- 1Inserm, UMR1098, Federation Hospitalo-Universitaire INCREASE, Besançon, France
- 2University Bourgogne Franche-Comté, Faculté de Médecine et de Pharmacie, LabEx LipSTIC, Besançon, France
- 3Structure Fédérative de Recherche, SFR FED4234, Besançon, France
- 4CHU Besançon, Department of Nephrology, Dialysis, and Renal Transplantation, Besançon, France
- 5EFS Bourgogne Franche-Comté, Plateforme de Biomonitoring, CIC 1431/UMR1098, Besançon, France
End-stage renal disease (ESRD) patients exhibit clinical features of premature ageing, including frailty, cardiovascular disease, and muscle wasting. Accelerated ageing also concerns the immune system. Patients with ESRD have both immune senescence and chronic inflammation that are resumed in the so-called inflammaging syndrome. Immune senescence is particularly characterised by premature loss of thymic function that is associated with hyporesponsiveness to vaccines, susceptibility to infections, and death. ESRD-related chronic inflammation has multiple causes and participates to accelerated cardiovascular disease. Although, both characterisation of immune senescence and its consequences are relatively well-known, mechanisms are more uncertain. However, prevention of immune senescence/inflammation or/and rejuvenation of the immune system are major goal to ameliorate clinical outcomes of ESRD patients.
Introduction
Patients with end-stage renal disease (ESRD) are especially prone to infection (1). Furthermore, concordant data also report that immune responses against vaccines are considerably reduced in this population (2). Concomitantly, ESRD patients exhibit aseptic low-grade inflammation (3, 4). These clinical features are close to those observed in elderly and suggest that inflammaging associating both premature senescence of the immune system and inflammation is a key part of the ESRD-related immune phenotype. What is more, some convincing studies established evidences of accelerated immune senescence in chronic kidney disease and dialysis patients compared to the general population (5–7).
In this review, we analyze recent knowledge on ESRD-associated accelerated immune ageing with a special focus on thymus involution. In addition, we speculate on therapeutic tools likely to prevent or reverse these immune alterations.
Immune Senescence in Ageing
The term immune senescence clusters all the changes that occur in the immune system during ageing. Although this process mainly affects T lymphocytes, all aspects of innate and adaptive immunity are concerned. Recently, immune ageing has been suggested to be more appropriate to design all immune changes associated with ageing. Indeed, the ageing of the immune system is a more general concept including two different processes. The first one is what specifically refers to immune senescence, which is mainly linked to age-dependent thymic involution leading to reduced immune repertoire diversity and compounded oligo-clonal increase in memory immune cells. Sensitivity to infections, reduced vaccine immunity, and defect in tumour clearance observed in elderly are thought to be at least in part linked to these immune alterations. Immune senescence in T cells is sometimes called cellular exhaustion even if the two phenomenon are not exactly identical. Exhausted T cells are defined by the loss of CD28 and the concomitant expression of Tim-3 and PD-1 (8). The second characteristics of aged immunity is inflammaging. Old age is associated with low-grade systemic inflammation. Chronic innate immune activation, pro-inflammatory cytokine profile secretion, and age-induced accumulation of self-reactive T cells contribute to age-related inflammation. Inflammaging is supposed to explain some degenerative disease associated with ageing. The term “Inflammaging” is frequently proposed to include these two aspects.
Immune Senescence: A Pivotal Role of Thymic Involution
T cell immune senescence is mainly linked to physiologic thymic involution. The thymus mainly serves to the development of a large but self-tolerant T cell repertoire. Briefly, multipotent hematopoietic stem cells (HSC) differentiate into common lymphoid or myeloid progenitors. T lymphoids precursors go to the thymus where they undergo several stages of maturation resulting in the formation of naive T lymphocytes called recent thymic emigrant (RTE) (9). These cells present a diversified polyclonal T cell receptor (TCR). Central tolerance occurs in the thymus via two mechanisms. The first one is thymocyte negative selection. This step consists in deletion of most of self-auto-reactive T cells via apoptosis (9). The second concerns the generation of CD4 single positive FoxP3+ regulatory T cells, which can eliminate auto-reactive T cells having escaped to negative selection (9).
The ability to generate RTE in the thymus declines with age. Thymic involution consists in reduction of both thymic size and thymocyte number and reorganisation of thymic ultrastructure. Soon after birth, functional tissue begins to be substituted by fat (10). After 50 years, there is almost no output of naïve T cells. The frequency of naïve T cells greatly diminishes both in periphery and in lymphoid organ, especially for CD8+ T cells. Nevertheless, homeostatic proliferation of previously generated naïve T cells enables to maintain a broad and diverse pool of naïve T cells, especially for CD4+ T cells. Continuous involution of the thymus with age finally causes a decrease in the thymic output of naïve T cells and subsequently a reduction of the peripheral TCR repertoire.
Two non-exclusive mechanisms account for thymus involution. The first one is mainly based on a reduced production of HSC. Indeed, self-renewal of HSC diminishes with age and tends to favour myeloid lineage (11, 12). Reduction in HSC production and switch toward myeloid lineage would both contribute to decrease the output of common lymphoid progenitors (13, 14). In addition, aged hematopoietic stem cells have less lymphoid differentiation potential (15). The second one depends on age-related reduction in stromal niches of the bone marrow () and thymus (16, 17). Recent studies mainly plead for the latter hypothesis. Stroma cells in the thymus are mainly thymic epithelial cells (TECs) (18). Convincing data show that age-associated thymic involution is dependent on TEC transcription factors involved in TEC homeostasis, such as Forkhead box N1 (19). Indeed, FOXN1 is essential for thymus development and thymocyte formation (19). A null mutation in the FOXN1 gene defines the “null mice” which phenotype is characterised, amongst others, by the absence of thymus and T cells (20). Reduction in thymic FOXN1 expression is observed as one the first step of thymic involution in aged individuals (21). Conditional KO mice studies have considerably explained the causal role of FOXN1 in thymus involution. LoxP-floxed-FoxN1 mouse with the ubiquitous CreER(T) transgene have a low dose of spontaneous activation and exhibit progressive loss of FOXN1 (22). Progressive loss of FOXN1 is associated with accelerated thymic involution (22). Finally, intra-thymic supplementation in FOXN1-cDNA partially reverses thymic involution and restores peripheral CD4+ T cell population (22).
