- 1Shunyi Hospital, Beijing Hospital of Traditional Chinese Medicine, Beijing, China
- 2Institute of Plant Resources, Yunnan University, Kunming, China
- 3Department of Emergency, China-Japan Friendship Hospital, Beijing, China
Tripterygium wilfordii Hook. f. (TWHF) is a traditional Chinese herbal medicine and widely used to treat diabetic kidney disease in China. Emerging evidences have revealed its ability to attenuate diabetic nephropathy (DN). Tripterygium wilfordii polyglycosides (TWPs), triptolide (TP), and celastrol are predominantly active compounds isolated from TWHF. The effects and molecular mechanisms of TWHF and its active compounds have been investigated in recent years. Currently, it is becoming clearer that the effects of TWHF and its active compounds involve in anti-inflammation, anti-oxidative stress, anti-fibrosis, regulating autophagy, apoptosis, and protecting podocytes effect. This review presents an overview of the current findings related to the effects and mechanisms of TWHF and its active compounds in therapies of DN, thus providing a systematic understanding of the mechanisms and therapeutic targets by which TWHF and its active compounds affect cells and tissues in vitro and in vivo.
Introduction
Diabetic nephropathy (DN) is defined as decreased renal function with persistent clinically detectable proteinuria (1). As a serious microvascular complication of types 1 or 2 diabetes mellitus (DM), DN occurs in ~25–40% of patients with DM, and has become the leading cause of end-stage renal disease (ESRD) in China (2, 3). Approximately 463 million people suffers from DM worldwide in 2019, and are expected to raise up to 700 million untill 2045 (4).
Proteinuria, an independent risk factor of disease progression, is the most important clinical characteristic of DN. The presence of microalbuminuria can increase all-cause mortality in patients with diabetes mellitus (DM) (5). Without early intervention, ~50% of DM patients with microalbuminuria will progress to macroalbuminuria (6, 7). Although several recent studies have confirmed that angiotensin-converting enzyme inhibitors (ACEIs)/angiotensin receptor blockers (ARBs) can reduce DN proteinuria and delay disease progression (8, 9), these have been shown to be ineffective in DN patients with normal blood pressure (10).
Various traditional Chinese herbal medicine (CHM) has been shown to be effective in the treatment of proteinuria (11, 12). Tripterygium wilfordii Hook. f. (TWHF), also known as Lei Gong Teng, is a traditional CHM which is widely used in the treatment of the inflammation and autoimmune disorders (13–15). Based on its diverse pharmacological activities, TWHF has been used to treat different diseases, such as cancer, rheumatoid arthritis, and Crohn's disease (16–18). Recent experimental and clinical studies have demonstrated that TWHF could significantly reduce proteinuria, protect renal function, and attenuate kidney injury (19–21).
Several randomized controlled clinical trials have found that TWHF possibly imparts nephroprotective effects by decreasing proteinuria, serum creatinine (Scr) levels, and blood urea nitrogen (BUN) levels (22–24). A network pharmacology research showed that TWHF may play a role in treating DN through AGE-RAGE signaling pathway, TNF signaling pathway, IL-17 signaling pathway, insulin resistance, and calcium signaling pathway (25). However, the underlying mechanisms by which TWHF and its active compounds attenuate proteinuria in DN remain unclear. This review discusses the molecular mechanisms of TWHF therapies in proteinuria in DN.
Main Active Compounds of TWHF
TWHF belongs to genus Tripterygium of family celastraceous, and its main bioactive ingredients include terpenoids, tripterygium wilfordii polyglycosides (TWPs), lignans, glycosides, and alkaloids. The terpenoids of TWHF are constituted by sesquiterpenes, diterpenes (triptonide, tripdiolide, and triptolide), triterpenes (wilforlide A, pristimerin, and celastrol) (26, 27).
TWPs, triptolide (TP) and celastrol, predominantly active natural products isolated from TWHF, are mainly used to treat DN (Figure 1). As the fat-soluble mixture extracted from the root of TWHF, TWPs are the first CHM studied and used in anti-inflammatory and immune regulation (28). In 1972, Kupchan et al. first isolated and characterized TP from TWHF (26). Celastrol was first isolated from TWHF for the activator of the mammalian heat shock transcription factor 1 (29). The pharmacological activities and mechanisms of TWHF and its active compounds have been extensively investigated in many kidney disease models (Table 1, Figures 2, 3).
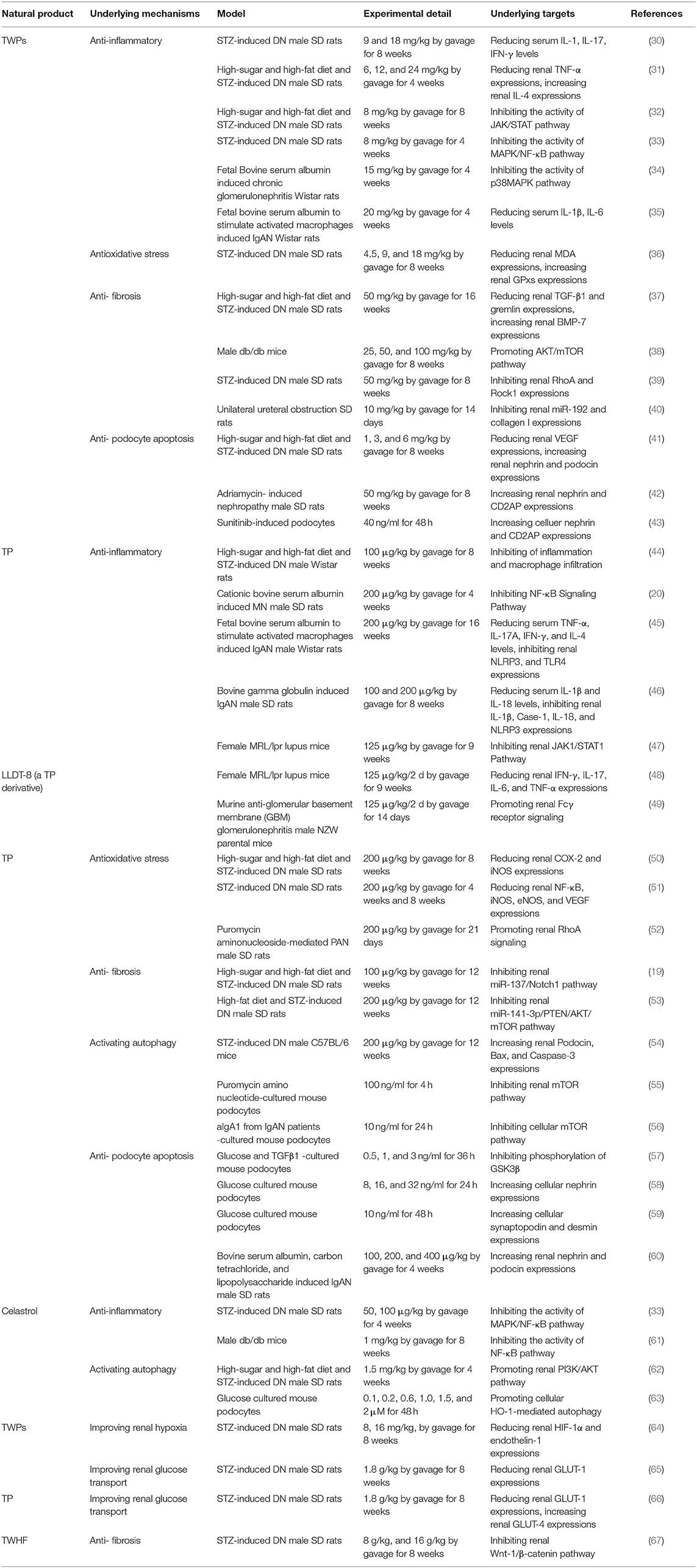
Table 1. Pharmacological activities of Tripterygium wilfordii Hook. f. and active compounds against proteinuria and kidney injury in DN.
