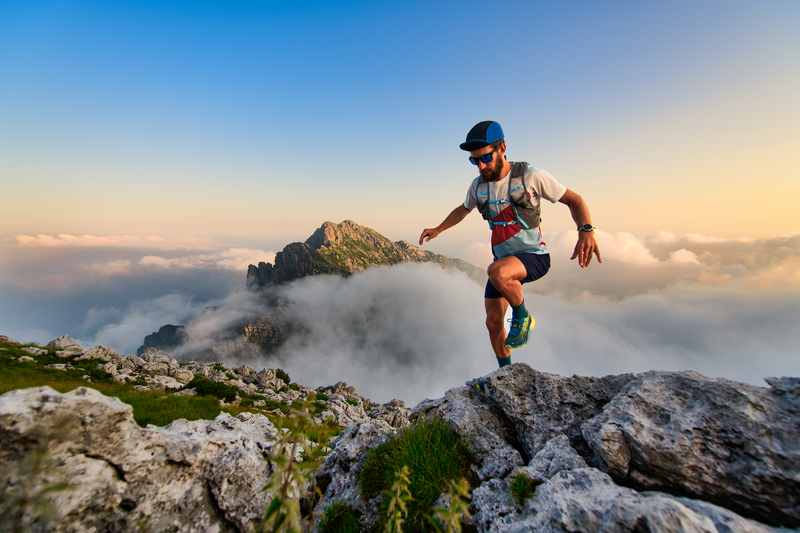
94% of researchers rate our articles as excellent or good
Learn more about the work of our research integrity team to safeguard the quality of each article we publish.
Find out more
ORIGINAL RESEARCH article
Front. Med. , 23 December 2021
Sec. Ophthalmology
Volume 8 - 2021 | https://doi.org/10.3389/fmed.2021.788663
This article is part of the Research Topic Novel Diagnostic and Therapeutic Strategies for Retinal Diseases View all 42 articles
Background and Objective: Retinal ischemia-reperfusion (IR) leads to massive loss of retinal ganglion cells (RGC) and characterizes several blind-causing ophthalmic diseases. However, the mechanism related to retinal IR is controversial, and a drug that could prevent the RGC loss caused by IR is still lacking. This study aimed to investigate the role of endogenous retinal peroxisome proliferator-activated receptor (PPAR)α and the therapeutic effect of its agonist, fenofibric acid (FA), in IR-related retinopathy.
Materials and Methods: Fenofibric acid treatment was applied to the Sprague–Dawley rats with IR and retinal cell line 28 cells with oxygen-glucose deprivation (OGD) (an in vitro model of IR). Western blotting, real-time PCR, and immunofluorescence were used to examine the expression levels of PPARα, glial fibrillary acidic protein (GFAP), and cyclooxygenase-2 (COX2). Hematoxylin and eosin (HE) staining, propidium iodide (PI) staining, retrograde tracing, and flash visual-evoked potential (FVEP) were applied to assess RGC injury and visual function.
Results: Retinal IR down-regulated PPARα expression in vitro and in vivo. Peroxisome proliferator-activated receptor α activation by FA promoted survival of RGCs, mitigated thinning of the ganglion cell complex, and decreased the latency of positive waves of FVEPs after IR injury. Further, FA treatment enhanced the expression of endogenous PPARα and suppressed the expression of GFAP and COX2 significantly.
Conclusion: Peroxisome proliferator-activated receptor α activation by FA is protective against RGC loss in retinal IR condition, which may occur by restoring PPARα expression, inhibiting activation of glial cells, and suppressing retinal inflammation. All these findings indicate the translational potential of FA in treating IR-related retinopathy.
Retinal ganglion cells (RGCs) are the only retinal neurons that directly project their axons to the central nervous system and perform visual function (1). Many retinal diseases such as acute angle-closure glaucoma, retinal vascular occlusions, and anterior ischemic optic neuropathy can directly or indirectly lead to the irreversible death of RGCs and severely threaten eyesight (2–4). The common pathologic feature among these diseases is retinal ischemia/reperfusion (IR). However, the precise pathways and molecular mechanism related to retinal IR are not well-understood and are controversial. A therapeutic drug to prevent RGC death caused by IR is lacking (5). Accordingly, understanding the pathological mechanism of retinal IR and developing effective therapy are imperative.
Peroxisome proliferator-activated receptor (PPAR)α is a ligand-activated transcription factor and member of the nuclear receptor superfamily. It plays an important part in regulation of lipid metabolism and has anti-inflammatory and antioxidant effects under several pathologic conditions (6–9). In the retina, PPARα is expressed in multiple cell types, including the retinal pigment epithelium (RPE), outer nuclear layer (ONL), inner nuclear layer (INL), and ganglion cell layer (GCL) (10), which is essential for lipid metabolism and neuronal survival in the retina (11).
Previous studies have demonstrated that PPARα expression is down-regulated in the retinas of patients suffering from diabetes mellitus (DM), as well as in the retinas of rodents with diabetic retinopathy or oxygen-induced proliferative retinopathy (10, 12). Knockout of PPARα expression aggravates retinal microvascular damage (12), overexpression of PPARα reduces retinal vascular leakage and retinal inflammation caused by diabetes (10), and alleviates retinal neovascularization in diabetic retinopathy (13). Moreover, Fenofibrate (a specific agonist of PPARα) can alleviate retinal damage by reducing apoptosis of capillary pericytes via amelioration of retinal inflammation and oxidative stress (14–16). Qiu et al. showed that PPARα activation by fenofibrate displayed therapeutic effects on age-related macular degeneration induced by lasers in rodents (17).
Taken together, these studies suggest that PPARα is a potential therapeutic target for ophthalmic diseases. However, the relationship between PPARα and ophthalmic diseases characterized by retinal IR is unclear. This study investigated whether PPARα is involved in IR-induced retinal injury (by increasing the intraocular pressure to 110 mmHg for 1 h via a saline-perfusion system). We also identified whether PPARα activation has protective effects on RGCs in this condition and explored the underlying mechanism of action.
Experiments were undertaken in accordance with the Guide for the Care and Use of Laboratory Animals (National Institutes of Health, No. 80-23, Bethesda, MD, USA). The study protocol was approved by the Animal Research Committee of the Xiangya School of Medicine (Changsha City, China).