The decrease in T cell production is compensated by homeostatic expansion of existing peripheral T cells occurs. This leads to an increased proportion of memory T cells and reduction in the diversity of TCR repertoire (23). Accumulation of memory T cells is mainly due to life-long exposure to chronic antigen stimulation by pathogens. The most important is cytomegalovirus. However, expansion of CD8+ T cells is only observed in CMV-exposed old patients (24).
Inflammaging
Somatic cellular senescence is defined by the permanent arrest of cell cycle accompanied by lack of proliferation, expression of anti-proliferative markers, and shortening of telomeres (25). This biological process is likely to be protective against cancer transformation (26).
Accumulation of somatic senescent cells contribute on one hand to organ dysfunction, and on the other hand to inflammation through induction of somatic cell senescence-associated secretory phenotype (SASP). Immune senescence favours increased production of SASP (27) due to decreased chemotaxis of immune cells toward somatic senescent cells and reduced phagocytosis by neutrophils and macrophages (28–31).
Many other mechanisms contribute to inflammation in ageing. Chronic viral infections, especially with CMV, induce low level of cytokines production (32).
Moreover, involution of the thymus is accompanied by a decrease ability to negatively select self-auto-reactive T cells, which explain propension to certain autoimmune diseases in the elderly (33). Paradoxically, peripheral Treg cells accumulate during ageing. Thomas et al. (34) developed a mock-self-antigen chimaera mouse model, in which membrane-bound ovalbumin transgenic mice, carrying a FOXN1-floxed gene for induction of conditional thymic atrophy, received ovalbumin-specific T cell receptor transgenic progenitor cells. The authors showed that a decreased number of ovalbumin-specific tTreg and pTreg, but not polyclonal Treg cells in chimeric mice with thymus atrophy. The ovalbumin-specific pTreg had less suppressive activity and a lower expression of FoxP3. This suggest that although generation of polyclonal pan-Treg is not affected by thymus involution, certain specific Treg clones may have aberrant agonist selection contributing to age-related chronic inflammation.
Thus, immune ageing is characterised by both immune deficiency (immune senescence driven by thymus involution) and inflammation leading to the concept of inflammaging.
Immune Ageing in End-Stage Renal Disease
Chronic kidney disease phenotype is very similar to premature ageing. Frailty, osteoporosis, muscle wasting, and cardiovascular disease occur at younger age in CKD patients. Many factors such as oxidative stress, accumulation of uremic toxins, and inflammation are supposed to contribute to accelerated ageing (35). The immune system undergoes a similar premature ageing. Indeed, peripheral blood mononuclear cell relative telomere length is shorter in CKD patients as compared to healthy individuals (5). Furthermore, ESRD patients frequently exhibit T cell lymphopenia (6) and concomitantly have both a marked susceptibility for infections and a decreased response to vaccines suggesting a T cell immune defect (7). Finally, ESRD patients exhibit a low-grade inflammation status (36). This association is typical of the “inflammaging” state observed in elderly.
Premature thymic involution is a key component of ESRD-associated immune senescence. Others and we reported that thymic output decreased with progression of CKD. Thymic output is comparable between 40-year-old uremic patients and 80 year-old non-uremic patients (5). Our group recently reported that, in ESRD patients, low thymic output was predictive of severe infections (5). The decrease in RTE could be the result of a reduction in the thymic output of naïve T cells and/or of a reduction in homeostatic proliferation. Premature loss of thymic function is likely to explain the decrease in naïve T cells in young patients with ESRD. Indeed, decreased CD4 naïve T cells percentage is also observed in paediatric CKD patients (37). Moreover, concordant data in animals suggest that acute renal failure accelerates thymus involution (38, 39).
However, there are few data documenting potential causes for premature thymic involution during chronic kidney disease. Chronic inflammation is likely to markedly contribute to immune ageing. Of note, a recent study shows that CRP levels inversely correlates with naïve T cells in haemodialysis patients suggesting either that inflammation and immune senescence evolve in parallel or that one is driving the other one (40). Activation of innate immunity, characterised by monocyte activation and overproduction of inflammatory cytokines such as Il-6, is a key feature of the CKD immune system (4, 41, 42). Thus, Jurk et al. (43) reported that knockout of the nfkb1 subunit of the transcription factor NF-κB induces chronic low-grade inflammation that leads to premature ageing in mice. Treating reversible source of inflammation is obviously a goal in CKD patients and such strategy may reduce premature ageing.
Main mechanisms of premature immune ageing are summarised in Figure 1.