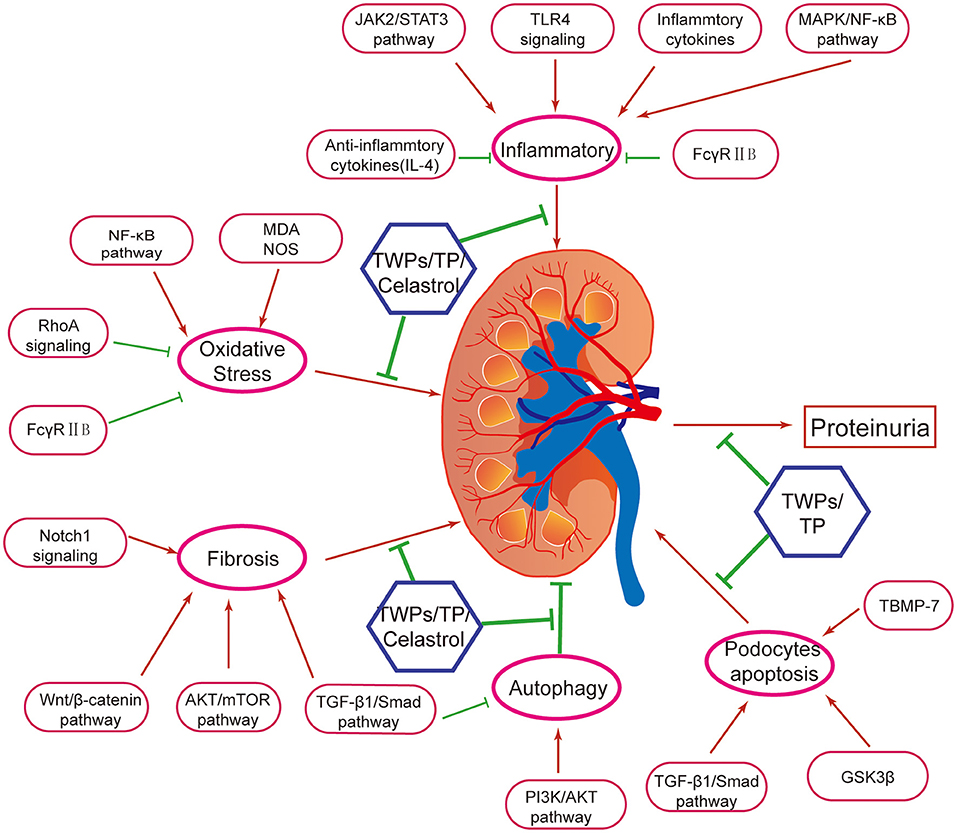
Figure 2. Mechanisms of Tripterygium wilfordii Hook. f. and active compounds against proteinuria and kidney injury in DN. TWPs, TP, and Celastrol are the effective medicine against proteinuria and kidney injury in DN. Mechanisms of TWHF, TWPs, TP, and Celastrol are including anti-inflammation, antioxidation, anti-fibrosis, activating autophagy, and anti- podocyte apoptosis, via several mechanisms.
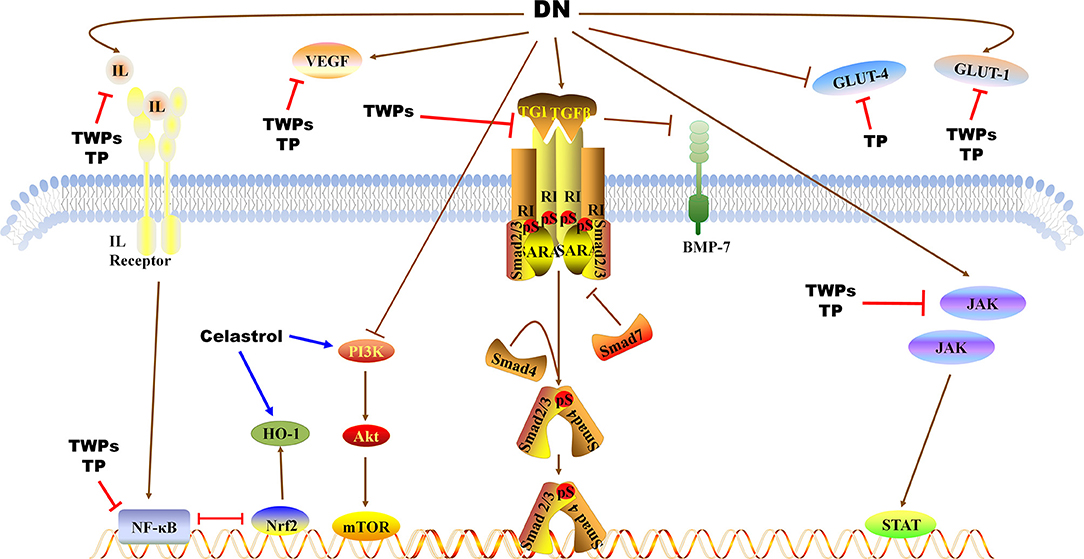
Figure 3. Pathways of TWPs, TP, and Celastrol against proteinuria and kidney injury in DN. TWPs and TP attenuate proteinuria in DN by regulating JAK/STAT pathway, TGF-β1/Smad pathway and NF-κB pathway, and regulating the expressions of IL, VEGF, BMP-7, GLU-1, and GLU-4. Celastrol attenuates proteinuria in DN by regulating PI3K/AKT/mTOR pathway and regulating the expressions of HO-1.
Effects, Mechanisms, and Therapeutic Targets of TWPS Against Proteinuria and Kidney Injury in DN
Anti-inflammatory Effects
Chronic systemic inflammation is associated with kidney injury, and animal and human studies have established that inflammation is a cornerstone in the development and progression of DN (68, 69). Inflammation can alter or interfere with the regulation and perfusion distribution can induce kidney injury, thereby enhancing the DN progression. Overproduction of Advanced glycation end products (AGEs)or damage from degradation may activate inflammation, which, in turn, promotes DN (70). Thus, the regulation of inflammation is key to the development of treatment schemes for kidney disease.
TWPs exhibit anti-inflammation activity in DN rats. TWPs improve renal inflammatory injury in DN rats by reducing the levels of inflammatory cytokines, such as IL-1, IL-17 and interferon- γ (IFN-γ) (30). TWPs downregulate TNF-α, whereas it upregulated IL-4 (anti-inflammatory T-helper cell type 2 cytokine) in renal tissues (31). The JAK2/STAT3 signaling pathway regulates a broad range of biological effects such as cell proliferation, differentiation, inflammation, and apoptosis (71). Inhibiting JAK2/STAT3 activation, which contributes to the pathogenesis of DN, has been shown to be a novel therapeutic scheme for the treatment of this disease (72). In DN rats, TWPs reduce the levels of BUN, Scr and improve kidney function, and also effectively blank the inflammatory response by inhibiting the activity of JAK/STAT pathway (32). Treatment with TWPs also inhibit inflammation via regulating the signal pathway of MAPK/NF-κB in renal tissues (33).
In bovine serum albumin induced chronic glomerulonephritis rat model, TWPs inhibit the inflammatory factor (TNF-α, IL-1β) expressions, and improve the renal pathological damage via regulating MAPK signaling pathway (34). In immunoglobulin A nephropathy (IgAN) rats, TWPs decrease the levels of serum IL-1β, IL-6, and reduce the pathological damage of renal tissue (35) (Table 1, Figures 2, 3).
Antioxidative Stress Effects of TWPs
Oxidative stress is associated with inflammation in DN progression. The presence and severity of systemic inflammation contribute to kidney injury-related oxidative stress (73). Oxidative stress caused by the overaccumulation of reactive oxygen species (ROS) induces protein and nucleic acid damage, thereby leading to impaired cellular damage and tissue pathology (74). The mitochondria are the major sources of ROS as well as the main targets of ROS (75). The damaged mitochondria with impaired respiration block the transfer of electrons along the respiratory chain, which then react with O2 in upstream respiratory chain components to form superoxide free radicals and ROS (76). In response to the excessive production of ROS, mammalian cells have evolved various peroxidases that catalyze the conversion of intracellular hydrogen peroxide to water. These include catalase, peroxiredoxins, and glutathione peroxidases (GPxs) (77). There is increasing evidence that oxidative stress contributes to DN progression (78, 79). TWPs up-regulate the levels of catalase in serum and GPxs in kidneys, and down-regulated the levels of malondialdehyde (MDA) in kidneys in the DN (36) (Table 1, Figures 2, 3).
