- Key Laboratory of Aging and Cancer Biology of Zhejiang Province, School of Basic Medical Sciences, Hangzhou Normal University, Hangzhou, China
Idiopathic pulmonary fibrosis is an age-dependent progressive and fatal lung disease of unknown etiology, which is characterized by the excessive accumulation of extracellular matrix inside the interstitial layer of the lung parenchyma that leads to abnormal scar architecture and compromised lung function capacity. Recent genetic studies have attributed the pathological genes or genetic mutations associated with familial idiopathic pulmonary fibrosis (IPF) and sporadic IPF to telomere-related components, suggesting that telomere dysfunction is an important determinant of this disease. In this study, we summarized recent advances in our understanding of how telomere dysfunction drives IPF genesis. We highlighted the key role of alveolar stem cell dysfunction caused by telomere shortening or telomere uncapping, which bridged the gap between telomere abnormalities and fibrotic lung pathology. We emphasized that senescence-associated secretory phenotypes, innate immune cell infiltration, and/or inflammation downstream of lung stem cell dysfunction influenced the native microenvironment and local cell signals, including increased transforming growth factor-beta (TGF-β) signaling in the lung, to induce pro-fibrotic conditions. In addition, the failed regeneration of new alveoli due to alveolar stem cell dysfunction might expose lung cells to elevated mechanical tension, which could activate the TGF-β signaling loop to promote the fibrotic process, especially in a periphery-to-center pattern as seen in IPF patients. Understanding the telomere-related molecular and pathophysiological mechanisms of IPF would provide new insights into IPF etiology and therapeutic strategies for this fatal disease.
Introduction
Idiopathic pulmonary fibrosis is a progressive and fatal lung disease of unknown etiology. The pathological manifestation of this disease is the deposition of excessive extracellular matrix filaments in the interstitial space and gradual loss of alveolar epithelial cells in the lung parenchyma (1, 2). Once diagnosed, the average survival time is 3–5 years, and patients usually die of respiratory failure. At present, no efficient treatment options are available for this disease, and mortality is increasing every year (3). Recent progress has revealed that telomere dysfunction drives idiopathic pulmonary fibrosis (IPF) genesis. Understanding the role of telomere dysfunction in IPF would provide new insights into the early-stage diagnosis and the effective therapeutic strategies for this fatal disease.
Telomere Structure And Function And Cellular Senescence
Telomeres are ribonucleoprotein structural complexes located at eukaryotic chromosomal ends and are mainly composed of tandem DNA repeats (TTAGGG in humans) and their binding proteins. Telomeres protect genome integrity and prevent degradation and chromosomal end-to-end fusion (4). Telomeric DNA usually terminates with a single-stranded 3′ G-rich overhang, which folds back to form a t-loop lariat structure with telomeric double-stranded repeats (5). This structure of telomeric DNA ends is capped and protected by a specialized shelterin complex that comprises six protein subunits (4, 5) (Figure 1). The ablation or deficiency of any shelterin component can result in telomere dysfunction, which triggers a DNA damage response. In most somatic cells, the telomere shortens at each cell division due to the end replication problem. However, stem cells, including adult stem cells in organs, express telomerase to counteract this shortening by adding telomeric sequences to the ends. Telomerase mainly consists of the catalytic subunit telomerase reverse transcriptase (TERT) and telomerase RNA component (TERC). It also has other accessory components, such as the most characterized element dyskerin, which combines with the other three smaller proteins NHP2, NOP10, and GAR1 to stabilize the TERC (6) (Figure 1). Additional components have also been identified as involved in telomerase assembly, trafficking, and regulation (6–8). In humans, telomere length is negatively correlated with age. When shortened telomeres reach a critical threshold, cellular senescence, and/or apoptosis are triggered by the DNA damage response (9).
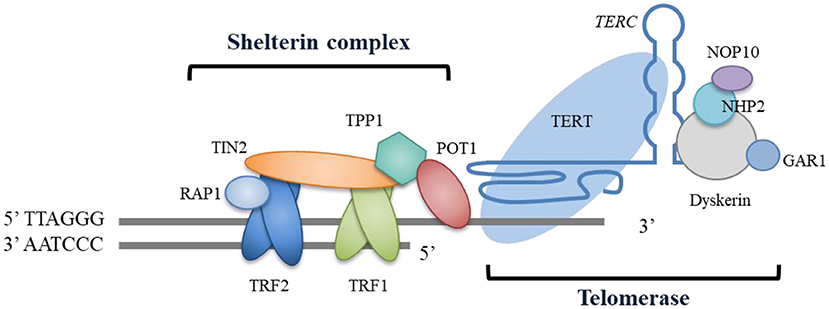
Figure 1. Schematic telomere structure and essential components. Human telomeric repeated DNA is protected by the shelterin complex, which consists of the protein components TRF1, TRF2, RAP1, TIN2, TPP1, and POT1. The ends of telomeres are bonded with telomerase, which is comprised of the catalytic subunit of TERT and the telomerase RNA component of TERC, and this exerts its effects by adding telomeric sequences to the telomeric ends. The telomerase also has other accessory components, including dyskerin, NHP2, NOP10, and GAR1. The function of these accessory components is to stabilize the TERC.
Cellular senescence, first described by Hayflick, is a cellular phenomenon of stable cell cycle arrest characterized by a series of dramatic changes, including those in cell morphology, metabolism, epigenome, and gene expression (10). Although cellular senescence is a state of proliferation arrest, senescent cells are still metabolically active, producing a distinct secretome with a variety of cytokines and chemokines, known as the senescence-associated secretory phenotype (SASP) (10–13). Cellular senescence can be triggered by telomere shortening, certain active oncogenes, oxidase stress, and embryonic developmental signals (10, 11, 13–15). Accumulating evidence suggests that cellular senescence plays a critical role in aging-associated diseases (10, 16, 17). In the lung tissue, several studies suggest that the cellular senescence of alveolar epithelial type 2 cells (AEC2s) is involved in the genesis of IPF (18–20).