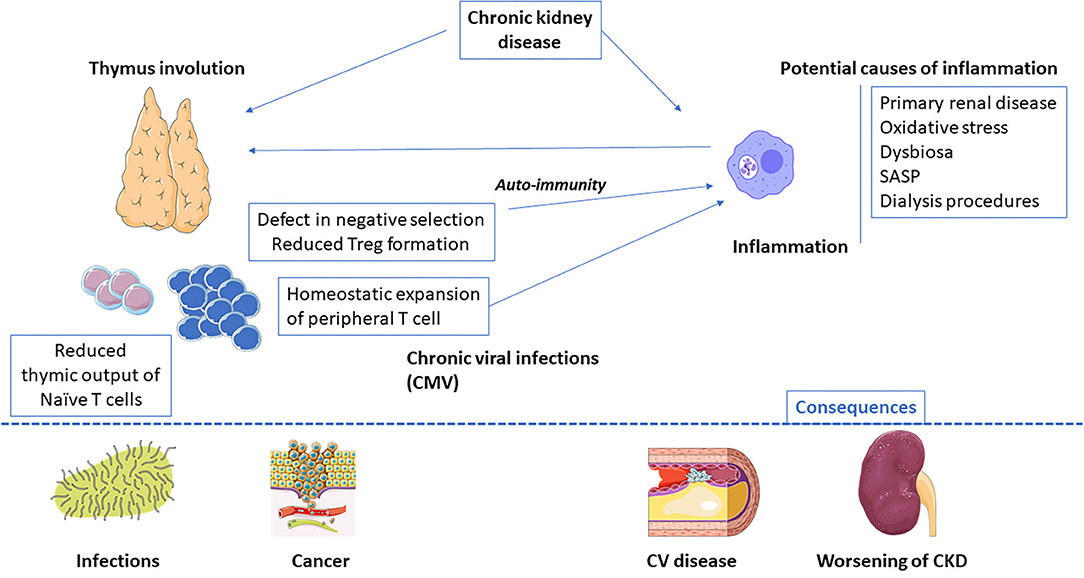
Figure 1. End-stage renal disease-related inflammaging: main causes and potential consequences. CMV, Cytomegalovirus; SASP, senescence-associated secretory phenotype.
Immune Rejuvenation: Facts and Perspectives in CKD
Immune senescence has deleterious consequences. Susceptibility to infection, premature cardiovascular disease, and increased cancer incidence are some of the most frequent and serious. A number of measures, from the simplest to the more complex, may be susceptible to reverse immune senescence, especially premature thymic involution (Figure 2).
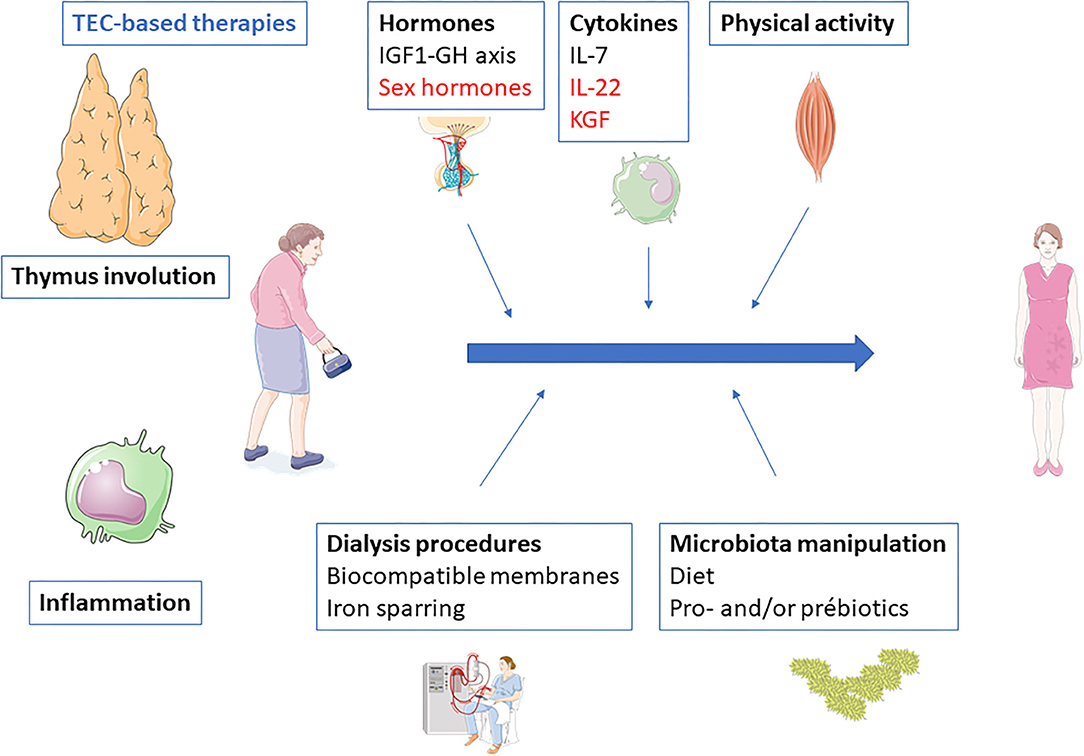
Figure 2. Potential therapeutic interventions to prevent or reverse end-stage renal disease-related inflammaging. Black, interventions with evidences; Blue, interventions without clinical evidence but with strong background and potentiality; Red, interventions with evidences of inefficacy.
Physical Activity
The impact of physical activity in maintaining thymic activity must not be neglected. It is one of the rare therapeutic strategies with consistent results in both animal and human studies (44).
In an immunological ageing mouse model, 4 weeks of free-wheel running increased naïve T lymphocytes and reduced effector ratio of cytotoxic T lymphocytes (45). Concordant data also exist in humans. Comparing adults (55–79 years) who had intensive sportive practise (cycling), age-matched adults and young sedentary adults, Duggal et al. (46) observed increased frequency of naïve T cells and RTE in cyclists. Sportsmen had also higher levels of IL-7 and lower levels of IL-6. By contrast, CD28-CD57+CD8+ T cell frequencies did not differ between the three groups. Even more powerful are the evidence that sustained physical activity in elderly improve immune responses against influenza vaccine (47, 48).
Skeletal muscles express and secrete different cytokines, also called myokines. Among them, IL-7 and IL-15 are released during exercise (49, 50). IL-6 is also released by muscle during exercise. Nevertheless, whereas IL-6 secreted through NF-KB signalling is pro-inflammatory, IL-6 produced by muscles is dependent on JUN N-terminal kinase and activator protein 1 signalling and exhibits anti-inflammatory properties (51, 52). Proof of concept is supported by experiments showing that both exercise and IL-6 infusion suppress inflammation induced by endotoxin injection (53). Modulation of cytokine secretion by muscles during exercise are likely to explain the link between physical activity and thymopoiesis.