Female Sprague–Dawley rats (200–250 g; 8 weeks; Slaccas, Changsha, China) were housed in an environment with free access to food and water under a 12-h light–dark cycle. In all procedures, rats were anesthetized with a solution of 2% sodium pentobarbital (80 mg/kg, i.p.; Sanshu, Beijing, China) and xylazine (10 mg/kg, i.p.; Huamu, Beijing, China). Oxybuprocaine hydrochloride (Santen Pharmaceuticals, Tokyo, Japan) was used to anesthetize corneas and tropicamide phenylephrine (Santen Pharmaceuticals) was used to dilate pupils.
Retinal IR injury was induced by increasing the pressure in the anterior chamber via the saline-perfusion system described by Tong et al. (18). Briefly, anesthetized animals with anesthetized corneas and dilated pupils were fixed on a heat-preservation countertop, and their underjaws were raised to prevent death from aspiration of saline. Then, 31-G needles were inserted into the anterior chamber and the intraocular pressure was increased gradually to 110 mmHg for 1 h (Figure 1A). Eyes that underwent needle puncture only but not perfusion were regarded as Sham operation (SO) eyes. During and after the procedure, antibiotic eye ointment was used to keep the eyes moist and uninfected. Only rats without saline-leaking or lens injury were included in our study (a total of two rats were excluded because of lens injury and one rat was discarded because of saline-leaking).
Figure 1. Expression of PPARα in IR-treated retinas (24 h post-modeling) and OGD-treated R28 cells (2 h post-modeling). (A) The diagrammatic picture of IR modeling. (B) The diagrammatic picture of OGD modeling. (C) Representative photomicrographs of immunostained PPARα (red) and nuclei (blue; counterstained by DAPI) in R28 cells and retinas. (D,E) Graph showing the mean fluorescence intensity (MFI) of PPARα in R28 cells and retinas. (F) The expression of PPARα and actin detected by western blotting in R28 cells and retinas. (G,H) Average expression of PPARα semi-quantified by densitometry and normalized to actin expression in R28 cells and retinas. Data are the mean ± SEM; *p < 0.05; **p < 0.01. Scale bar = 50 μm.
Retinal cell line 28 (R28) is an adherent retinal precursor cell line derived from neonatal Sprague-Dawley rat retina, which was immortalized by the 12S E1A gene and commonly used to study the function and neuroprotection of RGCs in vitro (19). In this study, R28 cells were offered by the Department of Anatomy and Neurobiology (Central South University, Changsha, China). Cells were cultured in low glucose DMEM (11885084; Gibco, Carlsbad, USA) with 10% fetal calf serum (0500; ScienCell, San Diego, USA), 1% L-glutamine (G3126; Sigma, St. Louis, USA), 1% non-essential amino acids (M7145; Sigma, St. Louis, USA), and 1% penicillin-streptomycin (60162ES76; Yeasen, Shanghai, China). To establish the Oxygen-Glucose Deprivation (OGD) model, an in vitro model of retinal IR, R28 cells were incubated in a glucose-free DMEM (11966025; Gibco, Carlsbad, USA) with an anoxic environment (95% N2, 5% CO2) for 2 h, then the cells were cultured in normal medium and reoxygenated (21% O2, 5% CO2) for 2 h before being examined (Figure 1B).
Fenofibric acid (FA) is a specific agonist of PPARα. In vivo, FA (S4527; Selleck Chemicals, Houston, TX, USA) was dissolved in dimethyl sulfoxide (DMSO; Solarbio, Beijing, China) at 10 mg/ml. One hour before IR modeling, animals were injected (i.p.) with FA at 1 ml/kg bodyweight. Treatment was administered once a day (excluding the modeling day) until rats were sacrificed 24 or 72 h after modeling. In vitro, 25 μM FA was added to the cell culture medium 1 h before OGD modeling and maintained until the cells were examined.
Anesthetized rats were perfused transcardially with pre-cooled phosphate-buffered saline. Intact eyes were enucleated and fixed in FAS eyeball fixative solution (G1109; Servicebio, Beijing, China) for 24 h at room temperature. After fixation, eyeballs were dehydrated in ethanol and embedded in paraffin. Sections (3 μm) were made around the optic nerve for further immunofluorescence staining and hematoxylin and eosin (HE) staining. Dewaxed and antigen-retrieved paraffin retinal slices were applied to immunofluorescence, as described in our previous study (20). Three antibodies (all from Abcam, Cambridge, UK) were used: anti-PPARα (ab215270; 1:100), anti-glial fibrillary acidic protein (GFAP; ab33922; 1:300), and anti-cyclooxygenase 2 (COX2; ab62331; 1:100). Retina HE staining was performed by the Hematoxylin-Eosin/HE Staining Kit (G1120; Servicebio, Wuhan, China) according to the manufacturer's instructions.
Retinal cell line 28 cells were fixed in 4% paraformaldehyde for 15 min (G1101; Servicebio, Beijing, China) at room temperature before immunofluorescence staining. The specific procedures and antibodies applied were the same as those used for retinal slices. Besides, propidium iodide (PI; P4170; Sigma, St. Louis, USA) staining and Hoechst staining (A3472; APExBIO, Houston, USA) were applied to detect the survival rate of R28 cells according to the manufacturer's instructions.
Freshly isolated retinal tissues were homogenized and lysed in RIPA buffer (P0013; Beyotime, Beijing, China). The protein concentration was quantified by a Bicinchoninic Acid kit (Pierce, Rockford, IL, USA). Western blotting was done as described in our previous study (20) with the following antibodies: anti-PPARα (ab215270; Abcam; 1:1,000), anti-GFAP (ab33922; Abcam; 1:1,000), anti-COX2 (ab62331; Abcam; 1:1,000), and anti-β-actin (GB12001; Servicebio; 1:3,000). The expression levels of PPARα, GFAP and COX2 were quantified by Image-Pro Plus 6.0 (Media Cybernetics, Rockville, MD, USA) and normalized by the densitometry of β-actin.