Telomere Biology Disorders And Chronic Lung Diseases
Telomere biology disorders are a heterogeneous phenotypic group of illnesses arising from germline mutations that affect telomere maintenance (21). The first telomere biology disorder (TBD) was described as dyskeratosis congenita (DC), a hereditary disorder seen in childhood, in which ~20% of DC patients develop pulmonary fibrosis in later life (21). DC-linked mutations in telomere genes include those of DKC1, TINF2, NOP10, NHP2, TCAB1, and CTC1 (6). IPF is the most common manifestation of other adult TBDs (21). It is not surprising that more than one TBD or clinical presentation can be observed in the same patient or family, but usually, one disease is predominant (6, 21, 22). This might be caused by germline telomere gene mutations that can affect the homeostasis of multiple organ systems.
To date, mutations in many telomere-related genes have been found to be associated with IPF (Table 1) (23–43). Other telomere-related chronic lung diseases or phenotypes include chronic obstructive pulmonary disease (COPD), emphysema, chronic hypersensitivity pneumonitis, and rheumatoid arthritis-associated interstitial lung disease (44–48). It has been found that IPF and emphysema co-exist in the same pedigree, and IPF and COPD or lung cancer can co-occur in the same patient (39, 46, 49, 50).
Accumulating evidence has implicated telomere dysfunction in the pathogenesis of IPF. However, the underlying molecular mechanisms remain unclear. Available data suggest that cellular senescence or death of alveolar stem cells induced by telomere dysfunction is implicated in pulmonary fibrosis by altering cell signaling and the microenvironment in the lung.
Association Between Ipf And Telomere Abnormalities
It has been noticed that some IPF cases seem to be inherited, which was inferred from the observation of familial clustering of the disease and twin studies. Most IPF families display an autosomal dominant inheritance pattern with incomplete penetrance, suggesting that a single gene is responsible for disease pathogenesis in each family. A breakthrough discovery using linkage analysis identified TERT mutations in two IPF families (23). Subsequent sequence screening revealed that 15% of IPF families had TERT or TERC mutations, whereas 2% of sporadic IPF cases possessed TERT or TERC mutations. Patients with these heterozygous mutations in TERT or TERC had significantly shorter telomeres and decreased telomerase activity. A similar study screened 73 probands from the Vanderbilt Familial Pulmonary Fibrosis Registry and found that six (8%) had heterozygous mutations in TERT or TERC (24). In a study of familial IPF with early-onset, it was found that some cases with mutations in TERT and markedly short telomeres present with extrapulmonary features, including liver cirrhosis, bone marrow hypoplasia, and premature graying (51–53). Intriguingly, overall, as many as 37% of familial IPF and 25% of sporadic IPF cases have shorter telomeres, whereas as many as 24% of familial IPF and 23% of sporadic IPF cases without TERT or TERC mutations also have significantly short telomeres, suggesting the existence of other gene mutations in IPF (25, 26). Indeed, many telomere gene mutations have been associated with IPF (Table 1) (23–43). Together, these studies identified telomere dysfunction as a major driver of IPF.
Alveolar Stem-Cell Failure Induced By Telomere Dysfunction
Genetic studies on IPF have indicated that ~40% of familial IPF and 25% of sporadic IPF cases have significant telomere shortening and that 15% of familial IPF is due to TERT or TERC mutations. This fact suggests the importance of telomere dysfunction in IPF pathogenesis, but the question of how telomere dysfunction drives the genesis of pulmonary fibrosis remains. The capacity to regenerate lung tissue was determined to be significantly decreased in generation (G) 4 TERC KO mice after partial pneumonectomy, indicating that adult lung stem cells are significantly affected by telomere shortening (54). Moreover, in these G4 TERC KO mice, the number of AEC2s was significantly decreased and the apoptotic pathway was activated in the lungs, suggesting that AEC2s are vulnerable to apoptosis during telomere shortening (55). Interestingly, we observed that AEC2s in G3 TERC KO mice present with significant cellular senescence (56, 57). In addition, we found that G2 TERC KO mice display aggravated bleomycin (BLM)-induced pulmonary fibrosis, suggesting that telomere shortening promotes the genesis of pulmonary fibrosis (57). In line with this observation, late-generation TERT KO mice develop increased pulmonary fibrosis under low-dose BLM induction (58). Furthermore, spontaneous pulmonary fibrosis was found to occur after conditionally deleting the telomere shelterin component telomeric repeat binding-factor (TRF1) in AEC2s (58, 59). In TRF1 conditional KO fibrotic lungs, the loss of AEC2 (~2/3), and increased lung cellular senescence and apoptosis were observed. Thus, these findings suggest the important role of alveolar stem cell AEC2s in the pathogenesis of pulmonary fibrosis (58–60). However, pulmonary fibrosis has not been observed in a mouse model of TRF1 deletion in fibroblasts, suggesting that telomere dysfunction leading to pulmonary fibrosis might be dependent on AEC2s (59). Interestingly, in conditional TRF1 KO mice, the telomere lengths of AEC2s are normal at the early stage, whereas marked telomere shortening was observed at the late stage, suggesting that the long-term deletion of TRF1 might lead to telomere shortening within AEC2s (59). Similarly, AEC2-conditional TRF2 KO mice exhibit significant telomere dysfunction and cellular senescence (61). Moreover, a recent study suggested that TPP1 degradation leads to telomere uncapping in AEC2s, thereby promoting stress-induced cellular senescence and pulmonary fibrosis (62). An investigation of IPF with or without TERT mutations indicated that AEC2s, but not other surrounding cells, have significantly short telomeres (63). These studies imply that telomere dysfunction might preferably affect lung AEC2s and compromise these cells to generate spontaneous pulmonary fibrosis.