Physical activity is often reduced in CKD patients. Sedentary life, socio-economics conditions, comorbidities, and uremia-related asthenia contribute to the reduced physical activity. Although a large number of studies reported the beneficial effects of exercise in CKD patients, no data are available concerning the potential consequences on immune status. However, other benefits of physical exercise in ESRD patients have been largely reported and physical rehabilitation programs should be encouraged in these patients. Further studies should analyze whether physical activity may at least in part reverse or prevent thymic involution and inflammation.
Hormones
Many hormonal pathways play a role in thymic physiology. However, most of them are impaired during chronic renal failure.
IGF-1-GH Pathway
The IGF-1–GH pathway interferes with many aspects of thymus biology. TECs express GH receptors (54) and IGF is expressed in the thymus (55). Growth hormone supplementation increases thymic cytokine production and T cell progenitor recruitment into the thymus and can reverse thymic involution (56–58). Hansen et al. (59) reported that treatment with rhGH increased thymus size, T cell receptor excision circles (TREC) frequency, and total TREC content in CD4 T cells in HIV-infected patients. GH withdrawal in patients receiving GH treatment is followed by decreased thymic output and intra-thymic T cell proliferation (60).
The IGF-1-GH axis is profoundly altered in dialysis patients. ESRF patients have increased GH secretion, but normal IGF-1 concentrations, indicating GH resistance (61, 62). The resistant state is related to alterations at several levels of GH/IGF-1 axis, GH signalling, and IGF-1 action (63, 64). Several studies reported that GH administration might increase IGF-1 levels in dialysis patients as in healthy subjects (65). Moreover, large studies confirmed the safety of long-term administration of GH in dialysis patients (66).
All these data suggest that GH may be a therapeutic hope to reverse thymopoiesis defect in ESRD patients.
Sex Hormones
The effects of sex hormones on thymus are well-known. A number of studies demonstrated that sex steroid ablation delay or reverse thymus involution in both animals and humans (67, 68). Sex steroids inhibit TEC expression of Notch ligand Delta-like 4 that promotes T cell differentiation and development (69).
Surgical castration is obviously not a therapeutic option in humans, but LHRH analogues use is also associated with thymic rejuvenation (70). Leuprolide desensitises LHRH receptors and reduce the release of LH and FSH. Goldberg et al. (71) showed that Leuprolide enhances T cell reconstitution following allogeneic bone marrow transplantation in mice. Similar data have been obtained in non-human primates (72). Leuprolide induces thymic rejuvenation in aged male baboons (72). Castration by Leuprolide is reversible and, due to the use of LHRH agonists in a variety of human diseases, safety, pharmacokinetics and efficacy are well-known.
Nevertheless, some studies also suggest that castration-induced thymic rejuvenation is only transient and potentially hazardous. Indeed, sex hormones deprivation favours self-reactivity (73, 74). Concordant with this concern, castration decreased CD4+CD25+ Treg and increased natural (NK) cells in humans (75). Moreover, androgens increase autoimmune regulator (AIRE) expression in mTEC and therefore enhance negative thymocyte selection while estrogens have opposite effects (76).
Despite some former results, the use of chemical castration to enhance thymic rejuvenation is consequently not a safe option.
Cytokines
IL-7
IL-7 is produced by both thymic stromal cells and bone marrow. IL-7 mediates lymphopoiesis of both T and B cells, and in the thymus, promotes proliferation, differentiation, and survival of thymocytes (77). IL-7 signals through its receptor IL-7R (78). Loss of function mutations in IL-7R leads to severe combined immunodeficiency (SCID) (78).
Administration of IL-7 in mice expand both naïve and memory CD4 and CD8 peripheral T cells (79).
RhIL-7 has been used in different clinical settings and constantly leads to increase circulating T cell populations, with more specific expansion of RTE, naïve T cells and central memory T cells (80–83). TCR repertoire diversification is also observed in rhIL-7 treated patients (84). The increase in both CD4+ and CD8+ T cell remain for months after the end of treatment by rhIL-7 (85).
IL-7 concentrations have been found to be elevated in CKD (86) suggesting a possible relative resistance to this cytokine. Nevertheless, to date, there is no study assessing the effects of rhIL-7 in lymphopenic CKD patients. Our group recently begun a phase II study (INDIA Study NCT…) using rhIL-7 to reverse thymic involution in ESRD patients on dialysis.
IL-22
Interleukin-22, also called IL-10-related T cell-derived inducible factor (IL-TIF) (87), is a member of the IL-10 family, including IL-19, IL-20, IL-24, IL-26, IL-28, and IL-29. IL-22R1 determines the cellular sensitivity toward IL-22. This receptor is restricted to specific cell types and is absent on immune cells (88). Il-22 interacts with IL-2R on the surface of TEC and allows both survival and proliferation of thymocytes.
IL-22 administration to mice having received total body irradiation increases both thymocytes and TEC recovery (89). Similar observations have been done after murine allogeneic hematopoietic cell transplant (90). IL-22 increases the number of TEC via a stat3-dependent signalling (91). More recently, it was shown that, after allogeneic hematopoietic transplantation, plasma IL-22 levels positively correlated with blood TREC levels (92).
Limitations in the therapeutic use of rhIL-22 are based on its dual effects, which strictly depend on the context. The pro-regenerative effects of IL-22 could be counterbalanced by its inflammatory and tumorigenic properties.
Keratinocyte Growth Factor
KGF belongs to the fibroblast growth factor family. This cytokine is involved in epithelial cell proliferation and differentiation in many tissues, including the thymus. KGF KO mice have impaired thymopoiesis and peripheral T-cell recovery after allogeneic bone marrow transplant (93). Moreover, KGF administration to mice enhance thymopoiesis and accelerate thymic recovery after irradiation (93, 94). In non-human primates, KGF enhances immune reconstitution after autologous hematopoietic progenitor cell transplantation (95, 96).