Total RNA was isolated from fresh retinal tissues with RNAiso buffer (GB3013; Servicebio, Wuhan, China). Peroxisome proliferator-activated receptor α mRNA was reverse transcribed to cDNA with the Servicebio®RT First Strand cDNA Synthesis Kit (G3330; Servicebio), according to the manufacturer's instructions. Peroxisome proliferator-activated receptor α mRNA expression was quantified using 2 × SYBR Green qPCR Master Mix (G3322; Servicebio). Peroxisome proliferator-activated receptor α mRNA levels were normalized to GAPDH mRNA levels. The following specific primers were synthesized by Servicebio: rat PPARα primers, forward 5′-CATCGAGTGTCGAATATGTGG-3′ and reverse 5′-GCAGTACTGGCATTTGTTCC-3′; rat GAPDH primers, forward 5′-GGAAGCTTGTCATCAATGGAAATC-3′ and reverse 5′-TGATGACCCTTTTGGCTCCC-3′.
One week before IR modeling, 4% fluorogold (FG; Fluorochrome, Denver, CO, USA) solution was injected into the bilateral superior colliculi of rats (6 mm posterior to the bregma, 1.8 mm lateral to the cranial midline, and 4 mm deep to the cranial surface) to retrograde label RGCs, as described in our previous study (21).
Flattened retinas were examined by a fluorescence microscope (DM5000 B; Leica, Wetzlar, Germany). Twelve images per retina were taken at 0.85, 2.26, and 3.68 mm (approximately 1/6, 1/2, and 5/6 retinal radius) from the optic disk in superonasal, inferonasal, superotemporal, and inferotemporal quadrants. A double-blind method and Image-Pro Plus 6.0 were used to count the labeled RGCs in each photomicrograph.
The GCC consists of a retinal nerve-fiber layer, GCL and inner plexiform layer, and corresponds to the anatomic distribution of RGCs in the retina (22). To better represent the change in GCC thickness, 12 points of GCC thickness per retinal slice stained by HE were measured by Image-Pro Plus 6.0 according to the following parameters: perpendicular to the surface of the RPE layer as well as ±800, ±1,600, ±2,400, ±3,200, ±4,000, and ±4,800 μm away from the center of the optic nerve (Figure 3F).
Flash Visual-Evoked Potentials (FVEPs) were obtained using a multifocal electroretinography recorder (GT-2008V-VI; Gotec, Chongqing, China) for functional evaluation of retinas 24 or 72 h after modeling. The stimuli intensity was set to 10.0 cd•s/m2, the flash frequency was 1 Hz, and the number of flashes was 64. After light adaptation for 15 min, anesthetized animals were fixed on a special holder with one silver-plate electrode inserted under the skin of the occipital bone (anode), anterior bregma (cathode), and ear (ground electrode), respectively. Then, the FVEP of right and left eyes was recorded in order by a Ganzfeld electrodiagnostic system (Gotec, Chongqing, China). The latency of the first positive wave (P1) and second positive wave (P2) of FVEP was analyzed.
SPSS 22.0 (IBM, Armonk, NY, USA) was utilized for statistical analyses. Data are the mean ± SEM. The Student's t-test and one-way analysis of variance followed by Tukey's post-hoc test were used for comparisons between two groups and more than two groups. Statistical significance was set at p < 0.05.
To verify whether PPARα is involved in the pathological process of retinal IR, we delineated PPARα expression in R28 cells and retinas after OGD/IR modeling by immunofluorescence analyses and western blotting. Oxygen-glucose deprivation-treated R28 cells showed significant cytoplasmic declination of PPARα expression compared with that in the control group (Figures 1C,D,F,G). In retinas, immunostained PPARα was detected in the GCL, INL, ONL, and the RPE, but most PPARα was expressed in the GCL (Figure 1C). Twenty-four hours after IR modeling, retinal immunolabeling level of PPARα was down-regulated, and this change was manifested mainly in the GCL (Figures 1C,E,F,H). Taken together, these data suggested that retinal IR procedure induced down-regulation of PPARα expression both in vitro and in vivo; PPARα was likely to participate in the pathological mechanism of retinal IR.
To clarify the role of PPARα in retinal IR, we used FA to activate PPARα and evaluated the effect of PPARα activation on R28 cells and RGC survival after OGD/IR modeling. As shown in Figures 2A,B, OGD modeling led to nearly half of R28 cells death 2 h after modeling, and FA treatment ameliorated OGD-induced cell death significantly (p = 0.0001, n = 4 per group). In vivo, surviving RGCs labeled by FG had a granular appearance with clear borders and golden color, whereas dead RGCs had a smaller volume and lighter color (white arrows, Figure 2C). Photomicrographs identified that dead RGCs appeared only in IR groups. The number of surviving RGCs was decreased markedly in IR rats in comparison with SO rats 24 and 72 h after modeling (p = 0.0001 for both, n = 6 per group). Moreover, FA treatment was able to increase the number of surviving RGCs (p = 0.0001 at 24 h and p = 0.002 at 72 h, n = 6 per group) (Figures 2D,E; Supplementary Table 1). These results suggested that PPARα activation by FA had a protective effect on IR-induced RGC loss.
Figure 2. Protective effect of PPARα activation by FA on RGC survival in vitro and in vivo. (A) Representative photomicrographs of R28 cells stained by propidium iodide (PI, dead cells) and Hoechst (total cells). (B) The survival rate of R28 cells. (C) RGCs labeled by fluorogold (FG) taken at 1/2 retinal radius distances from the optic disk (white arrows, dead RGCs). (D,E) The average number of FG-labeled RGCs at 24 h/72 h post-IR modeling. Data are the mean ± SEM; **p < 0.01. Scale bar = 50 μm.
To further determine the protective effect of PPARα activation on retinal IR, HE staining was used to measure GCC thickness. At 72 h post-modeling, GCC thickness was decreased significantly in IR rats when compared with SO rats (p = 0.0001, n = 7 in the IR group and n = 5 in the SO group). Fenofibric acid treatment efficaciously attenuated GCC thinning (p = 0.014, n = 7 per group), and all of these changes occurred in the whole retina. However, 24 h after modeling, no obvious difference was found between groups (n = 5 per group) (Figure 3; Supplementary Table 1). In summary, FA alleviated the GCC damage induced by IR at 72 h, suggesting that PPARα activation by FA had a protective effect on IR-induced retinal injury.