The animal models discussed above suggest that AEC2 failure plays a vital role in the development of pulmonary fibrosis. However, it is unclear how dysfunctional AEC2s cause the fibrotic process. One possibility might be due to defective AEC2 signaling other cells (61). Recently, a transgenic animal model study suggested that the cellular senescence, but not apoptosis, of AEC2s promotes the development of pulmonary fibrosis (19). A recent interesting discovery from a mouse model showed that conditionally deleted Cdc42 in AEC2s leads to progressive pulmonary fibrosis in mice, as seen in IPF patients (64). This study demonstrated that the deletion of Cdc42 results in the impaired differentiation ability of AEC2s to AEC1s, which suppresses new alveoli formation, in turn increasing mechanical tension in the lungs with a non-uniform distribution (64). Given the deficiency in the stem cell functions of AEC2s observed in late-generation TERC KO mice and conditional TRF2-deleted mice, it is possible that telomere dysfunction might be associated with this mechanical tension mechanism to promote pulmonary fibrosis caused by the inability to form new alveoli.
The Senescence-Associated Secretory Phenotype And Innate Immune Cell Infiltration Induce A Pro-Fibrotic Niche In The Lung
Senescent cells are metabolically active and produce a distinct SASP with various cytokines, chemokines, growth factors, and matrix metalloproteinases (10–13). Transforming growth factor-beta (TGF-β) secreted from senescent cells was found to cause senescence in neighboring cells, which might be important for organ aging, particularly in slowly self-renewing tissues such as the lung, because AEC2 cellular senescence might spread to other cells within the same organ (13). In an animal model, the deletion of TRF1 in AEC2s was found to result in the senescence of AEC2s and elevated levels of lung TGF-β1 (59). Similarly, lungs from late-generation (G2 and G3) TERC KO mice show significantly increased TGF-β1 and activated TGF-β/Smad signaling (57). Consistently, single-cell RNA-sequencing analysis revealed that IPF lungs harbor senescent AEC2s with increased TGF-β1 in these cells (65). In addition, microarray analysis of AEC2 TRF2-deficient mice revealed that the upregulated genes are involved in immune signaling and inflammation pathways (61). Moreover, in the late generation of both TERC and TERT KO mouse lungs, remarkably elevated levels of various cytokines, including IL-1, IL-6, CXCL15, IL-10, TNF-α, and CCL2, have been noted (56, 66).
It is widely observed that the lungs from transgenic mice with telomere dysfunction show innate immune cell infiltration, accompanied by lung inflammation. Late-generation TERC-deficient mouse lungs have increased CD11b+, CD16/CD32+, or CD45+ cells, macrophages, neutrophils, and natural killer (NK) cells (56, 57). TRF1-deficient mice show extensive macrophage infiltration in the lungs, intense pneumonia, and increased numbers of mononuclear cells, neutrophils, and lymphocytes in bronchoalveolar lavage (BAL) fluids (58, 59). Similarly, in conditional TRF2 KO mice, the BAL fluids contain a significantly increased number of macrophages and lymphocytes and the lungs exhibit macrophage inflammatory infiltrates and peribronchiolar and perivascular inflammation (61). These data suggest that telomere dysfunction promotes significant infiltration or the recruitment of innate immune cells in the lungs. In addition to its role in the phagocytosis of senescent and apoptotic cells, macrophages play a promoting role in the development of pulmonary fibrosis (67). Several profibrotic genes such as arginase1 and matrix metallopeptidase 13 (MMP13) were found to be upregulated in monocyte-derived macrophages in experimental pulmonary fibrosis, as well as in resident alveolar macrophages in IPF patients (68). Moreover, M2 macrophages are an important regulator of pulmonary fibrosis, partially through the secretion of profibrotic interleukin-10 (IL-10) and TGF-β (67). Whether and how the recruitment of macrophages in the lungs caused by telomere dysfunction participates in pulmonary fibrosis development requires further investigation (69).
In summary, we propose a model to demonstrate how telomere dysfunction drives the pathogenesis of IPF (Figure 2). Telomere-related mutations or telomere shortening preferentially affect alveolar stem cell AEC2s in the lung and cause senescence or apoptosis. This cellular dysfunction is sufficient to generate spontaneous pulmonary fibrosis, mainly through two pathways. First, cellular senescence and/or cell death of AEC2s leads to a pro-fibrotic niche through the SASP, and fibrocytes capable of differentiating into fibroblasts, myofibroblasts, and innate immune cells are recruited to the fibrotic lesion site. Second, the failure of AEC2s compromises the regeneration of new alveoli, which in turn leads to an increase in mechanical tension in a periphery-to-center spatial distribution, which activates the TGF-β signaling loop in AEC2s or other lung cells. These two pathways might significantly increase the local levels of TGF-β, myofibroblast differentiation, and fibrotic lesions in the lung tissue.