Anti-fibrosis Effects
Renal fibrosis is a highly complex process involving a variety of cell types including resident renal cells as well as infiltrating cells, such as macrophages, fibrocytes, and lymphocytes. Intracellular ROS generation in the context of diabetes initiates multiple inflammatory and profibrotic responses (80). Renal fibrosis in DN is caused by the accumulation of extracellular matrix (ECM) proteins, including predominantly various collagens, fibronectin, and laminin (81). Thickening of the glomerular basement membrane (GBM) is an early histopathological finding in DN (82). Altered GBM remnants contribute to the expansion of the mesangial matrix, but hyperglycemia also stimulates mesangial cells to proliferate and produce matrix by activating transforming growth factor-β (TGF-β) and vascular endothelial growth factor (VEGF), which directly induce the transcriptional activation of matrix collagens (83). It is currently believed that renal fibrosis develops in response to ECM accumulation due to epithelial-mesenchymal transition (EMT), TGF-β signaling, oxidative stress and proteinuria (84, 85).
TGF-β1/Smad signaling pathway plays a critical role in prolonged glomerulosclerosis, which is an important determinant during the progression in DN (86). Bone morphogenetic protein-7 (BMP-7) is a critical developmental and differentiation factor in the kidney, which can inhibit TGF-β signaling to ameliorate renal inflammation, apoptosis, and fibrosis after kidney injury (87, 88). In DN rats, TWPs ameliorate renal fibrosis by down-regulating the expression of TGF-β1 and gremlin (a BMP antagonist), and up-regulating the expression of BMP-7 (37). In db/db mice, TWPs reduce the serum levels of TC, TG, and LDL, glycated serum protein, BUN, Scr, and improve the renal injury by regulating AKT/mTOR pathway (38). And TWPs inhibit the expressions of RhoA and Rock1 to improve renal fibrosis in STZ-induced rats (39).
MicroRNAs (miRNAs) are a class of small non-coding RNAs that regulate gene expression by either downregulating mRNA levels or directly repressing translation of genes. Many miRNAs are corrected with renal injury in DN (89, 90). In unilateral ureteral obstruction rats, TWPs could attenuate renal fibrosis by inhibiting the expression of miR-192 and collagen I (40) (Table 1, Figures 2, 3).
Anti-podocyte Apoptosis Effects
Podocyte injury is a pathological feature in DN. Podocytes are highly specialized, terminally differentiated epithelial cells in the glomerular filtration barrier with interdigitating foot processes (FPs), and play a major role in preventing protein leakage into the Bowman space (91). Structural podocyte injury is central in the pathogenesis of most inherited and acquired glomerular diseases, which are all associated with decreased expression of slit diaphragm (SD) proteins, such as podocin, nephrin, synaptopodin, and CD2-associated protein (CD2AP) (92). These proteins are considered as critical components of epithelial SD and FPs and help maintain the integrity of podocytes in avoiding proteinuria (93). In addition, desmin is a component of the cytoskeleton and considered as a sensitive marker of injury in podocytes (94). DM induces podocytopathy, which is characterized by cellular hypertrophy, foot process effacement, and podocyte loss (6). Li et al. (41) showed using STZ-induced DN rats that TWPs could upregulate the expression of nephrin and podocin and suppress apoptosis in podocytes.
TWPs have also been shown to significantly reduce proteinuria and repair podocyte damage in rats with adriamycin-induced nephropathy, as well as facilitate mixing together of foot processes by upregulating nephrin and CD2AP (42). In addition, TWPs upregulates nephrin and CD2AP in sunitinib-induced podocytes (43) (Table 1, Figures 2, 3).
Effects, Mechanisms, and Therapeutic Targets of TP Against Proteinuria and Kidney Injury in DN
Anti-inflammatory Effects
Due to similar structures as hormones, TP can bind to nuclear receptors (95). This unique feature is the reason that triptolide is active to inflammation. Ma et al. (44) have shown that TP markedly attenuated proteinuria and renal injury in DN rats, which may have been correlated with the inhibition of macrophage infiltration and inflammation in the kidneys.
Chronic inflammation is also a common characteristic of membranous nephropathy (MN) and IgAN. Zhou et al. (20) concluded that TP significantly reduces the production of inflammatory cytokines (e.g., IL-1β, TNF-α, and monocyte chemotactic protein 1), and inhibits the NF-κB signaling pathway in MN rats. He et al. (45) declared that TP prevents IgAN progression via by ameliorating of inflammasome-mediated proinflammatory cytokine production by down-regulating Toll-like receptor 4 (TLR4) and nod-like receptor family pyrin domain-containing 3 (NLRP3) expression. In IgAN rats, TP decrease the levels of TNF-α, IL-17A, IFN-γ, and IL-4 in serum, reduce the expression of IL-1β, Caspase-1, IL-18, and NLRP3 in renal tissues (46).
In MRL/lpr lupus mice, TP also inhibition of inflammatory response, ameliorate renal damage, and the mediated by JAK1/STAT1 pathway is a possible molecular mechanism (47).
Zhang et al. (48) have shown that (5R)-5-hydroxytriptolide (LLDT-8, a TP derivative) provides therapeutic benefits to LN by suppressing chemokine expression and inhibiting immune cell infiltration in the kidneys of MRL/lpr mice. Moreover, LLDT-8 inhibits inflammation in the kidneys by downregulating the cytokines IL-6, IL-17, TNF-α, and IFN-γ and upregulating FcγRIIB in the kidneys of a murine anti-glomerular basement membrane (GBM) glomerulonephritis model (49) (Table 1, Figures 2, 3).
Antioxidative Stress Effects
TP effectively attenuates the levels of blood glucose, Scr and proteinuria by reducing the expression of cyclooxygenase-2 (COX-2) and inducible nitric oxide synthase (iNOS) in renal tissues of DN rats (50). NF-κB is a redox-sensitive transcription factor that responds to ROS at various sites within the signaling pathway such as by activating or inactivating the inhibitory κB kinase complex, which, in turn, affects downstream targets or activates NF-κB via alternative inhibitor κBα phosphorylation (96). TP protects glomerular endotheliocytes of DN by inhibiting the expression of NF-κB, iNOS, endothelial nitric oxide synthase (eNOS), and VEGF (51).
RhoA, a redox sensitive master regulator protein, regulates numerous biological functions (97). Due to lipid peroxidation is a major form of oxidative stress in diabetes, restoring normal RhoA activity levels prevents podocyte loss and consequent proteinuria in DN (98). Zheng et al. (52)concluded that TP ameliorated puromycin amino nucleoside-mediated podocyte injury by suppressing ROS generation and p38 mitogen-activated protein kinase activation while restoring RhoA signaling activity in vivo and in vitro (Table 1, Figures 2, 3).
Anti-fibrosis Effects
The Notch1 signaling plays a core role in the formation of mesangial cells during kidney development, and exacerbates renal tubulointerstitial fibrosis in DN (99). Han et al. (19) declared that TP has anti-glomerulosclerosis effects by suppressing miR-137/Notch1 pathway in DN rats. In addition, renal fibrosis can be regulated through autophagy, a biological regulatory program that maintains homeostasis (100). Phosphatase and tensin homolog deleted on chromosome ten (PTEN) plays an essential role in regulating of AKT/ mammalian target of rapamycin (mTOR) signaling (101). Li et al. (53) found that TP alleviates renal fibrosis by restoring autophagy through the miR-141-3p/PTEN/AKT/mTOR pathway in DN rats (Table 1, Figures 2, 3).