More recently, conflicting results made the benefits of KGF less clear. Coles et al. (97) reported on treatment with Palifermin (KGF) in patients having received alemtuzumab, a monoclonal anti-CD52 antibody, which induces profound and sustained T cell lymphopenia. Six months after treatment, individuals having received Palifermin had fewer naïve CD4+ T cells and sjTREC, leading to study discontinuation (97). Furthermore, in HIV-infected patients, Palifermin was not effective in either improving thymic function or rising circulating CD4+ T cells (98). Finally, Palifermin was associated with worse clinical outcomes in patients with acute respiratory distress syndrome (99).
All these results underline the difficulty to export results obtained in animal studies to humans and are to make cautious on KGF use.
Foxn1- and TEC-Based Approaches
Some studies tested whether TEC stem cell may help to restore thymic function.
In a mouse model, Kim et al. (100) reported that engraftment of young TEC allows thymic growth and increased T cell production. FOXN1-induced TEC from fibroblasts support CD4+ and CD8+ T cells development. Transplantation of such cells allows the formation of a complete thymus containing all the TEC subtypes required for T-cell differentiation (101). Another group recently confirmed the feasibility and relevance of such a strategy (102). Moreover, forced expression of FOXN1 in involuted thymus results in thymic regeneration with increased thymopoiesis and naïve T cell output (103). The structure of the regenerated thymus was very close to young thymus in terms of architecture and gene expression. These results suggests that up-regulation of FOXN1 is sufficient to reverse age-related thymic involution. Finally, recombinant FOXN1 protein fused with cell-penetrating peptides increased the number of TEC and enhanced thymopoiesis after hematopoietic stem cell transplantation in mice (104).
All together, these studies suggest that the FOXN1 axis research is a valuable strategy to reverse thymic involution. To date, there are no evaluation of FOXN1 expression during CKD.
Microbiota
Microbiota interferes with the immune system lifelong and its dysregulation results in inflammation (105). After great variations during the neonatal and early life periods, more subtle changes occur in microbiota until middle age before final stabilisation (106). Nevertheless, age-related changes in intestinal functions, inflammation, and co-morbidities may contribute to dysbiosis (107).
Whether microbiota interferes with immune senescence is challenging because the relative part of microbiota and health status are difficult to isolate. Moreover, even when dysbiosis may favour inflammation, inflammation may also promote dysbiosis asking the question of which came first the chicken or the egg? Indeed, chronic inflammation is a potent driver of increased gut permeability and microbial dysbiosis. For instance, age-induced dysbiosis is reduced in TNF KO mice as compared with wild type (108). Moreover, some cytokines decrease expression of tight junction proteins favours gut permeability, bacterial translocation, and systemic inflammation (109).
There are scarce but convincing data suggesting that aged microbiota contributes to drive immune senescence. Young germ-free (GF) mice raised with aged mice exhibit an inflammatory profile characterised by elevated inflammatory cytokines and macrophage activation (108). This effect was not observed when young GF mice were co-housed with young mice (108). Fransen et al. (109) reported on the transfer of gut microbiota from conventional old mice to young GF mice. T cell activation occurs in young GF mice after transfer of microbiota. Inflammation was related to higher levels of Proteobacteria and lower levels of Akkermansia in old CV mice. Once again, these alterations in immune status were not observed after transfer of microbiota from young conventional mice.
Short-chain fatty acid levels decreased in elderly. Yet, SCFA lead to increase Treg cell differentiation (110). SCFA supplementation, namely butyrate, suppresses arthritis in mice by a Breg-dependent mechanism (111). Precisely, Butyrate increases the levels of 5-HIAA (5-hydroxyindole-3-acetic acid) which activates the ary-hydrocarbon receptor, a transcriptional marker for Breg function (111).
Administration of high dose probiotics in elderly subjects enhanced CD8+CD25+ T cells and NK cells while low dose increased CD4+CD25+ and B lymphocytes (112). A more recent study reported that a probiotic mixture increased naive and regulatory T cells and decreased memory T cells (113).
Finally, best evidence of interactions between microbiota and immune senescence come from studies reporting better vaccine responses against influenza after treatment with pre- or probiotics. Akatsu et al. (114) performed a randomised study in elderly receiving enteral tube feeding. Patients received either a placebo or Bifidobacterium longum BB536. After influenza vaccine, patients having received BB536 exhibited higher levels of anti-H1N1 antibodies. Boge et al. (115) also showed increased response to influenza vaccination in elderly following prolonged administration of a probiotic. Other recent studies suggest that probiotics and prebiotics are effective to improve seroconversion and seroprotection after influenza vaccines (116, 117).
Dysbiosis is a hallmark of chronic kidney disease (118). Accumulation of uremic toxins in CKD leads to an insuatable excretion of urea, uric acid, and oxalates in the intestinal lumen (119). The enrichment in uremic toxins cause substantial modifications in gut physiology mainly an increased in permeability and in microbiota with an increase in uricase and urease-producing bacteria (120). Proteolytic fermentation leads to the formation of different uremic toxins such as p-cresyl sulphate and indoxyl-sulphate potentially aggravating the uremic status (120). Overgrowth of Bacteroidetes, Firmicutes, Ruminococcaceae and clostridia together with low abundance of Lactobacilli, Prevotellae, and bifidobacterium species depict main characteristics of intestinal microbiota of CKD patients compared to healthy subjects (121). Dysbiosis and increased gut permeability take account for bacterial translocation and inflammation (122). More recently, we reported (123) that the proportion of the inflammatory 14-carbon chain lipid A-LPS was increased in ESRD patients compared to healthy volunteers. Conversely, proportion of anti-inflammatory 18-carbon chain lipid A-LPS was decreased. Moreover, sera with predominance of 14-carbon chain lipid A-LPS induced higher secretion of pro-inflammatory cytokines than those with predominance of 18-carbon chain lipid A-LPS. TLR4 or LPS antagonists decreased LPS-induced cytokine production by monocytes, demonstrating an LPS-specific effect. This suggests that septic inflammation observed in ESRD is at least in part related to a shift toward more inflammatory LPS subtypes from altered microbiota.