Figure 3. PPARα activation by FA mitigates GCC thinning in IR rats. (A) Representative photomicrographs of HE-stained retinal slices taken at 2,400 μm away from the optic nerve at 24 and 72 h post-IR modeling. (B,C) Mean thickness of the GCC at 24 h/72 h post-IR modeling. (D,E) At 24 and 72 h after modeling, the GCC thickness of rats was measured ± 800, ±1,600, ±2,400, ±3,200, ±4,000, and ±4,800 μm away from the optic nerve. (F) The diagrammatic picture of GCC measured in the retina. Data are the mean ± SEM; *p < 0.05. Scale bar = 50 μm.
Moreover, we investigated the protective effect of PPARα activation on visual function. Flash visual-evoked potentials were applied to assess the effect of FA on retinal electrophysiologic activity. The latency of P1 waves and P2 waves was increased by IR at 24 and 72 h after modeling (p = 0.0001 for all, n = 6 per group), indicating that retinal IR severely affected the conduction function of vision. Furthermore, FA treatment decreased the latency of the P1 wave at 24 h (p = 0.021, n = 6 per group) and P2 wave at 24 or 72 h after modeling in IR rats (p = 0.001 for 24 h and p = 0.008 for 72 h, n = 6 per group) (Figure 4; Supplementary Table 1), suggesting that PPARα activation by FA ameliorated IR-induced retinal dysfunction.
Figure 4. Protective effect of PPARα activation by FA on FVEPs in IR rats. (A) Representative images of FVEPs at 24 h post-IR modeling. (B) Representative images of FVEPs at 72 h post-IR modeling. (C) The latency of the first positive wave (P1 wave) and second positive wave (P2 wave) of FVEPs in rats at 24 h post-IR modeling. (D) The latency of the P1 wave and P2 wave of FVEPs in rats at 72 h post-IR modeling. Data are the mean ± SEM; *p < 0.05; **p < 0.01. Scale bar = 10.0 μV and 50 ms.
We have demonstrated that retinal IR down-regulated PPARα expression in vitro and in vivo, and PPARα activation by FA alleviated IR-induced injury and protected visual function. However, the effect of FA on endogenous PPARα expression remains unclear. The immunofluorescence results showed that FA treatment increased PPARα expression in R28 cells and retinas after OGD/IR modeling (Figure 5A; Supplementary Figures 1A,B). Western blotting and Real-time PCR showed that retinal PPARα and mRNA levels were decreased at 24 h post-modeling, while FA treatment effectively reversed these changes (p = 0.035 for Western blotting and p = 0.0001 for PCR, n = 3 per group) (Figures 5B–D). These results suggested that FA as an agonist of PPARα could increase the transcription and translation level of PPARα.
Figure 5. Fenofibric acid increased PPARα expression in OGD-treated R28 cells (2 h post-modeling) and IR-treated retinas (24 h post-modeling). (A) Representative photomicrographs of immunostained PPARα (red) and nuclei (blue; counterstained by DAPI) in R28 cells and retinas. (B) Retinal expression of PPARα and actin detected by western blotting. (C) Average expression of PPARα semi-quantified by densitometry and normalized by actin levels. (D) Average expression of PPARα mRNA detected by Real-time PCR and normalized by GAPDH mRNA levels. Data are the mean ± SEM; *p < 0.05; **p < 0.01. Scale bar = 50 μm.
We wished to determine the underlying mechanism of PPARα activation on RGCs in IR rats. Over-activation of glial cells is one of the crucial pathogenic factors after retinal stress injury, up-regulation of GFAP [a marker of glial cells (23)] is regarded as a sensitive non-specific response of glial cells activation (24). In this study, the expression of GFAP was measured in retinas 24 h after IR modeling. Retinal GFAP was expressed mainly in the GCL, and such expression was up-regulated in the GCL of IR rats. However, FA treatment suppressed an up-regulation of GFAP in the GCL (Figure 6A; Supplementary Figure 1C). Consistent with the results of immunofluorescence analyses, western blotting showed that GFAP expression in retinas was also increased after IR modeling (p = 0.0001, n = 3 per group), FA decreased retinal GFAP level (p = 0.0001, n = 3 per group) and that there was no difference between rats treated by FA and those treated by DMSO in the SO group (Figures 6C,D).
Figure 6. Activation of PPARα by FA repressed GFAP and COX2 expression in the retinas of IR rats. (A,B) Representative photomicrographs of immunostained GFAP/COX2 (red) and nuclei (blue; counterstained by DAPI) in retinal sections at 24 h post-modeling. (C) Retinal expression of GFAP, COX2, and actin detected by western blotting. (D,E) Average expression of GFAP/COX2 semi-quantified by densitometry and normalized by actin levels in retinas. Data are the mean ± SEM; **p < 0.01. Scale bar = 50 μm.
Cyclooxygenase-2 is an important pro-inflammatory molecule (25). To further elucidate the therapeutic mechanism of FA on IR rats, we measured retinal level of COX2 24 h after IR modeling. Immunostained COX2 was detected in the all layers of the retina. Ischemia-reperfusion modeling increased COX2 level in the GCL and inner plexiform layers significantly. In contrast, FA treatment decreased COX2 level (Figure 6B; Supplementary Figure 1D). Western blotting showed that IR-induced retinopathy up-regulated COX2 level in the retina (p = 0.001, n = 3 per group), but this level was down-regulated by FA (p = 0.0001, n = 3 per group. Moreover, there was no distinction between rats treated by FA and those treated by DMSO (Figures 6C,E).
In summary, these results indicated that FA decreased GFAP and COX2 expression in the retina significantly, suggesting that PPARα activation mediated the repression of glial cells activation and suppression of retinal inflammation.
Peroxisome proliferator-activated receptor α is an important lipid-regulating transcription factor. It has been reported that PPARα plays an important part in ophthalmic diseases (14–17). However, the PPARα function in retinal IR is remained unknown. In this study, we first measured PPARα expression in retinal IR condition, and provided evidence that PPARα activation by FA (a PPARα agonist) ameliorated IR-induced RGC injury in vitro and in vivo. Our results suggested that this beneficial effect was through inhibition of activation of retinal glial cells and suppression of retinal inflammation.