Autophagy Regulatory Effects
Autophagy is a highly conserved and lysosome-dependent bulk degradative pathway that participates in the clearance of damaged organelles and proteins, as well as in maintaining homeostasis in tubules and glomeruli (102). Deficiency in autophagy aggravates DN in rodent models. STZ-induced autophagy-deficient mice develop severe microalbuminuria, endothelial lesions, and podocyte damage (103). High-fat diet-induced podocyte-specific autophagy-deficient mice develop hyperglycemia with proteinuria and podocyte damage. Autophagy contributes to the degradation of AGEs and suppresses inflammation in the kidneys (104). Moreover, increased ROS enhances autophagy by controlling the activity of Atg4, a family of cysteine proteases that is essential for autophagy formation (105). ROS promotes autophagy through the activation of AMP-activated protein kinase (AMPK), likely via suppression of mTOR (106). Experimental evidence has shown that autophagy acts as a double-edged sword with regard to cell death and survival because it is accompanied by other forms of cell death such as apoptosis (107). The ratio of LC3 I to LC3 II is closely correlated with the extent of autophagosome formation; therefore, LC3 II could be a marker of autophagic activity (108). In STZ-induced rats, TP decrease the expression of LC3 II, inhibite autophagy by upregulating PI3K/Akt/mTOR pathway (54). In puromycin amino nucleotide-cultured podocytes, TP reduces podocyte injury via the mTOR-autophagy pathway to increase autophagy levels and facilitates podocyte recovery from injury (55). Autophagy may be regulated by mTOR complex 1 (mTORC1) (109). Haploinsufficiency of mTORC1 in podocytes or administration of rapamycin (a mTORC1 inhibitor), resulting in the activation of autophagy, has been shown to prevent progressive DN (106). Conversely, the activation of mTORC1 in podocytes, which results in the inhibition of autophagy, leads to accelerated DN (110). Furthermore, Liang et al. found that TP protects podocyte autophagy by suppressing the mTOR and AKT pathways in IgAN (56) (Table 1, Figures 2, 3).
Anti-podocyte Apoptosis Effects
In glucose and TGFβ1-cultured mouse podocytes, TP protected podocytes against diabetic milieu-elicited injury, mitigated cytoskeleton derangement, and preserved podocyte filtration barrier function via inhibiting phosphorylation of GSK3β (57). In glucose-cultured mouse podocytes, TP increases renal synaptopodin, desmin, and nephrin expressions to ameliorate podocyte injury (58, 59). Similarly, TP could significantly decrease proteinuria and upregulate nephrin and podocin mRNA and protein expression in rats with IgAN, suggesting that TP could reduce podocyte injury and repair glomerular filtration membrane barrier damage (60) (Table 1, Figures 2, 3).
Effects, Mechanisms, and Therapeutic Targets of Celastrol Against Proteinuria and Kidney Injury in DN
Anti-inflammatory Effects
As one of triterpenes in TWHF, Celastrol reduces levels of Scr, BUN and proteinuria, inhibits inflammation by regulating MAPK/NF-κB pathway in STZ-induced rats (33). In db/db mice, Celastrol improves insulin resistance and attenuates renal injury by inhibiting the NF-κB-mediated inflammatory (61) (Table 1, Figures 2, 3).
Autophagy Regulatory Effects
The PI3K/AKT pathway is one of the most important signaling pathways that regulate autophagy, and phosphorylated AKT can promote the formation of p-mTOR to inhibit cell autophagy (111). In STZ-induced rats, Celastrol attenuates renal injury by promoting the PI3K/AKT pathway to activate autophagy (62). As a proverbial cytoprotective enzyme, heme oxygenase-1 (HO-1) ameliorates cell injury and inflammation in podocytes via activating autophagy pathway. Celastrol protects against high glucose-induced podocyte injury by restoring HO-1-mediated autophagy pathway (63) (Table 1, Figures 2, 3).
Other Effects of TWHF and Its Main Bioactive Ingredients
Glomerular hypertension and tubulointerstitial hypoxia occur following DN, causing loss of glomerular integrity and tubular damage (112). Hypoxia inducible factor 1 α (HIF-1α) plays a regulatory role in cellular response to renal hypoxia. Chen et al. (64) drew a conclusion that TWPs decreased levels of Scr, BUN, 24-h UAlb, mean glomerular area and mean glomerular volume; improved renal histopathology; and down-regulated the expression of HIF-1α and endothelin-1 mRNA and protein in the kidneys of diabetic rats. HIF-1α activation under hypoxia could upregulate downstream glucose transporter 1 (GLUT-1) gene (113). TWPs and TP significantly reduce proteinuria and GLUT-1 levels in glomerular mesangial and epithelial cells of DN rats (65, 66).
Wnt/β-catenin signaling is an evolutionary conserved signaling pathway, which plays a core role in modulating kidney injury and repair (114). In DN rats, Chang et al. drew a conclusion that TWHF mitigates hyperglycemia-induced upregulated Wnt-1 and β-catenin expression in kidney tissues and ameliorates kidney injury (67) (Table 1, Figures 2, 3).
Conclusions
In this review, we have summarized currently available information on the effects of TWHF on DN. Experimental studies have demonstrated that TWHF interacts with a wide range of cellular processes such as inflammation, oxidative stress, fibrosis, apoptosis, autophagy, and podocytes, indicating that these mechanisms are involved in a variety of cellular signals. Although several genes and proteins involved in the effect of TWHF on cells and tissues have been identified, many of the targets and exact mechanisms participating in these events remain unknown. Further studies regarding the mechanism of DN with TWHF treatment are thus warranted. Its narrow therapeutic window and severe side effects restrict its clinical applications (26, 27). Therefore, hepatotoxicity and sexual inhibition may occur among patients who have used TWHF long term, thus requiring regular monitoring, and if necessary, a reduction in dose or possibly termination of its use.
Author Contributions
PL, JZ, D-QC, and XQ mainly drafted the work critical for important intellectual content. YW, ZS, and CW finished the discussion. PL and JZ contributed equally to this work. All authors contributed to the article and approved the submitted version.
Funding
This work was supported by supported by Research Projects of the National Natural Science Foundation of China (No. 81904174), China Postdoctoral Science Foundation (No. 2021M693579), and National Training Program for Innovative Key Talents of Traditional Chinese Medicine (No. 2019-128).
Conflict of Interest
The authors declare that the research was conducted in the absence of any commercial or financial relationships that could be construed as a potential conflict of interest.
Publisher's Note
All claims expressed in this article are solely those of the authors and do not necessarily represent those of their affiliated organizations, or those of the publisher, the editors and the reviewers. Any product that may be evaluated in this article, or claim that may be made by its manufacturer, is not guaranteed or endorsed by the publisher.