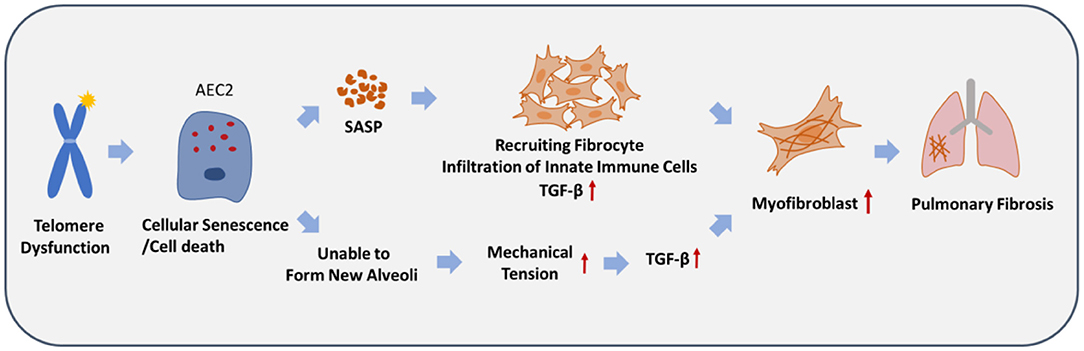
Figure 2. A proposed model to depict the pathogenesis of idiopathic pulmonary fibrosis caused by telomere dysfunction. Telomere dysfunction causes cellular senescence and/or cell death of alveolar epithelial type 2 cells (AEC2s), which leads to the production of a pro-fibrotic niche, mediated by the SASP. This probably leads to increased mechanical tension and a TGF-β signaling loop in a spatially distributed manner in the lung, owing to the inability to form new alveoli. Eventually, pulmonary fibrosis develops due to the significant increase in myofibroblasts.
Discussion
The available data from human IPF cases and animal model studies have established a connection between telomere dysfunction and pulmonary fibrosis mediated by alveolar stem cell AEC2s. However, it is still unclear how these cells promote the development of pulmonary fibrosis. Although some animal model studies support the idea that cellular senescence of AEC2s drives the development of pulmonary fibrosis (19, 59, 61), other animal studies suggest that AEC2 cell death plays an important role in the development of pulmonary fibrosis (58). It is likely that senescent AEC2s signal other cells through the SASP (61). Apoptotic, necrotic, necroptotic, and pyroptotic cells can also release diverse cytokines; specifically, apoptotic cells secrete IL-10 and TGF-β, necrotic cells release IL-1α, and necroptotic cells release IL-8, IL-1, chemokine ligand 2 (CXCL2), and cyclophilin A, whereas pyroptotic cells secrete IL-1β, IL-18, tumor necrosis factor-alpha (TNF-α), and IL-6 (70). The secretion of these factors is thought to lead to the recruitment of innate immune cells to remove these dying cells to regulate inflammation and wound healing, but the release of excessive amounts of IL-10 and TGF-β might contribute to pulmonary fibrosis. Thus, the contribution of cellular senescence and cell death of AEC2s to the pathogenesis of pulmonary fibrosis requires further investigation.
Although it is now well-recognized that telomere dysfunction preferably affects lung AEC2 function and is a major driver of pulmonary fibrosis, other stem cells such as club cells and basal cells might also be affected by telomere dysfunction, thereby exerting their respective effects. Notably, 40% of familial IPF and 25% of sporadic IPF cases have significantly short telomeres, in which genetic alterations in telomere-related genes have been identified, suggesting the important role of telomere dysfunction in the pathogenesis of pulmonary fibrosis. Furthermore, it has been reported that some telomere-unrelated genes are also implicated in the genesis of pulmonary fibrosis, suggesting complex mechanisms of IPF at different pathophysiological levels (71, 72).
It is worth mentioning that IPF cases are usually seen in old age, and some relatives of IPF patients with both TERT or TERC mutations and telomere shortening do not develop pulmonary fibrosis, suggesting that aging and environmental factors are also involved in disease pathogenesis (25). The aging-associated changes in telomere length, stem cell senescence, autophagy, endoplasmic reticulum stress responses, immunosenescence, and epigenetics, as well as cumulative environmental exposure, might contribute to disease development through an aging mechanism (73, 74). In addition, viral infection, cigarette smoking, and occupational exposure could also confer a risk of IPF (73, 75).
Clinical outcomes and therapeutic responses might differ based on different genotypes in IPF; further understanding this difference will allow for the development of personalized approaches to disease diagnosis, management, and treatment (76, 77). Patients with remarkably short telomeres have more rapid disease progression, a shorter lung transplant-free survival time, and a poor prognosis (78). It has been found that IPF patients with mutations in TERT, PARN, TERC, or RTEL1 have shorter telomeres and earlier disease onset than patients without, and IPF cases with TERC mutations are diagnosed at an earlier age than those with PARN mutations (30). Moreover, it has been found that IPF with mutations in TERT, RTEL1, or PARN has a significantly higher risk of death and chronic lung allograft dysfunction compared to those in patients without these mutations (79, 80). Advances in next-generation sequencing and gene-chip technologies would facilitate the development of standard methods and criteria for the precise diagnosis of IPF. We anticipate that CRISPR/Cas9 genomic editing, stem cell therapy, telomerase treatment, or targeting cellular senescence will hold promise for the future treatment of this fatal disease.
Author Contributions
KZ wrote the first draft of the manuscript. LX and KZ prepared the tables and figures. All authors critically revised the manuscript, reviewed the final version, agreed upon submission, and agree to be accountable for the content of the work.
Funding
This work was supported by grants from the National Natural Science Foundation of China (31730020), Hangzhou Science and Technology Bureau (20182014B01), and Hangzhou Human Resources and Social Security Bureau (4125F5061700502).
Conflict of Interest
The authors declare that the research was conducted in the absence of any commercial or financial relationships that could be construed as a potential conflict of interest.