Studies have shown that PPARα is expressed in the retina, kidney, intestine, heart and brain (10, 26–28). Peroxisome proliferator-activated receptor α expression in the retina under chronic ischemic and hypoxic conditions has been studied (10, 12), but PPARα expression under acute ischemia and hypoxia has not. Therefore, we first measured PPARα expression in the retinas of IR rats. Consistent with down-regulation of PPARα expression in chronic ischemic retinopathy [e.g., DM-induced retinopathy (10), oxygen-induced proliferative retinopathy (15)] and in other non-retinal organs injured by IR [e.g., heart (29), kidney (30), and intestine (31)], PPARα expression in the IR retina was decreased 24 h after modeling, indicating that PPARα was involved in the retinal pathological process of IR injury. However, unlike the reduction of PPARα expression in the whole retina caused by DM (10), the decrease in IR-induced PPARα expression was manifested mainly in the GCL. One hypothesis is that IR modeling mainly hindered the blood supply of the inner retina by blocking the central retinal artery and central retinal vein (32). In this case, the GCL was more susceptible to ischemia and hypoxia. This hypothesis could be correct because the thickness of the retinal ONL was not affected by IR (Supplementary Figure 2).
To verify the effect of PPARα in IR-induced retinopathy, we used a specific PPARα agonist, FA, to activate PPARα and ascertain if PPARα plays an important part in this pathologic process. First, we used FG to retrograde-label surviving RGCs in the retina. Retinal ganglion cell loss is a crucial feature of acute angle-closure glaucoma, retinal vascular occlusions, and anterior ischemic optic neuropathy. We found that IR modeling led to a significant loss of RGCs 24 and 72 h after modeling, which is consistent with the pathologic process of the diseases mentioned above. Peroxisome proliferator-activated receptor α activation by FA reduced the RGC loss caused by IR efficaciously, indicating that PPARα had a protective role in this process. Second, we measured retinal GCC thickness [a widely used indicator for detection of RGC loss in clinical diagnoses (33, 34)] after FA treatment to further demonstrate the protective effect of PPARα on RGCs. Our results showed that GCC was thinner than the normal group at 72 h post-modeling, and FA treatment alleviated this structural change in the IR retina. However, a change in GCC thickness 24 h after modeling was not found, indicating that the change in GCC thickness occurred later than RGC death. Third, we detected RGC function. Use of FVEPs is a sensitive method that reflects the function of visual pathways (35). Studies have demonstrated that RGC damage would directly affect the visual conduction function, resulting in extension of the latency of each peak of FVEPs (36, 37). In our study, IR-induced RGC injury also increased the latency of the P1 wave and P2 wave of FVEPs, but FA treatment reduced the prolongation of latency. In summary, PPARα activation by FA alleviated IR-induced damage to the structure and function of RGCs, actions that are consistent with its protective effects in diabetic retinopathy or oxygen-induced proliferative retinopathy (14–17). In addition, our results are also consistent with the studies reported by Bulhak et al. (38) and Ravingerova et al. (39), they both demonstrate that PPARα activation protects myocardium from IR injury. All these results suggest that PPARα activation had a protective role in the retina, and PPARα itself could be a potential target for treatment of IR-induced retinopathy.
Fenofibrate is a synthetic ligand of PPARα and has been used as a hypolipidemic drug for >30 years (40). Fenofibrate is safe and inexpensive, and use of fenofibrate for treating retinal diseases was inspired by two large clinical studies (FIELD and ACCORD) in which researchers reported its robust therapeutic effects on retinopathy in patients with type-2 DM (41, 42). Fenofibric acid is the active metabolite of fenofibrate (43) and retains the function and advantage of fenofibrate while avoiding some off-target effects caused by direct application of fenofibrate [e.g., inhibition of cytochrome-P450 expression or suppression of voltage-dependent K+ channels (44, 45)]. All of these advantages might be conducive to convert FA to a drug for clinical treatment. As for the potential time window for FA treatment of ophthalmic clinical disease (such as acute angle-closure glaucoma, retinal vascular occlusions, and anterior ischemic optic neuropathy), we cannot predict the occurrence of these diseases and administer FA in advance as in this study, so we consider that FA should be administered as soon as these diseases are diagnosed.
We investigated the underlying mechanism of the neuroprotective effect of PPARα activation. Some in vitro studies have shown that fenofibrate and FA have a stimulatory effect on elevation of PPARa levels in osteogenic precursor cells (46) and palmitate treated retinal precursor cells (47). Our results demonstrated that FA also increased the expression of PPARα in a retinal IR model, indicating that FA exerts its neuroprotective effect partially relies on up-regulation of PPARα. Glial cells are crucial for maintaining the blood–retinal barrier and RGC survival (24, 48, 49). It has been reported that glial cells may lose their physiologic functions and be activated in disease or injury, and that over-activation of glial cells is related to retinal neurodegeneration (24). Moran et al. demonstrated that PPARα activation can attenuate over-activation of glial cells in an oxygen-induced retinopathy model (15). Hence, we conjectured that PPARα exerted its protective role in an IR model through this mechanism. We found that expression of GFAP (a marker of glial cells) was increased markedly in the GCL after IR modeling, and that FA treatment reduced GFAP expression significantly. Besides, we measured expression of COX2 (an important proinflammatory mediator produced by activated glial cells) because increased COX2 expression can aggravate local inflammation and promote RGC death (50). After IR modeling, COX2 expression increased significantly, and FA treatment attenuated this increase markedly. These data are in accordance with the work of Zhang et al., who also found that activated glial cells could produce COX2 rapidly in injured optic nerves (51). All these results suggested that the therapeutic effect of PPARα activation was due (at least in part) to inhibition of activation of glial cells and reduction of inflammation in the retina. Importantly, the changes in expression of GFAP and COX2 manifested mainly in the inner layer of the retina (especially in the GCL), which provides further evidence that activation of glial cells and inflammation had direct roles in RGC injury.