References
1. Srivastava SP, Zhou H, Setia O, Liu B, Kanasaki K, Koya D, et al. Loss of endothelial glucocorticoid receptor accelerates diabetic nephropathy. Nat Commun. (2021) 12:2368. doi: 10.1038/s41467-021-22617-y
2. Cundy T, Holden A, Stallworthy E. Early worsening of diabetic nephropathy in type 2 diabetes after rapid improvement in chronic severe hyperglycemia. Diabetes Care. (2021) 44:e55–6. doi: 10.2337/dc20-2646
3. Zhang L, Long J, Jiang W, Shi Y, He X, Zhou Z, et al. Trends in chronic kidney disease in China. N Engl J Med. (2016) 375:905–6. doi: 10.1056/NEJMc1602469
4. Saeedi P, Petersohn I, Salpea P, Malanda B, Karuranga S, Unwin N, et al. Global and regional diabetes prevalence estimates for 2019 and projections for 2030 and 2045: results from the International Diabetes Federation Diabetes Atlas, 9(th) edition. Diabetes Res Clin Pract. (2019) 157:107843. doi: 10.1016/j.diabres.2019.107843
5. Alder H, Ambühl PM. Managing diabetic kidney disease. Ther Umsch. (2020) 77:333–8. doi: 10.1024/0040-5930/a001200
6. Anders HJ, Huber TB, Isermann B, Schiffer M. CKD in diabetes: diabetic kidney disease versus nondiabetic kidney disease. Nat Rev Nephrol. (2018) 14:361–77. doi: 10.1038/s41581-018-0001-y
7. Chen C, Wang C, Hu C, Han Y, Zhao L, Zhu X, et al. Normoalbuminuric diabetic kidney disease. Front Med. (2017) 11:310–8. doi: 10.1007/s11684-017-0542-7
8. Alicic RZ, Cox EJ, Neumiller JJ, Tuttle KR. Incretin drugs in diabetic kidney disease: biological mechanisms and clinical evidence. Nat Rev Nephrol. (2021) 17:227–44. doi: 10.1038/s41581-020-00367-2
9. Hartman RE, Rao P, Churchwell MD, Lewis SJ. Novel therapeutic agents for the treatment of diabetic kidney disease. Expert Opin Investig Drugs. (2020) 29:1277–93. doi: 10.1080/13543784.2020.1811231
10. Association AD. 11. Microvascular Complications and Foot Care: standards of medical care in diabetes-2020. Diabetes Care. (2020) 43(Suppl. 1):S135–51. doi: 10.2337/dc20-S011
11. Sun GD, Li CY, Cui WP, Guo QY, Dong CQ, Zou H, et al. Review of herbal traditional chinese medicine for the treatment of diabetic nephropathy. J Diabetes Res. (2016) 2016:5749857. doi: 10.1155/2016/5749857
12. Yang X, Zhang B, Lu X, Yan M, Wen Y, Zhao TT, et al. Effects of Tangshen Formula on urinary and plasma liver-type fatty acid binding protein levels in patients with type 2 diabetic kidney disease: post-hoc findings from a multi-center, randomized, double-blind, placebo-controlled trial investigating the efficacy and safety of Tangshen Formula in patients with type 2 diabetic kidney disease. BMC Complement Altern Med. (2016) 16:246. doi: 10.1186/s12906-016-1228-4
13. Li Y, Wang J, Xiao Y, Wang Y, Chen S, Yang Y, et al. A systems pharmacology approach to investigate the mechanisms of action of Semen Strychni and Tripterygium wilfordii Hook F for treatment of rheumatoid arthritis. J Ethnopharmacol. (2015) 175:301–14. doi: 10.1016/j.jep.2015.09.016
14. Law SK, Simmons MP, Techen N, Khan A, He MF, Shaw PC, et al. Molecular analyses of the Chinese herb Leigongteng (Tripterygium wilfordii Hook.f.). Phytochemistry. (2011) 72:21–6. doi: 10.1016/j.phytochem.2010.10.015
15. Graziose R, Lila MA, Raskin I. Merging traditional Chinese medicine with modern drug discovery technologies to find novel drugs and functional foods. Curr Drug Discov Technol. (2010) 7:2–12. doi: 10.2174/157016310791162767
16. Wang X, Zu Y, Huang L, Yu J, Zhao H, Wen C, et al. Treatment of rheumatoid arthritis with combination of methotrexate and Tripterygium wilfordii: a meta-analysis. Life Sci. (2017) 171:45–50. doi: 10.1016/j.lfs.2017.01.004
17. Sun YY, Xiao L, Wang D, Ji YC, Yang YP, Ma R, et al. Triptolide inhibits viability and induces apoptosis in liver cancer cells through activation of the tumor suppressor gene p53. Int J Oncol. (2017) 50:847–52. doi: 10.3892/ijo.2017.3850
18. Zhu W, Li Y, Gong J, Zuo L, Zhang W, Cao L, et al. Tripterygium wilfordii Hook. f. versus azathioprine for prevention of postoperative recurrence in patients with Crohn's disease: a randomized clinical trial. Dig Liver Dis. (2015) 47:14–9. doi: 10.1016/j.dld.2014.09.008
19. Han F, Wang S, Chang Y, Li C, Yang J, Han Z, et al. Triptolide prevents extracellular matrix accumulation in experimental diabetic kidney disease by targeting microRNA-137/Notch1 pathway. J Cell Physiol. (2018) 233:2225–37. doi: 10.1002/jcp.26092
20. Zhou Y, Hong Y, Huang H. Triptolide attenuates inflammatory response in membranous glomerulo-nephritis rat via downregulation of NF-κB signaling pathway. Kidney Blood Press Res. (2016) 41:901–10. doi: 10.1159/000452591
21. Liu Q. Clinical study on clinical effects of multiple target therapy on renal function of patients with refractory adult nephrotic syndrome. Med J Natl Defend Forces Southw China. (2016) 26:511–3.
22. Wang D, Zhao XH, Cui Y, Zhang TT, Wang F, Hu YH. Efficacy and safety of Tripterygium wilfordii Hook F. for CKD in Mainland China: a systematic review and meta-analysis. Phytother Res. (2018) 32:436–51. doi: 10.1002/ptr.5987
23. Chang X, Li L, Wang B, Huang C, Liu XW, Liu HB, et al. Evaluation of the efficacy and safety of TWHF in diabetic nephropathy patients with overt proteinuria and normal eGFR. J Formos Med Assoc. (2020) 119:685–92. doi: 10.1016/j.jfma.2019.11.001
24. Xu LN, Zhao B, Wang HT, Liu LL, Chen AQ, Wang H, et al. Tripterygium wilfordii Hook F. treatment for stage IV diabetic nephropathy: protocol for a prospective, randomized controlled trial. Biomed Res Int. (2020) 2020:9181037. doi: 10.1155/2020/9181037
25. Wang Y, Liu T, Ma F, Lu X, Mao H, Zhou W, et al. A network pharmacology-based strategy for unveiling the mechanisms of Tripterygium Wilfordii Hook F. against diabetic kidney disease. J Diabetes Res. (2020) 2020:2421631. doi: 10.1155/2020/2421631
26. Kupchan SM, Court WA, Dailey RJ, Gilmore CJ, Bryan RF, et al. Triptolide and tripdiolide, novel antileukemic diterpenoid triepoxides from Tripterygium wilfordii. J Am Chem Soc. (1972) 94:7194–5. doi: 10.1021/ja00775a078
27. Chen SR, Dai Y, Zhao J, Lin L, Wang Y, Wang Y, et al. A mechanistic overview of triptolide and celastrol, natural products from Tripterygium wilfordii Hook F. Front Pharmacol. (2018) 9:104. doi: 10.3389/fphar.2018.00104
28. Yao JR, Sun Y, Luo SK, Xie DH. Progress in clinical application of polyglycosides of Tripterygium wilfordii. Chin J N Drugs Clin Med. (2010) 29:179–82.
29. Trott A, West JD, Klaić L, Westerheide SD, Silverman RB, Morimoto RI, et al. Morano: activation of heat shock and antioxidant responses by the natural product celastrol: transcriptional signatures of a thiol-targeted molecule. Mol Biol Cell. (2008) 19:1104–12. doi: 10.1091/mbc.e07-10-1004
30. Liu Y, Bai H, Liu J, Chu Y, Yuan XH. protective effect of tripterygium glycosides on renal inflammatory injury in diabetic nephropathy rats. Guangming J Chin Med. (2017) 32:1577–9.
31. Kong Y, Shan CY, Chang BC, Yang JH, Zheng MY, Ren HZ, et al. Effect of tripterygium wilfordii polyglucosides on the expression of tumor necrosis factor-α and interleukin-4 in renal tissue from rats with diabetic nephropathy. Chin J Diabete. (2013) 5:541–6. doi: 10.3760/cma.j.issn.1674-5809.2013.09.006
32. Meng B, Yan CQ, Cai SY. Protective effect of tripterygium glycosides on kidney tissue of rats with diabetic nephropathy. Chin J Clin Pharmacol. (2020) 10:1270–3. doi: 10.13699/j.cnki.1001-6821.2020.10.027
33. Zhang M, Chen Y, Yang MJ, Fan XR, Xie H, Zhang L, et al. Celastrol attenuates renal injury in diabetic rats via MAPK/NF-κB pathway. Phytother Res. (2019) 33:1191–8. doi: 10.1002/ptr.6314
34. Li W, Chen CY, Luo HL. Effect of tripterygium glycosides on the renal tissue inflammatory factor expression of chronic glomerulonephritis rats. Chin J Clin Pharmacol. (2020) 36:3030–2+3065. doi: 10.13699/j.cnki.1001-6821.2020.19.019
35. Yang ZM, Cai JY, Sun LY, Wang XS, Shen SZ. Effect of tripterygium glycosides tablets on kidney pathological damage and serum inflammatory factors expression in immunoglobulin. Chin J Clin Pharmacol. (2020) 36:2242–5. doi: 10.13699/j.cnki.1001-6821.2020.15.024
36. Zhang YX, Liu GL, Wang JQ, Li YC, Hu LW, Lu K. Antioxidative effect of Tripterygium wilfordii polyglycosides on diabetic rats. Chin J Pharmacol Toxicol. (2014) 28:358–61. doi: 10.3867/j.issn.1000-3002.2014.03.008
37. Zhang Y, Chang BS, Chen WD. Triptergium wilfordii polyglucoside interfere the expression of TGF-β1?BMP-7 and Gremlin in renal tissue of diabetic nephropathy rats. J Nephrol Dialy Transplant. (2012) 21:237–43.