Different uremic toxins are generated in the intestine and contribute to inflammation in CKD patients (124). p-Cresol is a product of the bacteria metabolization of the aromatic amino acid tyrosine in the colon. Increased levels of p-Cresol in CKD patients correlate with the expansion of terminally differentiated CD8+ T cells (125). A recent randomised study reported that nutritional intervention based on very low protein diet modifies microbiota toward a potential anti-inflammatory profile and reduces p-Cresyl Sulphate (126). This suggests that dietary interventions may mitigate uremic syndrome and immune ageing through microbiota modulation.
Kidney Transplantation
Successful kidney transplantation reverses renal failure and increases life expectancy. Contrasting effects of kidney transplantation have been observed on immune senescence.
Our group studied markers of immune senescence before and after kidney transplantation. In patients not having received polyclonal antithymocyte globulins (ATG), both T cell relative telomere length and telomerase activity increased after transplantation whereas they were not modified in ATG-treated patients (127). This suggests that renal function recovery may induce a partial reversion of immunesenescence. Nevertheless, Meijers et al. (128) did not observe such changes in T cell RTL after transplantation.
By contrast, concordant data exist to state that thymic output do not increase in non-ATG treated patients and decrease in those having received ATG (127, 128). In vitro, ATG binds to TEC and exerts a complement-independent, dose-dependent cytotoxicity (129). Nevertheless, Preville et al. (130) suggested that ATG could not enter into the thymus. Indeed, the authors observed a dose-dependent T cell depletion in spleen and lymph nodes but not in the thymus. However, these first results were not confirmed in a swine model in which lymphodepletion occurs in the thymus after administration of ATG (131). Alternatively, ATG may decrease lymphoid progenitors (127).
ESRD-associated CD8+ T cell expansion tends to marginally increase after transplantation mainly due to CMV reactivation (132). Nevertheless, inflammation measured by CRP or different proinflammatory cytokines substantially dropped after transplantation (122).
All together, these results suggest that kidney transplantation does not reverse ESRD-associated accelerated thymus involution. Whether this absence of effect is due to fixed immune changes or competitive effects of immunosuppressive drugs is difficult to ascertain.
Dialysis Procedures
Dialysis procedure itself is a source of inflammation. Bioincompatible membranes, prosthetic vascular accesses, PD solution are potential sources of immune activation.
Peritoneal Dialysis vs. Haemodialysis
Whether PD results in systemic inflammation is not clear. Some studies reported that longer PD duration results in higher IL-6 concentrations (133, 134), but others did not observe any increase in IL-6 or CRP levels (135). By contrast, a burst in inflammation is well-described during HD procedure (136–138). Expression of TLR2 and TLR4 on monocytes from patients on haemodialysis is increased (139) whereas the expression of TLR4 has been reported to be reduced on monocytes in patients with CKD not receiving dialysis (140). Bioincompatible dialyzer induces intermittent activation of monocytes and up-regulation of TLR4. Accordingly, we observed higher inflammatory monocytes counts in patients on HD as compared to those on PD (141).
We also reported higher relative telomerase activity in PD patients (141). Of note, some cytokines released during haemodialysis session, such as IFN-α, may inhibit telomerase activity in hematopoietic cells (142, 143).
Finally, T cell exhaustion was more pronounced in HD patients, especially in those with previous exposure to CMV (141). Different mechanisms may explain this difference. Persistent low-grade inflammation in HD patients may contribute to immune responses to self-antigens and pathological ageing by promoting T cell exhaustion. Alternatively, repeated antigenic stimulation of T cells during haemodialysis session may cause enhanced proliferation and accelerated ageing compared to PD.
Dialysis Membranes Choice as a Modulator of Inflammation
Bio-incompatible membranes induce sustained activation of innate immunity. During a dialysis session, both neutrophils and monocytes are recruited and activated. After activation, these cells release a number of pro-inflammatory cytokines and complement pathways activators (144). Simultaneously, their phagocytic functions are markedly altered (145, 146). Dialysis membranes also mediate complement activation (147, 148). These phenomena induce persistent pro-inflammatory and pro-coagulant states and partly explain the oxidative burst observed in ESRD patients. Although a direct effect of bio-incompatibility on adaptive immunity is more difficult to demonstrate, inter-connexions between innate and adaptive immunity may explain the consequences of bio-incompatibility on T cell functions (149).
A major challenge to reduce immune activation during dialysis is the development and use of more biocompatible membranes.
Membranes may be modified to reduce oxidative stress. For instance, vitamin E-coded dialyzers reduced indoleamine 2,3-dioxygenase-1 activity and nitric oxide formation (150). Of note, TEC are especially vulnerable to oxidative DNA damage. Thymic stromal deficiency in catalase induces thymic atrophy (151). Treatment with antioxidant can delay the onset of thymus involution (151).
Recently, median cut-off (MCO) membranes characterised by wider pores and more uniformity in pore size were developed. These membranes reduce uremic toxins at a greater degree (152). A randomised study showed that MCO significantly decrease the expression of TNF-α mRNA and IL-6 mRNA in PBMC compared to high-flux dialyzers (153). Polymethyl methacrylate (PMMA) membranes can remove large-weight molecular substances thanks to their adsorptive capacities (154). PMMA membranes seem to be associated with lower pre-dialysis values of IL-6 (155). Contrary to other dialyzers, PMMA membranes are able to clear sCD40 which accumulation in ESRD is associated with unresponsiveness to hepatitis B vaccine (156).
Even when dialysis membrane influence cytokines clearance, complement and coagulation activation, and removal of uremic toxins, a direct impact on immune senescence is not yet proven. However, there are, as described above, several mechanisms linking inflammation and immune ageing. Further studies should examine the effects of different membranes on adaptive immunity, vaccine responses, and clinical outcomes.