Publisher's Note
All claims expressed in this article are solely those of the authors and do not necessarily represent those of their affiliated organizations, or those of the publisher, the editors and the reviewers. Any product that may be evaluated in this article, or claim that may be made by its manufacturer, is not guaranteed or endorsed by the publisher.
References
1. King TE Jr, Pardo A, Selman M. Idiopathic pulmonary fibrosis. Lancet. (2011) 378:1949–61. doi: 10.1016/S0140-6736(11)60052-4
2. Fernandez IE, Eickelberg O. New cellular and molecular mechanisms of lung injury and fibrosis in idiopathic pulmonary fibrosis. Lancet. (2012) 380:680–8. doi: 10.1016/S0140-6736(12)61144-1
3. du Bois RM. Idiopathic pulmonary fibrosis: present understanding and future options. Eur Respir Rev. (2011) 20:132–3. doi: 10.1183/09059180.00001511
4. Martínez P, Blasco MA. Replicating through telomeres: a means to an end. Trends Biochem Sci. (2015) 40:504–15. doi: 10.1016/j.tibs.2015.06.003
5. Smith EM, Pendlebury DF, Nandakumar J. Structural biology of telomeres and telomerase. Cell Mol Life Sci. (2020) 77:61–79. doi: 10.1007/s00018-019-03369-x
6. Armanios M, Blackburn EH. The telomere syndromes, Nat Rev Genet (2012) 13:693–704. doi: 10.1038/nrg3246
7. Moon DH, Segal M, Boyraz B, Guinan E, Hofmann I, Cahan P, et al. Poly(A)-specific ribonuclease (PARN) mediates 3′-end maturation of the telomerase RNA component. Nat Genet. (2015) 47:1482–8. doi: 10.1038/ng.3423
8. Grill S, Nandakumar J. Molecular mechanisms of telomere biology disorders. J Biol Chem. (2020) 296:100064. doi: 10.1074/jbc.REV120.014017
9. Sperka T, Song Z, Morita Y, Nalapareddy K, Guachalla LM, Lechel A, et al. Puma and p21 represent cooperating checkpoints limiting self-renewal and chromosomal instability of somatic stem cells in response to telomere dysfunction. Nat Cell Biol. (2011) 14:73–9. doi: 10.1038/ncb2388
10. Pawlikowski JS, Adams PD, Nelson DM. Senescence at a glance. J Cell Sci. (2013) 126:4061–7. doi: 10.1242/jcs.109728
11. Hubackova S, Krejcikova K, Bartek J, Hodny Z. IL1- and TGFβ-Nox4 signaling, oxidative stress and DNA damage response are shared features of replicative, oncogene-induced, and drug-induced paracrine 'bystander senescence'. Aging. (2012) 4:932–51. doi: 10.18632/aging.100520
12. Freund A, Orjalo AV, Desprez PY, Campisi J. Inflammatory networks during cellular senescence: causes and consequences. Trends Mol Med. (2010) 16:238–46. doi: 10.1016/j.molmed.2010.03.003
13. Acosta JC, Banito A, Wuestefeld T, Georgilis A, Janich P, Morton JP, et al. A complex secretory program orchestrated by the inflammasome controls paracrine senescence. Nat Cell Biol. (2013) 15:978–90. doi: 10.1038/ncb2784
14. Banito A, Lowe SW. A new development in senescence. Cell. (2013) 155:977–8. doi: 10.1016/j.cell.2013.10.050
15. Zhang K, Chen C, Liu Y, Chen H, Liu JP. Cellular senescence occurred widespread to multiple selective sites in the fetal tissues and organs of mice. Clin Exp Pharmacol Physiol. (2014) 41:965–75. doi: 10.1111/1440-1681.12328
16. Childs BG, Durik M, Baker DJ, van Deursen JM. Cellular senescence in aging and age-related disease: from mechanisms to therapy. Nat Med. (2015) 21:1424–35. doi: 10.1038/nm.4000
17. Rodier F, Campisi J. Four faces of cellular senescence. J Cell Biol. (2011) 192:547–56. doi: 10.1083/jcb.201009094
18. Parimon T, Yao C, Stripp BR, Noble PW, Chen P. Alveolar epithelial type II cells as drivers of lung fibrosis in idiopathic pulmonary fibrosis. Int J Mol Sci. (2020) 21:2269. doi: 10.3390/ijms21072269
19. Yao C, Guan X, Carraro G, Parimon T, Liu X, Huang G, et al. Senescence of alveolar type 2 cells drives progressive pulmonary fibrosis. Am J Respir Crit Care Med. (2021) 203:707–17. doi: 10.1164/rccm.202004-1274OC
20. Ruaro B, Salton F, Braga L, Wade B, Confalonieri P, Volpe MC, et al. The history and mystery of alveolar epithelial type II cells: focus on their physiologic and pathologic role in lung. Int J Mol Sci. (2021) 22:2566. doi: 10.3390/ijms22052566
21. Kam MLW, Nguyen TTT, Ngeow JYY. Telomere biology disorders. NPJ Genom Med. (2021) 6:36. doi: 10.1038/s41525-021-00198-5
22. Bertuch AA. The molecular genetics of the telomere biology disorders. RNA Biol. (2016) 13:696–706. doi: 10.1080/15476286.2015.1094596
23. Tsakiri KD, Cronkhite JT, Kuan PJ, Xing C, Raghu G, Weissler JC, et al. Adult-onset pulmonary fibrosis caused by mutations in telomerase. Proc Natl Acad Sci USA. (2007) 104:7552–7. doi: 10.1073/pnas.0701009104