In this study, we demonstrated, for the first time, that PPARα is involved in the pathologic process of retinal IR. Peroxisome proliferator-activated receptor α activation by FA ameliorates IR-induced RGC injury and protects visual function, inhibits activation of glial cells and suppresses retinal inflammation. Taken together, these findings suggest that PPARα could be a new target for the treatment of retinal IR-related ophthalmic diseases.
The original contributions presented in the study are included in the article/Supplementary Materials, further inquiries can be directed to the corresponding author/s.
The animal study was reviewed and approved by Animal Research Committee of the Xiangya School of Medicine.
FY wrote the first draft of the paper. XZ, XX, and LD edited the paper. FY, JJ, and LD designed research. FY, XZ, and XY performed research. FY and XR analyzed data. All authors contributed to the article and approved the submitted version.
This work was supported by the National Natural Science Foundation of China (81500720 and 82070966 to LD) and the Science and Technology Innovation Program of Hunan Province (2021RC3026 to LD).
The authors declare that the research was conducted in the absence of any commercial or financial relationships that could be construed as a potential conflict of interest.
All claims expressed in this article are solely those of the authors and do not necessarily represent those of their affiliated organizations, or those of the publisher, the editors and the reviewers. Any product that may be evaluated in this article, or claim that may be made by its manufacturer, is not guaranteed or endorsed by the publisher.
We thank Prof. Ma Jianxing (Health Sciences Center, Oklahoma University, Oklahoma, USA) for helpful comments on the manuscript, Yangzhou Zhang, Jingning Long, Rong Rong, Mengling You, and Zhaolin Gao for technical assistance.
The Supplementary Material for this article can be found online at: https://www.frontiersin.org/articles/10.3389/fmed.2021.788663/full#supplementary-material
1. Sanes JR, Masland RH. The types of retinal ganglion cells: current status and implications for neuronal classification. Annu Rev Neurosci. (2015) 38:221–46. doi: 10.1146/annurev-neuro-071714-034120
2. Flores-Sanchez BC, Tatham AJ. Acute angle closure glaucoma. Br J Hosp Med (Lond). (2019) 80:C174–9. doi: 10.12968/hmed.2019.80.12.C174
3. Scott IU, Campochiaro PA, Newman NJ, Biousse V. Retinal vascular occlusions. Lancet. (2020) 396:1927–40. doi: 10.1016/S0140-6736(20)31559-2
4. Akbari M, Abdi P, Fard MA, Afzali M, Ameri A, Yazdani-Abyaneh A, et al. Retinal Ganglion cell loss precedes retinal nerve fiber thinning in nonarteritic anterior ischemic optic neuropathy. J Neuroophthalmol. (2016) 36:141–6. doi: 10.1097/WNO.0000000000000345
5. Boia R, Ruzafa N, Aires ID, Pereiro X, Ambrosio AF, Vecino E, et al. Neuroprotective Strategies for retinal Ganglion cell degeneration: current status and challenges ahead. Int J Mol Sci. (2020) 21:2262. doi: 10.3390/ijms21072262
6. Bordet R, Ouk T, Petrault O, Gele P, Gautier S, Laprais M, et al. PPAR: a new pharmacological target for neuroprotection in stroke and neurodegenerative diseases. Biochem Soc Trans. (2006) 34(Pt 6):1341–6. doi: 10.1042/BST0341341
7. Chen XR, Besson VC, Palmier B, Garcia Y, Plotkine M, Marchand-Leroux C. Neurological recovery-promoting, anti-inflammatory, and anti-oxidative effects afforded by fenofibrate, a PPAR alpha agonist, in traumatic brain injury. J Neurotrauma. (2007) 24:1119–31. doi: 10.1089/neu.2006.0216
8. Araki M, Nakagawa Y, Oishi A, Han SI, Wang Y, Kumagai K, et al. The peroxisome proliferator-activated receptor alpha (PPARalpha) agonist pemafibrate protects against diet-induced obesity in mice. Int J Mol Sci. (2018) 19:2148. doi: 10.3390/ijms19072148
9. Wojtowicz S, Strosznajder AK, Jezyna M, Strosznajder JB. The novel role of PPAR alpha in the brain: promising target in therapy of Alzheimer's disease and other neurodegenerative disorders. Neurochem Res. (2020) 45:972–88. doi: 10.1007/s11064-020-02993-5
10. Hu Y, Chen Y, Ding L, He X, Takahashi Y, Gao Y, et al. Pathogenic role of diabetes-induced PPAR-alpha down-regulation in microvascular dysfunction. Proc Natl Acad Sci U S A. (2013) 110:15401–6. doi: 10.1073/pnas.1307211110
11. Pearsall EA, Cheng R, Zhou K, Takahashi Y, Matlock HG, Vadvalkar SS, et al. PPARalpha is essential for retinal lipid metabolism and neuronal survival. BMC Biol. (2017) 15:113. doi: 10.1186/s12915-017-0451-x
12. Chen Q, Qiu F, Zhou K, Matlock HG, Takahashi Y, Rajala RVS, et al. Pathogenic role of microRNA-21 in diabetic retinopathy through downregulation of PPARalpha. Diabetes. (2017) 66:1671–82. doi: 10.2337/db16-1246
13. Wang Y, Lin W, Ju J. MicroRNA-409-5p promotes retinal neovascularization in diabetic retinopathy. Cell Cycle. (2020) 19:1314–25. doi: 10.1080/15384101.2020.1749484
14. Ding L, Cheng R, Hu Y, Takahashi Y, Jenkins AJ, Keech AC, et al. Peroxisome proliferator-activated receptor alpha protects capillary pericytes in the retina. Am J Pathol. (2014) 184:2709–20. doi: 10.1016/j.ajpath.2014.06.021
15. Moran E, Ding L, Wang Z, Cheng R, Chen Q, Moore R, et al. Protective and antioxidant effects of PPARalpha in the ischemic retina. Invest Ophthalmol Vis Sci. (2014) 55:4568–76. doi: 10.1167/iovs.13-13127
16. Feng X, Gao X, Wang S, Huang M, Sun Z, Dong H, et al. PPAR-alpha Agonist fenofibrate prevented diabetic nephropathy by inhibiting M1 macrophages via improving endothelial cell function in db/db mice. Front Med (Lausanne). (2021) 8:652558. doi: 10.3389/fmed.2021.652558
17. Qiu F, Matlock G, Chen Q, Zhou K, Du Y, Wang X, et al. Therapeutic effects of PPARalpha agonist on ocular neovascularization in models recapitulating neovascular age-related macular degeneration. Invest Ophthalmol Vis Sci. (2017) 58:5065–75. doi: 10.1167/iovs.17-22091