Iron Supplementation
Iron supplementation is widely used in HD (157). Intravenous iron administration induces oxidative stress (158). Iron overload is associated with shorter telomere length in ESRD patients (141). Association between iron overload and telomere length has been reported in different studies (159–161). Reduced telomere length is associated with mortality in dialysis patients (5). Excessive iron load enhances ferroptosis (162), which has an important role in sterile inflammatory conditions such as tissue acute injury, ischemic-reperfusion injury, and neurotoxicity.
Conclusion
Premature thymic involution and chronic inflammation greatly contribute to increased morbidity and mortality in CKD patients. Mechanisms are likely to be multiple and interlinked. Even when the quest to fountain of youth is a pipe dream, there are many scientific opportunities to prevent or to, at least in part, reverse CKD-related immune senescence. Further studies should precisely define most important pathways driving premature immune ageing in CKD patients and best therapeutic options to control them.
Author Contributions
DD, ML, and TC wrote the paper. PS and JB corrected the proofs. All authors participate in the works supporting this review.
Funding
This work was supported by grants from the Fondation Transplantation, the PHRC 2005 and 2011 (to DD), the Fondation de France (Appel d'offre Maladies Cardiovasculaires 2007 #2007 001859 to PS), the DHOS/INSERM/INCa (Appel d'offre Recherche Translationnelle 2008 to DD and PS), and the APICHU 2010 (to JB), from the Agence Nationale de la Recherche (Labex LipSTIC, ANR-11-LABX-0021) and the Région de Franche-Comté (support to Labex LipSTIC to PS). JB, CC, and TC received financial support from the Fondation Transplantation (#ET-031211 and #ET-050320, respectively). This work was a part of the RIALTO (Research in Immunology of AtheroscLerosis after TransplantatiOn) program. This work was also sponsored by the Fédération hospitalo-universitaire INCREASE (INtegrated Centre for Research in Inflammatory DisEASEs).
Conflict of Interest
The authors declare that the research was conducted in the absence of any commercial or financial relationships that could be construed as a potential conflict of interest.
Publisher's Note
All claims expressed in this article are solely those of the authors and do not necessarily represent those of their affiliated organizations, or those of the publisher, the editors and the reviewers. Any product that may be evaluated in this article, or claim that may be made by its manufacturer, is not guaranteed or endorsed by the publisher.
References
1. Li PK, Chow KM. Infectious complications in dialysis-epidemiology and outcomes. Nat Rev Nephrol. (2011) 8:77–88. doi: 10.1038/nrneph.2011.194
2. Krueger KM, Ison MG, Ghossein C. Practical guide to vaccination in all stages of CKD, including patients treated by dialysis or kidney transplantation. Am J Kidney Dis. (2020) 75:417–25. doi: 10.1053/j.ajkd.2019.06.014
3. Ducloux D, Bresson-Vautrin C, Kribs M, Abdelfatah A, Chalopin JM. C-reactive protein and cardiovascular disease in peritoneal dialysis patients. Kidney Int. (2002) 62:1417–22. doi: 10.1111/j.1523-1755.2002.kid562.x
4. Cobo G, Lindholm B, Stenvinkel P. Chronic inflammation in end-stage renal disease and dialysis. Nephrol Dial Transplant. (2018) 33(suppl_3):iii35–40. doi: 10.1093/ndt/gfy175
5. Crépin T, Legendre M, Carron C, Vachey C, Courivaud C, Rebibou JM, et al. Uraemia- induced immune senescence and clinical outcomes in chronic kidney disease patients. Nephrol Dial Transplant. (2020) 35:624–32. doi: 10.1093/ndt/gfy276
6. Yoon JW, Gollapudi S, Pahl MV, Vaziri ND. Naive and central memory T-cell lymphopenia in end-stage renal disease. Kidney Int. (2006) 70:371–6. doi: 10.1038/sj.ki.5001550
7. Litjens NH, Huisman M, van den Dorpel M, Betjes MG. Impaired immune responses and antigen-specific memory CD4+ T cells in hemodialysis patients. J Am Soc Nephrol. (2008) 19:1483–90. doi: 10.1681/ASN.2007090971
8. Crespo J, Sun H, Welling TH, Tian Z, Zou W. T cell anergy, exhaustion, senescence, and stemness in the tumor microenvironment. Curr Opin Immunol. (2013) 25:214–21. doi: 10.1016/j.coi.2012.12.003
9. Miller JFAP. The function of the thymus and its impact on modern medicine. Science. (2020) 369:eaba2429. doi: 10.1126/science.aba2429
10. Aspinall R, Andrew D. Thymic involution in aging. J Clin Immunol. (2000) 20:250–6. doi: 10.1023/A:1006611518223
11. De Haan G, Lazare SS. Aging of hematopoietic stem cells. Blood. (2018) 131:479–87. doi: 10.1182/blood-2017-06-746412
12. Wahlestedt M, Erlandsson E, Kristiansen T, Lu R, Brakebusch C, Weissman IL, et al. Clonal reversal of ageing-associated stem cell lineage bias via a pluripotent intermediate. Nat Commun. (2017) 8:14533. doi: 10.1038/ncomms14533
13. Beerman I, Maloney WJ, Weissmann IL, Rossi DJ. Stem cells and the aging hematopoietic system. Curr Opin Immunol. (2010) 22:500–6. doi: 10.1016/j.coi.2010.06.007