24. Armanios MY, Chen JJ, Cogan JD, Alder JK, Ingersoll RG, Markin C, et al. Telomerase mutations in families with idiopathic pulmonary fibrosis. N Engl J Med. (2007) 356:1317–26. doi: 10.1056/NEJMoa066157
25. Cronkhite JT, Xing C, Raghu G, Chin KM, Torres F, Rosenblatt RL, et al. Telomere shortening in familial and sporadic pulmonary fibrosis. Am J Respir Crit Care Med. (2008) 178:729–37. doi: 10.1164/rccm.200804-550OC
26. Alder JK, Chen JJ, Lancaster L, Danoff S, Su SC, Cogan JD, et al. Short telomeres are a risk factor for idiopathic pulmonary fibrosis. Proc Natl Acad Sci USA. (2008) 105:13051–6. doi: 10.1073/pnas.0804280105
27. Gansner JM, Rosas IO, Ebert BL. Pulmonary fibrosis, bone marrow failure, and telomerase mutation. N Engl J Med. (2012) 366:1551–3. doi: 10.1056/NEJMc1200999
28. Gutierrez-Rodrigues F, Donaires FS, Pinto A, Vicente A, Dillon LW, Clé DV, et al. Pathogenic TERT promoter variants in telomere diseases. Genet Med. (2019) 21:1594–602. doi: 10.1038/s41436-018-0385-x
29. Petrovski S, Todd JL, Durheim MT, Wang Q, Chien JW, Kelly FL, et al. An exome sequencing study to assess the role of rare genetic variation in pulmonary fibrosis. Am J Respir Crit Care Med. (2017) 196:82–93. doi: 10.1164/rccm.201610-2088OC
30. Dressen A, Abbas AR, Cabanski C, Reeder J, Ramalingam TR, Neighbors M, et al. Analysis of protein-altering variants in telomerase genes and their association with MUC5B common variant status in patients with idiopathic pulmonary fibrosis: a candidate gene sequencing study. Lancet Respir Med. (2018) 6:603–14. doi: 10.1016/S2213-2600(18)30135-8
31. Kropski JA, Mitchell DB, Markin C, Polosukhin VV, Choi L, Johnson JE, et al. A novel dyskerin (DKC1) mutation is associated with familial interstitial pneumonia. Chest. (2014) 146:e1–7. doi: 10.1378/chest.13-2224
32. Gaysinskaya V, Stanley SE, Adam S, Armanios M. Synonymous mutation in DKC1 causes telomerase RNA insufficiency manifesting as familial pulmonary fibrosis. Chest. (2020) 158:2449–57. doi: 10.1016/j.chest.2020.07.025
33. Alder JK, Stanley SE, Wagner CL, Hamilton M, Hanumanthu VS, Armanios M. Exome sequencing identifies mutant TINF2 in a family with pulmonary fibrosis. Chest. (2015) 147:1361–8. doi: 10.1378/chest.14-1947
34. Hoffman TW, van der Vis JJ, van Oosterhout MF, van Es HW, van Kessel DA, Grutters JC, et al. TINF2 gene mutation in a patient with pulmonary fibrosis. Case Rep Pulmonol. (2016) 2016:1310862. doi: 10.1155/2016/1310862
35. Kannengiesser C, Borie R, Ménard C, Réocreux M, Nitschké P, Gazal S, et al. Heterozygous RTEL1 mutations are associated with familial pulmonary fibrosis. Eur Respir J. (2015) 46:474–85. doi: 10.1183/09031936.00040115
36. Stuart BD, Choi J, Zaidi S, Xing C, Holohan B, Chen R, et al. Exome sequencing links mutations in PARN and RTEL1 with familial pulmonary fibrosis and telomere shortening. Nat Genet. (2015) 47:512–7. doi: 10.1038/ng.3278
37. Zhang D, Zhou Z, Abu-Hijleh M, Batra K, Xing C, Garcia CK. Homozygous rare PARN missense mutation in familial pulmonary fibrosis. Am J Respir Crit Care Med. (2019) 199:797–9. doi: 10.1164/rccm.201809-1632LE
38. Kropski JA, Reiss S, Markin C, Brown KK, Schwartz DA, Schwarz MI, et al. Rare genetic variants in PARN are associated with pulmonary fibrosis in families. Am J Respir Crit Care Med. (2017) 196:1481–4. doi: 10.1164/rccm.201703-0635LE
39. Stanley SE, Gable DL, Wagner CL, Carlile TM, Hanumanthu VS, Podlevsky JD, et al. Loss-of-function mutations in the RNA biogenesis factor NAF1 predispose to pulmonary fibrosis-emphysema. Sci Transl Med. (2016) 8:351ra107. doi: 10.1126/scitranslmed.aaf7837
40. Kannengiesser C, Manali ED, Revy P, Callebaut I, Ba I, Borgel A, et al. First heterozygous NOP10 mutation in familial pulmonary fibrosis. Eur Respir J. (2020) 55:1902465. doi: 10.1183/13993003.02465-2019
41. Benyelles M, O'Donohue MF, Kermasson L, Lainey E, Borie R, Lagresle-Peyrou C, et al. NHP2 deficiency impairs rRNA biogenesis and causes pulmonary fibrosis and Høyeraal-Hreidarsson syndrome. Hum Mol Genet. (2020) 29:907–22. doi: 10.1093/hmg/ddaa011
42. Hoffman TW, van der Vis JJ, van der Smagt JJ, Massink MPG, Grutters JC, van Moorsel CHM. Pulmonary fibrosis linked to variants in the ACD gene, encoding the telomere protein TPP1. Eur Respir J. (2019) 54:1900809. doi: 10.1183/13993003.00809-2019
43. Gable DL, Gaysinskaya V, Atik CC, Talbot CC Jr, Kang B, Stanley SE, et al. ZCCHC8, the nuclear exosome targeting component, is mutated in familial pulmonary fibrosis and is required for telomerase RNA maturation. Genes Dev. (2019) 33:1381–96. doi: 10.1101/gad.326785.119