38. Chang S, Xu XQ, Xiang H, Meng W, Zheng HY, Zhang H, et al. Effects of tripterygium glycosides on the renal injury in mice with diabetic nephropathy via Akt /mTOR signaling pathway. Hebei Med J. (2021) 1:25–9. doi: 10.3969/j.issn.1002-7386.2021.01.005
39. Song CD, Song D, Ren XQ, Zhai WS, Ding Y. Effects of tripeygium wilfordi multiglucoside tablets on expressions of RhoA and ROCK1 in RENAL tissue of rats with diabetic nephropathy. Chin J Tradition Chin Med. (2020) 8:166–9+280. doi: 10.13193/j.issn.1673-7717.2020.08.040
40. Liu YN, Ma H, Jia, Huang L. Expression of microRNA-192 in kidney interstitium in young rats with unilateral ureteral obstruction and in-tervention with tripterygium wilfordii polyglycoside. Chin Remed Clin. (2014) 14:1019–21. doi: 10.11655/zgywylc2014.08.004
41. Li L, Zhao R, Li CJ, Sun B, Yang W, Ma ZJ, et al. Protective effects of tripterygium wilfordii polyglycosides on kidney of diabetic rats. Chin J Diabetes. (2016) 24:459–64. doi: 10.3969/j.issn.1006-6187.2016.05.017
42. Wang JJ, Hu Y, Mao YY, Zhou N. Effect of triptergium glycosides on podocyte injury in rats with Adriamycin- induced nephropathy. Shaanxi Med J. (2011) 40:119–22+1273. doi: 10.3969/j.issn.1000-7377.2011.09.004
43. Jiang C, Chen YX, Qin SK, Yang AZ, Ma XQ, Cheng Y, et al. Research on the mice podocyte apoptosis and expression of the associated protein caused by TWP intervene sunitinib. Acta Univ Med Anhui. (2016) 51:800–4.
44. Ma R, Liu L, Liu X, Wang Y, Jiang W, Xu L. Triptolide markedly attenuates albuminuria and podocyte injury in an animal model of diabetic nephropathy. Exp Ther Med. (2013) 6:649–56. doi: 10.3892/etm.2013.1226
45. He L, Peng X, Liu G, Tang C, Liu H, Liu F, et al. Anti-inflammatory effects of triptolide on IgA nephropathy in rats. Immunopharmacol Immunotoxicol. (2015) 37:421–7. doi: 10.3109/08923973.2015.1080265
46. Zhou H, Qu SY, Tian LD. Effect of triptolide on renal protection and nlrp3 inflammasome in IgA nephropathy rats. J Bengbu Med Coll. (2020) 12:1593–7+1601. doi: 10.13898/j.cnki.issn.1000-2200.2020.12.001
47. Duan R, Wu YH, Liu W. Effect and mechanism of Triptolide on ameliorating renal injury of MRL/lpr lupus mice. China J Modern Med. (2021) 31:7–12.
48. Zhang LY, Li H, Wu YW, Cheng L, Yan YX, Yang XQ, et al. (5R)-5-hydroxytriptolide ameliorates lupus nephritis in MRL/lpr mice by preventing infiltration of immune cells. Am J Physiol Renal Physiol. (2017) 312:F769–77. doi: 10.1152/ajprenal.00649.2016
49. Qi Q, Li H, Lin ZM, Yang XQ, Zhu FH, Liu YT, et al. (5R)-5-hydroxytriptolide ameliorates anti-glomerular basement membrane glomerulonephritis in NZW mice by regulating Fcγ receptor signaling. Acta Pharmacol Sin. (2018) 39:107–16. doi: 10.1038/aps.2017.88
50. Wang DN, Yu R. Effects of triptolide on expressions of iNOS and COX-2 in renal tissue of diabetic nephropathy rats. Progr Anatom Sci. (2017) 23:148–50. doi: 10.16695/j.cnki.1006-2947.2017.02.011
51. Liu Q, Chen SJ, Liu FH, Wang XJ, Shi ZW, Chen HP. Effects of triptolide on expressions of NF- κB, NOS and VEGF in glomeruli of diabetes mellitus rats. J Clin Exp Med. (2014) 13:1925–9. doi: 10.3969/j.issn.1671-4695.2014.23.002
52. Zheng CX, Chen ZH, Zeng CH, Qin WS, Li LS, Liu ZH. Triptolide protects podocytes from puromycin aminonucleoside induced injury in vivo and in vitro. Kidney Int. (2008) 74:596–612. doi: 10.1038/ki.2008.203
53. Li XY, Wang SS, Han Z, Han F, Chang YP, Yang Y, et al. triptolide restores autophagy to alleviate diabetic renal fibrosis through the miR-141–3p/PTEN/Akt/mTOR pathway. Mol Ther Nucleic Acids. (2017) 9:48–56. doi: 10.1016/j.omtn.2017.08.011
54. Tang XT, Xu J, Wang LY, Yu Y, Cui XB, Sun WY. Effect of triptolide on autophagy and apoptosis of renal podocytes in diabetic nephropathy mice. World Chin Med. (2019) 10:2623–26.
55. Liu LQ, Wang K, Han RH, Song Q. Effects of triptolide on the level of autophagy in PAN cultured podocytes. Chin J Integr Tradition Western Nephrol. (2015) 16:1056–8.
56. Liang S, Jin J, Shen X, Jiang X, Li Y, He Q. Triptolide protects podocytes via autophagy in immunoglobulin A nephropathy. Exp Ther Med. (2018) 16:2275–80. doi: 10.3892/etm.2018.6480
57. Liang X, Chen B, Wang P, Ge Y, Malhotra DK, Dworkin LD, et al. Triptolide potentiates the cytoskeleton-stabilizing activity of cyclosporine A in glomerular podocytes via a GSK3β dependent mechanism. Am J Transl Res. (2020) 12:800–12.
58. Xu XP, Hong YZ, Ye X. Effects of triptol ide intervention in high glucose environment on expression of podocyte nephrin proteins. Chin J Integr Tradition Chin Western Med Nephropathy. (2010) 11:872–4. doi: 10.3969/j.issn.1009-587X.2010.10.009
59. Zhao XL, Liu QX. Effect of Triptolide on podocyte synaptopodin and desmin expression in high glucose environment. J Qingdao Univ Med Coll. (2015) 51:134–7. doi: 10.13361/j.qdyxy.201502003
60. Zhu CF, Zhu B, Bao ZR, Li XF, Chen HY, Shen FP. Effect of triptolide on proteinuria, the expression of proteins and mRNA of nephrin and podocin in rats with IgAN. Chin J Integr Tradition Western Nephrol. (2015) 16:106–9.
61. Kim JE, Lee MH, Nam DH, Song HK, Kang YS, Lee JE, et al. Celastrol, an NF-κB inhibitor, improves insulin resistance and attenuates renal injury in db/db mice. PLoS ONE. (2013) 8:e62068. doi: 10.1371/journal.pone.0062068
62. Nie Y, Fu C, Zhang H, Zhang M, Xie H, Tong X, et al. Celastrol slows the progression of early diabetic nephropathy in rats via the PI3K/AKT pathway. BMC Compl Med Ther. (2020) 20:321. doi: 10.1186/s12906-020-03050-y
63. Zhan X, Yan C, Chen Y, Wei X, Xiao J, Deng L, et al. Celastrol antagonizes high glucose-evoked podocyte injury, inflammation and insulin resistance by restoring the HO-1-mediated autophagy pathway. Mol Immunol. (2018) 104:61–8. doi: 10.1016/j.molimm.2018.10.021
64. Chen WD, Chang BC, Zhang Y, Yang P, Liu L. Effect of Tripterygium glycosides on expression of hypoxia inducible factor- 1α and endothelin-1 in kidney of diabetic rats. J South Med Univ. (2015) 499–505.
