and disease. Nature. (2017) 551:585–9. doi: 10.1038/nature24628
97. Ghosh TS, Arnoux J, O'Toole PW. Metagenomic analysis reveals distinct patterns of gut lactobacillus prevalence, abundance, and geographical variation in health and disease. Gut Microbes. (2020) 12:1–19. doi: 10.1080/19490976.2020.1822729
98. Rossi M, Johnson DW, Morrison M, Pascoe EM, Coombes JS, Forbes JM, et al. Synbiotics easing renal failure by improving gut microbiology (SYNERGY): a randomized trial. Clin J Am Soc Nephrol. (2016) 11:223–31. doi: 10.2215/CJN.05240515
99. Cruz-Mora J, Martínez-Hernández NE, Martín del Campo-López F, Viramontes-Hörner D, Vizmanos-Lamotte B, Muñoz-Valle JF, et al. Effects of a symbiotic on gut microbiota in Mexican patients with end-stage renal disease. J Ren Nutr. (2014) 24:330–5. doi: 10.1053/j.jrn.2014.05.006
100. Guida B, Germanò R, Trio R, Russo D, Memoli B, Grumetto L, et al. Effect of short-term synbiotic treatment on plasma p-cresol levels in patients with chronic renal failure: a randomized clinical trial. Nutr Metab Cardiovasc Dis. (2014) 24:1043–9. doi: 10.1016/j.numecd.2014.04.007
101. Lopes RCSO, Balbino KP, Jorge MDP, Ribeiro AQ, Martino HSD, Alfenas RCG. Modulation of intestinal microbiota, control of nitrogen products and inflammation by pre/probiotics in chronic kidney disease: a systematic review. Nutr Hosp. (2018) 35:722–30. doi: 10.20960/nh.1642
102. Fernandez-Prado R, Esteras R, Perez-Gomez MV, Gracia-Iguacel C, Gonzalez-Parra E, Sanz AB, et al. Nutrients turned into toxins: microbiota modulation of nutrient properties in chronic kidney disease. Nutrients. (2017) 9:489. doi: 10.3390/nu9050489
103. Mafra D, Borges N, Alvarenga L, Esgalhado M, Cardozo L, Lindholm B, et al. Dietary components that may influence the disturbed gut microbiota in chronic kidney disease. Nutrients. (2019) 11:496. doi: 10.3390/nu11030496
104. Ramezani A, Raj DS. The gut microbiome, kidney disease, and targeted interventions. J Am Soc Nephrol. (2014) 25:657–70. doi: 10.1681/ASN.2013080905
105. Lu L, Huang Y, Wang M, Chen D, Wan H, Wei L, et al. Dietary fiber intake is associated with chronic kidney disease (CKD) progression and cardiovascular risk, but not protein nutritional status, in adults with CKD. Asia Pac J Clin Nutr. (2017) 26:598–605. doi: 10.6133/apjcn.072016.08
106. Camerotto C, Cupisti A, D'Alessandro C, Muzio F, Gallieni M. Dietary fiber and gut microbiota in renal diets. Nutrients. (2019) 1:2149. doi: 10.3390/nu11092149
107. Esgalhado M, Kemp JA, Azevedo R, Paiva BR, Stockler-Pinto MB, Dolenga CJ, et al. Could resistant starch supplementation improve inflammatory and oxidative stress biomarkers and uremic toxins levels in hemodialysis patients? a pilot randomized controlled trial. Food Funct. (2018) 9:6508–16. doi: 10.1039/C8FO01876F
108. Tayebi-Khosroshahi H, Habibzadeh A, Niknafs B, Ghotaslou R, Yeganeh Sefidan F, Ghojazadeh M, et al. The effect of lactulose supplementation on fecal microflora of patients with chronic kidney disease; a randomized clinical trial. J Renal Inj Prev. (2016) 5:162–7. doi: 10.15171/jrip.2016.34
109. Whiteside SA, Razvi H, Dave S, Reid G, Burton JP. The microbiome of the urinary tract–a role beyond infection. Nat Rev Urol. (2015) 12:81–90. doi: 10.1038/nrurol.2014.361
110. Morand A, Cornu F, Dufour J, Tsimaratos M, Lagier J, Raoult D. Human bacterial repertoire of the urinary tract: a potential paradigm shift. J Clin Microbiol. (2019) 57:675. doi: 10.1128/JCM.00675-18
111. Gerges-Knafl D, Pichler P, Zimprich A, Hotzy C, Barousch W, Lang RM, et al. The urinary microbiome shows different bacterial genera in renal transplant recipients and non-transplant patients at time of acute kidney injury - a pilot study. BMC Nephrol. (2020) 21:117. doi: 10.1186/s12882-020-01773-1
112. Rani A, Ranjan R, McGee HS, Andropolis KE, Panchal DV, Hajjiri Z, et al. Urinary microbiome of kidney transplant patients reveals dysbiosis with potential for antibiotic resistance. Transl Res. (2017) 181:59–70. doi: 10.1016/j.trsl.2016.08.008
113. Wu JF, Muthusamy A, Al-Ghalith GA, Knights D, Guo B, Wu B, et al. Urinary microbiome associated with chronic allograft dysfunction in kidney transplant recipients. Clin Transplant. (2018) 32:e13436. doi: 10.1111/ctr.13436
114. Schreiber PW, Kufner V, Hübel K, Schmutz S, Zagordi O, Kaur A, et al. Metagenomic Virome sequencing in living donor and recipient kidney transplant pairs revealed JC polyomavirus transmission. Clin Infect Dis. (2019) 69:987–94. doi: 10.1093/cid/ciy1018
115. Kramer H, Kuffel G, Thomas-White K, Wolfe AJ, Vellanki K, Leehey DJ, et al. Diversity of the midstream urine microbiome in adults with chronic kidney disease. Int Urol Nephrol. (2018) 50:1123–30. doi: 10.1007/s11255-018-1860-7
116. Wilmanski T, Rappaport N, Earls JC, Magis AT, Manor O, Lovejoy J, et al. Blood metabolome predicts gut microbiome α-diversity in humans. Nat Biotechnol. (2019) 37:1217–28. doi: 10.1038/s41587-019-0233-9
Keywords: chronic kidney disease, gut microbiota, urinary microbiome, dysbiosis, uremic toxins, renoprotective, diet therapy
Citation: Wehedy E, Shatat IF and Al Khodor S (2022) The Human Microbiome in Chronic Kidney Disease: A Double-Edged Sword. Front. Med. 8:790783. doi: 10.3389/fmed.2021.790783
Received: 07 October 2021; Accepted: 20 December 2021;
Published: 17 January 2022.
Edited by:
Dong Zhou, University of Connecticut, United StatesReviewed by:
Yanjiao Zhou, UCONN Health, United StatesCopyright © 2022 Wehedy, Shatat and Al Khodor. This is an open-access article distributed under the terms of the Creative Commons Attribution License (CC BY). The use, distribution or reproduction in other forums is permitted, provided the original author(s) and the copyright owner(s) are credited and that the original publication in this journal is cited, in accordance with accepted academic practice. No use, distribution or reproduction is permitted which does not comply with these terms.
*Correspondence: Souhaila Al Khodor, c2Fsa2hvZG9yQHNpZHJhLm9yZw==
Disclaimer: All claims expressed in this article are solely those of the authors and do not necessarily represent those of their affiliated organizations, or those of the publisher, the editors and the reviewers. Any product that may be evaluated in this article or claim that may be made by its manufacturer is not guaranteed or endorsed by the publisher.
Research integrity at Frontiers
Learn more about the work of our research integrity team to safeguard the quality of each article we publish.