18. Tong JB, Chen D, Zeng LP, Mo XY, Wang H, Huang J, et al. Differential changes of local blood supply in rat retinae are involved in the selective loss of retinal ganglion cells following the acute high intraocular pressure. Curr Eye Res. (2010) 35:425–34. doi: 10.3109/02713680903514675
19. Rong R, Xia X, Peng H, Li H, You M, Liang Z, et al. Cdk5-mediated Drp1 phosphorylation drives mitochondrial defects and neuronal apoptosis in radiation-induced optic neuropathy. Cell Death Dis. (2020) 11:720. doi: 10.1038/s41419-020-02922-y
20. Xiong Y, Ji H, You Z, Yao F, Zhou R, Song W, et al. Otx2 enhances transdifferentiation of Muller cells-derived retinal stem cells into photoreceptor-like cells. J Cell Mol Med. (2019) 23:943–53. doi: 10.1111/jcmm.13995
21. Yao F, Zhang E, Gao Z, Ji H, Marmouri M, Xia X. Did you choose appropriate tracer for retrograde tracing of retinal ganglion cells? The differences between cholera toxin subunit B and fluorogold. PLoS ONE. (2018) 13:e0205133. doi: 10.1371/journal.pone.0205133
22. Bloch E, Yonova-Doing E, Jones-Odeh E, Williams KM, Kozareva D, Hammond CJ. Genetic and environmental factors associated with the Ganglion cell complex in a healthy aging British cohort. JAMA Ophthalmol. (2017) 135:31–8. doi: 10.1001/jamaophthalmol.2016.4486
23. Yang Z, Wang KK. Glial fibrillary acidic protein: from intermediate filament assembly and gliosis to neurobiomarker. Trends Neurosci. (2015) 38:364–74. doi: 10.1016/j.tins.2015.04.003
24. Bringmann A, Pannicke T, Biedermann B, Francke M, Iandiev I, Grosche J, et al. Role of retinal glial cells in neurotransmitter uptake and metabolism. Neurochem Int. (2009) 54:143–60. doi: 10.1016/j.neuint.2008.10.014
25. Chan PC, Hsiao FC, Chang HM, Wabitsch M, Hsieh PS. Importance of adipocyte cyclooxygenase-2 and prostaglandin E2-prostaglandin E receptor 3 signaling in the development of obesity-induced adipose tissue inflammation and insulin resistance. FASEB J. (2016) 30:2282–97. doi: 10.1096/fj.201500127
26. Chinetti G, Fruchart JC, Staels B. Peroxisome proliferator-activated receptors (PPARs): nuclear receptors at the crossroads between lipid metabolism and inflammation. Inflamm Res. (2000) 49:497–505. doi: 10.1007/s000110050622
27. Wayman NS, Hattori Y, McDonald MC, Mota-Filipe H, Cuzzocrea S, Pisano B, et al. Ligands of the peroxisome proliferator-activated receptors (PPAR-gamma and PPAR-alpha) reduce myocardial infarct size. FASEB J. (2002) 16:1027–40. doi: 10.1096/fj.01-0793com
28. Chandra S, Jana M, Pahan K. Aspirin induces lysosomal biogenesis and attenuates amyloid plaque pathology in a mouse model of Alzheimer's disease via PPARAspirin induces lysosomal biogenesis and attenuates amyloid plaque pathology in a mouse model of Alzheimer's disease via PPARα. J Neurosci. (2018) 38:6682–99. doi: 10.1523/JNEUROSCI.0054-18.2018
29. Yuan J, Mo H, Luo J, Zhao S, Liang S, Jiang Y, et al. PPARα activation alleviates damage to the cytoskeleton during acute myocardial ischemia/reperfusion in rats. Mol Med Rep. (2018) 17:7218–26. doi: 10.3892/mmr.2018.8771
30. Sivarajah A, Chatterjee PK, Hattori Y, Brown PA, Stewart KN, Todorovic Z, et al. Agonists of peroxisome-proliferator activated receptor-alpha (clofibrate and WY14643) reduce renal ischemia/reperfusion injury in the rat. Med Sci Monit. (2002) 8:BR532–9. doi: 10.1017/S1355770X11000337
31. Li LX, Yin LH, Gao M, Xu LN Qi Y, Peng JY. MiR-23a-5p exacerbates intestinal ischemia-reperfusion injury by promoting oxidative stress via targeting PPAR alpha. Biochem Pharmacol. (2020) 180:114194. doi: 10.1016/j.bcp.2020.114194
32. Osborne NN, Casson RJ, Wood JP, Chidlow G, Graham M, Melena J. Retinal ischemia: mechanisms of damage and potential therapeutic strategies. Prog Retin Eye Res. (2004) 23:91–147. doi: 10.1016/j.preteyeres.2003.12.001
33. Dascalescu D, Corbu C, Coviltir V, Schmitzer S, Constantin M, Burcel M, et al. The ganglion cell complex as an useful tool in glaucoma assessment. Rom J Ophthalmol. (2018) 62:300–3. doi: 10.22336/rjo.2018.46
34. Asanad S, Tian JJ, Frousiakis S, Jiang JP, Kogachi K, Felix CM, et al. Optical coherence tomography of the retinal Ganglion cell complex in leber's hereditary optic neuropathy and dominant optic atrophy. Curr Eye Res. (2019) 44:638–44. doi: 10.1080/02713683.2019.1567792
35. Young B, Eggenberger E, Kaufman D. Current electrophysiology in ophthalmology: a review. Curr Opin Ophthalmol. (2012) 23:497–505. doi: 10.1097/ICU.0b013e328359045e
36. Huo Y, Yin XL Ji SX, Zou H, Lang M, Zheng Z, et al. Amino-Nogo inhibits optic nerve regeneration and functional recovery via the integrin alphav signaling pathway in rats. Cell Physiol Biochem. (2015) 35:616–26. doi: 10.1159/000369723
37. Liu L, Sun Q, Wang R, Chen Z, Wu J, Xia F, et al. Methane attenuates retinal ischemia/reperfusion injury via anti-oxidative and anti-apoptotic pathways. Brain Res. (2016) 1646:327–33. doi: 10.1016/j.brainres.2016.05.037
38. Bulhak AA, Jung C, Ostenson CG, Lundberg JO, Sjoquist PO, Pernow J. PPAR-alpha activation protects the type 2 diabetic myocardium against ischemia-reperfusion injury: involvement of the PI3-Kinase/Akt and NO pathway. Am J Physiol Heart Circ Physiol. (2009) 296:H719–27. doi: 10.1152/ajpheart.00394.2008
39. Ravingerova T, Carnicka S, Nemcekova M, Ledvenyiova V, Adameova A, Kelly T, et al. PPAR-alpha activation as a preconditioning-like intervention in rats in vivo confers myocardial protection against acute ischaemia-reperfusion injury: involvement of PI3K-Akt. Can J Physiol Pharmacol. (2012) 90:1135–44. doi: 10.1139/y2012-052
40. Canzler H, Bojanovski D. Lowering effect of fenofibrate (procetofene) on lipoproteins in different types of hyperlipoproteinemias. Artery. (1980) 8:171–8.