65. Li YC, Liu GL, Zhang YX, Ye WZ, Chang JZ, Shen YJ. Effect of tripterygium wilfordii glycosides on expression of GLUT-1 in kidney of diabetic rats. Chin J Cell Mol Immunol. (2012) 709–10.
66. You LJ, Guo H, Li YC. Influence of triptolide on the expression of Glut-1,Glut-4 in kidney of diabetic nephropathy rats. Pract Pharm Clin Remed. (2015) 18:390–3.
67. Chang B, Chen W, Zhang Y, Yang P, Liu L. Tripterygium wilfordii mitigates hyperglycemia-induced upregulated Wnt/β-catenin expression and kidney injury in diabetic rats. Exp Ther Med. (2018) 15:3874–82. doi: 10.3892/etm.2018.5901
68. Schmidt IM, Srivastava A, Sabbisetti V, McMahon GM, He J, Chen J, et al. Plasma kidney injury molecule 1 in CKD: findings From the boston kidney biopsy cohort and CRIC studies. Am J Kidney Dis. (2021) 7:S0272–6386. doi: 10.1053/j.ajkd.2021.05.013
69. Yang M, Wang X, Han Y, Li C, Wei L, Yang J, et al. Targeting the NLRP3 inflammasome in diabetic nephropathy. Curr Med Chem. (2021) 28. doi: 10.2174/0929867328666210705153109. [Epub ahead of print].
70. Eisa NH, Khodir AE, El-Sherbiny M, Elsherbiny NM, Said E. Phenethyl isothiocyanate attenuates diabetic nephropathy via modulation of glycative/oxidative/inflammatory signaling in diabetic rats. Biomed Pharmacother. (2021) 12:111666. doi: 10.1016/j.biopha.2021.111666
71. Pace J, Paladugu P, Das B, He JC, Mallipattu SK. Targeting STAT3 signaling in kidney disease. Am J Physiol Renal Physiol. (2019) 316:F1151–61. doi: 10.1152/ajprenal.00034.2019
72. Li X, Wang Y, Wang K, Wu Y. Renal protective effect of Paeoniflorin by inhibition of JAK2/STAT3 signaling pathway in diabetic mice. Biosci Trends. (2018) 12:168–76. doi: 10.5582/bst.2018.01009
73. Vermot A, Petit-Härtlein I, Smith S, Fieschi F. NADPH Oxidases (NOX): an overview from discovery, molecular mechanisms to physiology and pathology. Antioxidants. (2021) 10:890. doi: 10.3390/antiox10060890
74. Zhang Q, Hu Y, Hu JE, Ding Y, Shen Y, Xu H, et al. Sp1-mediated upregulation of Prdx6 expression prevents podocyte injury in diabetic nephropathy via mitigation of oxidative stress and ferroptosis. Life Sci. (2021) 278:119529. doi: 10.1016/j.lfs.2021.119529
75. Huang C, Xue LF, Hu B, Liu HH, Huang SB, Khan S, et al. Calycosin-loaded nanoliposomes as potential nanoplatforms for treatment of diabetic nephropathy through regulation of mitochondrial respiratory function. J Nanobiotechnol. (2021) 19:178. doi: 10.1186/s12951-021-00917-1
76. Gezginci-Oktayoglu S, Coskun E, Ercin M, Bolkent S. 4-Methylcatechol prevents streptozotocin-induced acute kidney injury through modulating NGF/TrkA and ROS-related Akt/GSK3β/β-catenin pathways. Int Immunopharmacol. (2018) 64:52–9. doi: 10.1016/j.intimp.2018.08.017
77. Wang Q, Dai X, Xiang X, Xu Z, Su S, Wei D, et al. A natural product of acteoside ameliorate kidney injury in diabetes db/db mice and HK-2 cells via regulating NADPH/oxidase-TGF-β/Smad signaling pathway. Phytother Res. (2021). doi: 10.1002/ptr.7196. [Epub ahead of print].
78. Liu T, Chen XM, Sun JY, Jiang XS, Wu Y, Yang S, et al. Palmitic acid-induced podocyte apoptosis via the reactive oxygen species-dependent mitochondrial pathway. Kidney Blood Press Res. (2018) 43:206–19. doi: 10.1159/000487673
79. Hou Y, Shi Y, Han B, Liu X, Qiao X, Qi Y, et al. The antioxidant peptide SS31 prevents oxidative stress, downregulates CD36 and improves renal function in diabetic nephropathy. Nephrol Dial Transplant. (2018) 33:1908–18. doi: 10.1093/ndt/gfy021
80. Wang B, Ding X, Ding C, Tesch G, Zheng J, Tian P, et al. WNT1-inducible-signaling pathway protein 1 regulates the development of kidney fibrosis through the TGF-β1 pathway. FASEB J. (2020) (2020) 34:14507–20. doi: 10.1096/fj.202000953R
81. Zeng LF, Xiao Y, Sun L. A glimpse of the mechanisms related to renal fibrosis in diabetic nephropathy. Adv Exp Med Biol. (2019) 1165:49–79. doi: 10.1007/978-981-13-8871-2_4
82. Salem RM, Todd JN, Sandholm N, Cole JB, Chen WM, Andrews D, et al. Genome-wide association study of diabetic kidney disease highlights biology involved in glomerular basement membrane collagen. J Am Soc Nephrol. (2019) 30:2000–16. doi: 10.1681/ASN.2019030218
83. Li Y, Hu Q, Li C, Liang K, Xiang Y, Hsiao H, et al. PTEN-induced partial epithelial-mesenchymal transition drives diabetic kidney disease. J Clin Invest. (2019) 129:1129–51. doi: 10.1172/JCI121987
84. Lv W, Booz GW, Fan F, Wang Y, Roman RJ. oxidative stress and renal fibrosis: recent insights for the development of novel therapeutic strategies. Front Physiol. (2018) 9:105. doi: 10.3389/fphys.2018.00105
85. Ke B, Zhu N, Luo F, Xu Y, Fang X. Targeted inhibition of endoplasmic reticulum stress: new hope for renal fibrosis (Review). Mol Med Rep. (2017) 16:1014–20. doi: 10.3892/mmr.2017.6762
86. Tian J, Zhang M, Suo M, Liu D, Wang X, Liu M, et al. Dapagliflozin alleviates cardiac fibrosis through suppressing EndMT and fibroblast activation via AMPKα/TGF-β/Smad signalling in type 2 diabetic rats. J Cell Mol Med. (2021) 25:7642–59. doi: 10.1111/jcmm.16601
87. Xiao Y, Jiang X, Peng C, Zhang Y, Xiao Y, Liang D, et al. BMP-7/Smads-induced inhibitor of differentiation 2 (Id2) upregulation and Id2/Twist interaction was involved in attenuating diabetic renal tubulointerstitial fibrosis. Int J Biochem Cell Biol. (2019) 116:105613. doi: 10.1016/j.biocel.2019.105613
88. Feng Y, Jin Y, Liu DW, Wei L. Bone morphogenetic protein (BMP) 7 expression is regulated by the E3 ligase UBE4A in diabetic nephropathy. Arch Physiol Biochem. (2020) 126:416–9. doi: 10.1080/13813455.2018.1551905
89. Martinez B, Peplow PV. MicroRNAs as biomarkers of diabetic retinopathy and disease progression. Neural Regen Res. (2019) 14:1858–69. doi: 10.4103/1673-5374.259602
90. Tang J, Yao D, Yan H, Chen X, Wang L, Zhan H. The role of microRNAs in the pathogenesis of diabetic nephropathy. Int J Endocrinol. (2019) 2019:8719060. doi: 10.1155/2019/8719060
91. Novelli R, Benigni A, Remuzzi G. The role of B7-1 in proteinuria of glomerular origin. Nat Rev Nephrol. (2018) 14:589–96. doi: 10.1038/s41581-018-0037-z
92. Verheijden K, Sonneveld R, Bakker-van M, Wetzels J, van der Vlag J, Nijenhuis T. The calcium-dependent protease calpain-1 links TRPC6 activity to podocyte injury. J Am Soc. Nephrol. (2018) 29:2099–109. doi: 10.1681/ASN.2016111248