44. Morlá M, Busquets X, Pons J, Sauleda J, MacNee W, Agustí AG. Telomere shortening in smokers with and without COPD. Eur Respir J. (2006) 27:525–8. doi: 10.1183/09031936.06.00087005
45. Alder JK, Guo N, Kembou F, Parry EM, Anderson CJ, Gorgy AI, et al. Telomere length is a determinant of emphysema susceptibility. Am J Respir Crit Care Med. (2011) 184:904–12. doi: 10.1164/rccm.201103-0520OC
46. Stanley SE, Merck SJ, Armanios M. Telomerase and the genetics of emphysema susceptibility. implications for pathogenesis paradigms and patient care. Ann Am Thorac Soc. (2016) 13:S447–51. doi: 10.1513/AnnalsATS.201609-718AW
47. Newton CA, Oldham JM, Ley B, Anand V, Adegunsoye A, Liu G, et al. Telomere length and genetic variant associations with interstitial lung disease progression and survival. Eur Respir J. (2019 53:1801641. doi: 10.1183/13993003.01641-2018
48. Ley B, Newton CA, Arnould I, Elicker BM, Henry TS, Vittinghoff E, et al. The MUC5B promoter polymorphism and telomere length in patients with chronic hypersensitivity pneumonitis: an observational cohort-control study. Lancet Respir Med. (2017) 5:639–47. doi: 10.1016/S2213-2600(17)30216-3
49. Gauldie J, Kolb M, Ask K, Martin G, Bonniaud P, Warburton D. Smad3 signaling involved in pulmonary fibrosis and emphysema. Proc Am Thorac Soc. (2006) 3:696–702. doi: 10.1513/pats.200605-125SF
50. Saito A, Horie M, Micke P, Nagase T. The role of TGF-β signaling in lung cancer associated with idiopathic pulmonary fibrosis. Int J Mol Sci. (2018) 19:3611. doi: 10.3390/ijms19113611
51. Fernandez BA, Fox G, Bhatia R, Sala E, Noble B, Denic N, et al. A Newfoundland cohort of familial and sporadic idiopathic pulmonary fibrosis patients: clinical and genetic features. Respir Res. (2012) 13:64. doi: 10.1186/1465-9921-13-64
52. Diaz de Leon A, Cronkhite JT, Yilmaz C, Brewington C, Wang R, Xing C, et al. Subclinical lung disease, macrocytosis, and premature graying in kindreds with telomerase (TERT) mutations. Chest. (2011) 140:753–63. doi: 10.1378/chest.10-2865
53. Molina-Molina M, Borie R. Clinical implications of telomere dysfunction in lung fibrosis. Curr Opin Pulm Med. (2018) 24:440–4. doi: 10.1097/MCP.0000000000000506
54. Jackson SR, Lee J, Reddy R, Williams GN, Kikuchi A, Freiberg Y, et al. Partial pneumonectomy of telomerase null mice carrying shortened telomeres initiates cell growth arrest resulting in a limited compensatory growth response. Am J Physiol Lung Cell Mol Physiol. (2011) 300:L898–909. doi: 10.1152/ajplung.00409.2010
55. Lee J, Reddy R, Barsky L, Scholes J, Chen H, Shi W, et al. Lung alveolar integrity is compromised by telomere shortening in telomerase-null mice. Am J Physiol Lung Cell Mol Physiol. (2009) 296:L57–70. doi: 10.1152/ajplung.90411.2008
56. Chen R, Zhang K, Chen H, Zhao X, Wang J, Li L, et al. Telomerase deficiency causes alveolar stem cell senescence-associated low-grade inflammation in lungs. J Biol Chem. (2015) 290:30813–29. doi: 10.1074/jbc.M115.681619
57. Liu YY, Shi Y, Liu Y, Pan XH, Zhang KX. Telomere shortening activates TGF-β/Smads signaling in lungs and enhances both lipopolysaccharide and bleomycin-induced pulmonary fibrosis. Acta Pharmacol Sin. (2018) 39:1735–45. doi: 10.1038/s41401-018-0007-9
58. Povedano JM, Martinez P, Flores JM, Mulero F, Blasco MA. Mice with pulmonary fibrosis driven by telomere dysfunction. Cell Rep. (2015) 12:286–99. doi: 10.1016/j.celrep.2015.06.028
59. Naikawadi RP, Disayabutr S, Mallavia B, Donne ML, Green G, La JL, et al. Telomere dysfunction in alveolar epithelial cells causes lung remodeling and fibrosis. JCI Insight. (2016) 1:e86704. doi: 10.1172/jci.insight.86704
60. Barkauskas CE, Cronce MJ, Rackley CR, Bowie EJ, Keene DR, Stripp BR, et al. Type 2 alveolar cells are stem cells in adult lung. J Clin Invest. (2013) 123:3025–36. doi: 10.1172/JCI68782
61. Alder JK, Barkauskas CE, Limjunyawong N, Stanley SE, Kembou F, Tuder RM, et al. Telomere dysfunction causes alveolar stem cell failure. Proc Natl Acad Sci USA. (2015) 112:5099–104. doi: 10.1073/pnas.1504780112
62. Wang L, Chen R, Li G, Wang Z, Liu J, Liang Y, et al. FBW7 mediates senescence and pulmonary fibrosis through telomere uncapping. Cell Metab. (2020) 32:860–77.e9. doi: 10.1016/j.cmet.2020.10.004
63. Snetselaar R, van Batenburg AA, van Oosterhout MFM, Kazemier KM, Roothaan SM, Peeters T, et al. Short telomere length in IPF lung associates with fibrotic lesions and predicts survival. PLoS One. (2017) 12:e0189467. doi: 10.1371/journal.pone.0189467