41. Keech AC, Mitchell P, Summanen PA, O'Day J, Davis TM, Moffitt MS, et al. Effect of fenofibrate on the need for laser treatment for diabetic retinopathy (FIELD study): a randomised controlled trial. Lancet. (2007) 370:1687–97. doi: 10.1016/S0140-6736(07)61607-9
42. Group AS, Group AES, Chew EY, Ambrosius WT, Davis MD, Danis RP, et al. Effects of medical therapies on retinopathy progression in type 2 diabetes. N Engl J Med. (2010) 363:233–44. doi: 10.1056/NEJMoa1001288
43. Vlase L, Popa A, Muntean D, Leucuta SE. Pharmacokinetics and comparative bioavailability of two fenofibrate capsule formulations in healthy volunteers. Arzneimittelforschung. (2010) 60:560–3. doi: 10.1055/s-0031-1296325
44. Li H, Shin SE, Seo MS, An JR, Jung WK, Ha KS, et al. The PPARalpha activator fenofibrate inhibits voltage-dependent K(+) channels in rabbit coronary arterial smooth muscle cells. Eur J Pharmacol. (2017) 812:155–62. doi: 10.1016/j.ejphar.2017.07.027
45. Gong Y, Shao Z, Fu Z, Edin ML, Sun Y, Liegl RG, et al. Fenofibrate inhibits cytochrome P450 epoxygenase 2C activity to suppress pathological ocular angiogenesis. EBioMedicine. (2016) 13:201–11. doi: 10.1016/j.ebiom.2016.09.025
46. Kim YH, Jang WG, Oh SH, Kim JW, Lee MN, Song JH, et al. Fenofibrate induces PPARalpha and BMP2 expression to stimulate osteoblast differentiation. Biochem Biophys Res Commun. (2019) 520:459–65. doi: 10.1016/j.bbrc.2019.10.048
47. Deng G, Moran EP, Cheng R, Matlock G, Zhou K, Moran D, et al. Therapeutic Effects of a novel agonist of peroxisome proliferator-activated receptor alpha for the treatment of diabetic retinopathy. Invest Ophthalmol Vis Sci. (2017) 58:5030–42. doi: 10.1167/iovs.16-21402
48. Kaur C, Foulds WS, Ling EA. Blood-retinal barrier in hypoxic ischaemic conditions: basic concepts, clinical features and management. Prog Retin Eye Res. (2008) 27:622–47. doi: 10.1016/j.preteyeres.2008.09.003
49. Biesecker KR, Srienc AI, Shimoda AM, Agarwal A, Bergles DE, Kofuji P, et al. Glial cell calcium signaling mediates capillary regulation of blood flow in the retina. J Neurosci. (2016) 36:9435–45. doi: 10.1523/JNEUROSCI.1782-16.2016
50. Luo H, Zhuang J, Hu P, Ye W, Chen S, Pang Y, et al. Resveratrol delays retinal ganglion cell loss and attenuates gliosis-related inflammation from ischemia-reperfusion injury. Invest Ophthalmol Vis Sci. (2018) 59:3879–88. doi: 10.1167/iovs.18-23806
Keywords: peroxisome proliferator-activated receptor α, fenofibric acid, ischemia-reperfusion, neuroprotection, retinal ganglion cell, retinal diseases
Citation: Yao F, Zhang X, Yao X, Ren X, Xia X, Jiang J and Ding L (2021) Peroxisome Proliferator-Activated Receptor α Activation Protects Retinal Ganglion Cells in Ischemia-Reperfusion Retinas. Front. Med. 8:788663. doi: 10.3389/fmed.2021.788663
Received: 03 October 2021; Accepted: 24 November 2021;
Published: 23 December 2021.
Edited by:
Hetian Lei, Shenzhen Eye Hospital, ChinaReviewed by:
Xiaoyan Dai, Guangzhou Medical University, ChinaCopyright © 2021 Yao, Zhang, Yao, Ren, Xia, Jiang and Ding. This is an open-access article distributed under the terms of the Creative Commons Attribution License (CC BY). The use, distribution or reproduction in other forums is permitted, provided the original author(s) and the copyright owner(s) are credited and that the original publication in this journal is cited, in accordance with accepted academic practice. No use, distribution or reproduction is permitted which does not comply with these terms.
*Correspondence: Jian Jiang, amlhbmdqaWFueHlAMTI2LmNvbQ==; Lexi Ding, bGV4aWRpbmdAY3N1LmVkdS5jbg==
†These authors have contributed equally to this work and share first authorship
Disclaimer: All claims expressed in this article are solely those of the authors and do not necessarily represent those of their affiliated organizations, or those of the publisher, the editors and the reviewers. Any product that may be evaluated in this article or claim that may be made by its manufacturer is not guaranteed or endorsed by the publisher.
Research integrity at Frontiers
Learn more about the work of our research integrity team to safeguard the quality of each article we publish.