93. Senouthai S, Wang J, Fu D, You Y. Fractalkine is involved in lipopolysaccharide-induced podocyte injury through the Wnt/β-catenin pathway in an acute kidney injury mouse model. Inflammation. (2019) 42:1287–300. doi: 10.1007/s10753-019-00988-1
94. Russo ER, Facincani I, Nakazato KC, Coimbra TM, Crevelin EJ, Pereira A, et al. Oral administration of powdered dried rhizomes of Curcuma longa L. (turmeric, Zingiberaceae) is effective in the treatment of doxorubicin-induced kidney injury in rats. Phytother Res. (2018) 32:2408–16. doi: 10.1002/ptr.6176
95. Liu X, Wang K, Duan N, Lan Y, Ma P, Zheng H. Computational prediction and experimental validation of low-affinity target of triptolide and its analogues. RSC Adv. (2015) 5:34572–9. doi: 10.1039/C4RA17009A
96. Forrester SJ, Kikuchi DS, Hernandes MS, Xu Q, Griendling KK. reactive oxygen species in metabolic and inflammatory signaling. Circ Res. (2018) 122:877–902. doi: 10.1161/CIRCRESAHA.117.311401
97. Song R, Lei S, Yang S, Wu SJ. LncRNA PAXIP1-AS1 fosters the pathogenesis of pulmonary arterial hypertension via ETS1/WIPF1/RhoA axis. J Cell Mol Med. (2021) 25:7321–34. doi: 10.1111/jcmm.16761
98. Zheng W, Qian C, Xu F, Cheng P, Yang C, Li X, et al. Fuxin Granules ameliorate diabetic nephropathy in db/db mice through TGF-β1/Smad and VEGF/VEGFR2 signaling pathways. Biomed Pharmacother. (2021) 141:111806. doi: 10.1016/j.biopha.2021.111806
99. Zhu QQ, Yang XY, Zhang XJ, Yu CJ, Pang QQ, Huang YW, et al. EGCG targeting Notch to attenuate renal fibrosis via inhibition of TGFβ/Smad3 signaling pathway activation in streptozotocin-induced diabetic mice. Food Funct. (2020) 11:9686–95. doi: 10.1039/D0FO01542C
100. Ding Y, Choi ME. Regulation of autophagy by TGF-β: emerging role in kidney fibrosis. Semin Nephrol. (2014) 34:62–71. doi: 10.1016/j.semnephrol.2013.11.009
101. Song N, Zhang T, Xu X, Lu Z, Yu X, Fang Y, et al. miR-21 protects against ischemia/reperfusion-induced acute kidney injury by preventing epithelial cell apoptosis and inhibiting dendritic cell maturation. Front Physiol. (2018) 9:790. doi: 10.3389/fphys.2018.00790
102. Koch E, Nakhoul R, Nakhoul F, Nakhoul N. Autophagy in diabetic nephropathy: a review. Int Urol Nephrol. (2020) 52:1705–12. doi: 10.1007/s11255-020-02545-4
103. Tagawa A, Yasuda M, Kume S, Yamahara K, Nakazawa J, Chin-Kanasaki M, et al. Impaired podocyte autophagy exacerbates proteinuria in diabetic nephropathy. Diabetes. (2016) 65:755–67. doi: 10.2337/db15-0473
104. Takahashi A, Takabatake Y, Kimura T, Maejima I, Namba T, Yamamoto T, et al. Autophagy inhibits the accumulation of advanced glycation end products by promoting lysosomal biogenesis and function in the kidney proximal tubules. Diabetes. (2017) 66:1359–72. doi: 10.2337/db16-0397
105. Audzeyenka I, Rogacka D, Piwkowska A, Rychlowski M, Bierla JB, Czarnowska E, et al. Reactive oxygen species are involved in insulin-dependent regulation of autophagy in primary rat podocytes. Int J Biochem Cell Biol. (2016) 75:23–33. doi: 10.1016/j.biocel.2016.03.015
106. Kim KY, Park KI, Kim SH, Yu SN, Park SG, Kim YW, et al. Inhibition of autophagy promotes salinomycin-induced apoptosis via reactive oxygen species-mediated pi3k/AKT/mTOR and ERK/p38 MAPK-dependent signaling in human prostate cancer cells. Int J Mol Sci. (2017) 18:1088. doi: 10.3390/ijms18051088
107. Jin J, Zhao L, Zou W, Shen W, Zhang H, He Q. Activation of cyclooxygenase-2 by ATF4 during endoplasmic reticulum stress regulates kidney podocyte autophagy induced by lupus nephritis. Cell Physiol Biochem. (2018) 48:753–64. doi: 10.1159/000491904
108. Vizza D, Perri A, Toteda G, Lupinacci S, Perrotta I, Lofaro D, Leone F, et al. Rapamycin-induced autophagy protects proximal tubular renal cells against proteinuric damage through the transcriptional activation of the nerve growth factor receptor NGFR. Autophagy. (2018) 14:1028–42. doi: 10.1080/15548627.2018.1448740
109. Bhayana S, Baisantry A, Kraemer TD, Wrede C, Hegermann J, Bräsen JH, et al. Autophagy in kidney transplants of sirolimus treated recipients. J Nephropathol. (2017) 6:90–6. doi: 10.15171/jnp.2017.15
110. Yao Y, Wang J, Yoshida S, Nada S, Okada M, Inoki K. Role of ragulator in the regulation of mechanistic target of rapamycin signaling in podocytes and glomerular function. J Am Soc Nephrol. (2016) 27:3653–65. doi: 10.1681/ASN.2015010032
111. Park JH, Kim KP, Ko JJ, Park KS. PI3K/Akt/mTOR activation by suppression of ELK3 mediates chemosensitivity of MDA-MB-231 cells to doxorubicin by inhibiting autophagy. Biochem Biophys Res Commun. (2016) 477:277–82. doi: 10.1016/j.bbrc.2016.06.057
112. Zhou Q, Gong X, Kuang G, Jiang R, Xie T, Tie H, et al. Ferulic acid protected from kidney ischemia reperfusion injury in mice: possible mechanism through increasing adenosine generation via HIF-1α. Inflammation. (2018) 41:2068–78. doi: 10.1007/s10753-018-0850-3
113. Nakaigawa N, Kondo K, Ueno D, Namura K, Makiyama K, Kobayashi K, et al. The acceleration of glucose accumulation in renal cell carcinoma assessed by FDG PET/CT demonstrated acquisition of resistance to tyrosine kinase inhibitor therapy. BMC Cancer. (2017) 17:39. doi: 10.1186/s12885-016-3044-0
Keywords: diabetic nephropathy, Tripterygium wilfordii Hook f., tripterygium wilfordii polyglycosides, triptolide, celastrol
Citation: Liu P, Zhang J, Wang Y, Shen Z, Wang C, Chen D-Q and Qiu X (2021) The Active Compounds and Therapeutic Target of Tripterygium wilfordii Hook. f. in Attenuating Proteinuria in Diabetic Nephropathy: A Review. Front. Med. 8:747922. doi: 10.3389/fmed.2021.747922
Received: 27 July 2021; Accepted: 25 August 2021;
Published: 21 September 2021.
Edited by:
Qin Zhou, The First Affiliated Hospital of Sun Yat-Sen University, ChinaReviewed by:
Liang Ma, Sichuan University, ChinaYingying Zhang, Tongji University School of Medicine, China
Copyright © 2021 Liu, Zhang, Wang, Shen, Wang, Chen and Qiu. This is an open-access article distributed under the terms of the Creative Commons Attribution License (CC BY). The use, distribution or reproduction in other forums is permitted, provided the original author(s) and the copyright owner(s) are credited and that the original publication in this journal is cited, in accordance with accepted academic practice. No use, distribution or reproduction is permitted which does not comply with these terms.
*Correspondence: Dan-Qian Chen, Y2hlbmRhbnFpYW4yMDEzQDE2My5jb20=; Xinping Qiu, cWl1NzcxMTE1QHNvaHUuY29t
†These authors have contributed equally to this work