64. Wu H, Yu Y, Huang H, Hu Y, Fu S, Wang Z, et al. Progressive pulmonary fibrosis is caused by elevated mechanical tension on alveolar stem cells. Cell. (2020) 180:107–21.e17. doi: 10.1016/j.cell.2019.11.027
65. Xu Y, Mizuno T, Sridharan A, Du Y, Guo M, Tang J, et al. Single-cell RNA sequencing identifies diverse roles of epithelial cells in idiopathic pulmonary fibrosis. JCI Insight. (2016) 1:e90558. doi: 10.1172/jci.insight.90558
66. Amsellem V, Gary-Bobo G, Marcos E, Maitre B, Chaar V, Validire P, et al. Telomere dysfunction causes sustained inflammation in chronic obstructive pulmonary disease. Am J Respir Crit Care Med. (2011) 184:1358–66. doi: 10.1164/rccm.201105-0802OC
67. Cheng P, Li S, Chen H. Macrophages in lung injury, repair, and fibrosis. Cells. (2021) 10:436. doi: 10.3390/cells10020436
68. Misharin AV, Morales-Nebreda L, Reyfman PA, Cuda CM, Walter JM, McQuattie-Pimentel AC, et al. Monocyte-derived alveolar macrophages drive lung fibrosis and persist in the lung over the life span. J Exp Med. (2017) 214:2387–404. doi: 10.1084/jem.20162152
69. Trombetta AC, Soldano S, Contini P, Tomatis V, Ruaro B, Paolino S, et al. A circulating cell population showing both M1 and M2 monocyte/macrophage surface markers characterizes systemic sclerosis patients with lung involvement. Respir Res. (2018) 19:186. doi: 10.1186/s12931-018-0891-z
70. Atkin-Smith GK. Phagocytic clearance of apoptotic, necrotic, necroptotic and pyroptotic cells. Biochem Soc Trans. (2021) 49:793–804. doi: 10.1042/BST20200696
71. Garcia CK. Idiopathic pulmonary fibrosis: update on genetic discoveries. Proc Am Thorac Soc. (2011) 8:158–62. doi: 10.1513/pats.201008-056MS
72. Michalski JE, Schwartz DA. Genetic risk factors for idiopathic pulmonary fibrosis: insights into immunopathogenesis. J Inflamm Res. (2021) 13:1305–18. doi: 10.2147/JIR.S280958
73. Naik PK, Moore BB. Viral infection and aging as cofactors for the development of pulmonary fibrosis. Expert Rev Respir Med. (2010) 4:759–71. doi: 10.1586/ers.10.73
74. López-Otín C, Blasco MA, Partridge L, Serrano M, Kroemer G. The hallmarks of aging. Cell. (2013) 153:1194–217. doi: 10.1016/j.cell.2013.05.039
75. Yang IV. Epigenomics of idiopathic pulmonary fibrosis. Epigenomics. (2012) 4:195–203. doi: 10.2217/epi.12.10
76. Kaur A, Mathai SK, Schwartz DA. Genetics in idiopathic pulmonary fibrosis pathogenesis, prognosis, and treatment. Front Med. (2017) 4:154. doi: 10.3389/fmed.2017.00154
77. Baratella E, Ruaro B, Giudici F, Wade B, Santagiuliana M, Salton F, et al. Evaluation of correlations between genetic variants and high-resolution computed tomography patterns in idiopathic pulmonary fibrosis. Diagnostics. (2021) 11:762. doi: 10.3390/diagnostics11050762
78. Courtwright AM, El-Chemaly S. Telomeres in interstitial lung disease: the short and the long of it. Ann Am Thorac Soc. (2019) 16:175–81. doi: 10.1513/AnnalsATS.201808-508CME
79. Moore C, Blumhagen RZ, Yang IV, Walts A, Powers J, Walker T, et al. Resequencing study confirms that host defense and cell senescence gene variants contribute to the risk of idiopathic pulmonary fibrosis. Am J Respir Crit Care Med. (2019) 200:199–208. doi: 10.1164/rccm.201810-1891OC
Keywords: telomere dysfunction, telomere shortening, alveolar stem cells, SASP, innate immune cells, TGF-β
Citation: Zhang K, Xu L and Cong Y-S (2021) Telomere Dysfunction in Idiopathic Pulmonary Fibrosis. Front. Med. 8:739810. doi: 10.3389/fmed.2021.739810
Received: 12 July 2021; Accepted: 05 October 2021;
Published: 11 November 2021.
Edited by:
Elizabeth Cordoba-Lanus, University of La Laguna, SpainReviewed by:
Helen Parfrey, Royal Papworth Hospital NHS Foundation Trust, United KingdomBarbara Ruaro, University of Trieste, Italy
Amiq Gazdhar, Bern University Hospital, Switzerland
Copyright © 2021 Zhang, Xu and Cong. This is an open-access article distributed under the terms of the Creative Commons Attribution License (CC BY). The use, distribution or reproduction in other forums is permitted, provided the original author(s) and the copyright owner(s) are credited and that the original publication in this journal is cited, in accordance with accepted academic practice. No use, distribution or reproduction is permitted which does not comply with these terms.
*Correspondence: Kexiong Zhang, a3h6aGFuZ0Boem51LmVkdS5jbg==; Yu-Sheng Cong, eXNjb25nQGh6bnUuZWR1LmNu