14. Young K, Borikar S, Bell R, Ku?er L, Philip V, Trowbridge JJ. Progressive alterations in multipotent hematopoietic progenitors underlie lymphoid cell loss in aging. J Exp Med. (2016) 213:2259–67. doi: 10.1084/jem.20160168
15. Sudo K, Ema H, Morita Y, Nakauchi H. Age-related characteristics of murine hematopoietic stemm cells. J Exp Med. (2000) 192:1273–80. doi: 10.1084/jem.192.9.1273
16. Latchney SE, Calvi LM. The aging hematopoietic stem cell niche: phenotypic and functional changes and mechanisms that contribute to hematopoietic aging. Semin Hematol. (2017) 54:25–32. doi: 10.1053/j.seminhematol.2016.10.001
17. Sun L, Brown R, Chen S, Zhuge Q, Su DM. Aging induced decline in T-lymphopoiesis is primarly dependent on status of progenitoc niches in the bone marrow and thymus. Aging. (2012) 4:606–19. doi: 10.18632/aging.100487
18. Anderson G, Jenkinson EJ. Lymphostromal interactions in thymic development and function. Nat Rev Immunol. (2001) 1:31–40. doi: 10.1038/35095500
19. Vaidya HJ, Briones Leon A, Blackburn CC. FOXN1 in thymus organogenesis and development. Eur J Immunol. (2016) 46:1826–37. doi: 10.1002/eji.201545814
20. Pantelouris EM. Absence of thymus in a mouse mutant. Nature. (1968) 217:370–1. doi: 10.1038/217370a0
21. Rode I, Martins VC, Kublbeck G, Maltry N, Tessmer C, Rodewald HR. Foxn1 protein expression in the developing, aging, and regenerating thymus. J Immunol. (2015) 195:5678–87. doi: 10.4049/jimmunol.1502010
22. Sun L, Guo J, Brown R, Amagai T, Zhao Y, Su D-M. Declining expression of a single epithelial autonomous gene accelerates age-related thymic involution. Aging Cell. (2010) 9:347–57. doi: 10.1111/j.1474-9726.2010.00559.x
23. Linton PJ, Dorshkind K. Age-related changes in lymphocyte development and function. Nat Immunol. (2004) 5:133–9. doi: 10.1038/ni1033
24. Wertheimer AM, Bennett MS, Park B, Uhrlaub JL, Martinez C, Pulko V, et al. Aging and cytomegalovirus infection differentially and jointly affect distinct circulating T cell subsets in humans. J Immunol. (2014) 192:2143–55. doi: 10.4049/jimmunol.1301721
25. Hernandez-Segura A, Nehme J, Demaria M. Hallmarks of cellular senescence. Trends Cell Biol. (2018) 28:436–53. doi: 10.1016/j.tcb.2018.02.001
26. He S, Sharpless NE. Senescence in health and disease. Cell. (2017) 169:1000–11. doi: 10.1016/j.cell.2017.05.015
27. Coppe JP, Patil CK, Rodier F, Sun Y, Munoz DP, Goldstein J, et al. Senescence-associated secretory phenotypes reveal cell-nonautonomous functions of oncogenic RAS and the p53 tumor suppressor. PLoS Biol. (2008) 6:2853–68. doi: 10.1371/journal.pbio.0060301
28. Prata L, Ovsyannikova IG, Tchkonia T, Kirkland JL. Senescent cell clearance by the immune system: emerging therapeutic opportunities. Semin Immunol. (2018) 40:101275. doi: 10.1016/j.smim.2019.04.003
29. Burton DGA, Stolzing A. Cellular senescence: immunosurveillance and future immunotherapy. Ageing Res Rev. (2018) 43:17–25. doi: 10.1016/j.arr.2018.02.001
30. Plowden J, Renshaw-Hoelscher M, Engleman C, Katz J, Sambhara S. Innate immunity in aging: impact on macrophage function. Aging Cell. (2004) 3:161–7. doi: 10.1111/j.1474-9728.2004.00102.x
31. Solana R, Tarazona R, Gayoso I, Lesur O, Dupuis G, Fulop T. Innate immunosenescence: effect of aging on cells and receptors of the innate immune system in humans. Semin Immunol. (2012) 24:331–41. doi: 10.1016/j.smim.2012.04.008
32. Brunner S, Herndler-Brandstetter D, Weinberger B, Grubeck-Loebenstein B. Persistent viral infections and immune aging. Ageing Res Rev. (2011) 10:362–9. doi: 10.1016/j.arr.2010.08.003
33. Coder B, Su DM. Thymic involution beyond T-cell insufficiency. Oncotarget. (2015) 6:21777–8. doi: 10.18632/oncotarget.4970
34. Thomas R, Oh J, Wang W, Su D-M. Thymic atrophy creates holes in Treg-mediated immuno-regulation via impairment of an antigen-specific clone. Immunology. (2021) 163:478–92. doi: 10.1111/imm.13333
35. Kooman JP, Kotanko P, Schols AM, Shiels PG, Stenvinkel P. Chronic kidney disease and premature ageing. Nat Rev Nephrol. (2014) 10:732–42. doi: 10.1038/nrneph.2014.185
36. Ebert T, Pawelzik S-C, Witasp A, Arefin S, Hobson S, Kublickiene K, et al. Inflammation and premature ageing in chronic kidney disease. Toxins. (2020) 4:227. doi: 10.3390/toxins12040227
37. George RP, Mehta AK, Perez SD, Winterberg P, Cheeseman J, Johnson B, et al. Premature T cell senescence in pediatric CKD. J Am Soc Nephrol. (2017) 28:359–67. doi: 10.1681/ASN.2016010053
38. Levine S, Saltzman A. Are urea and creatinine uremic toxins in the rat? Ren Fail. (2001) 23:53–9. doi: 10.1081/JDI-100001283
39. Raskova J, Czerwinski DK, Shea SM, et al. Cellular immunity and lymphocyte populations in developing uremia in the rat. J Exp Pathol. (1986) 2:229–45.
40. Xiang F, Cao X, Chen X, Zhang Z, Ding X, Zou J, et al. Decreased peripheral naïve T cell number and its role in predicting cardiovascular and infection events in hemodialysis patients. Front Immunol. (2021) 12:644627. doi: 10.3389/fimmu.2021.644627
42. Girndt M, Trojanowicz B, Ulrich C. Monocytes in uremia. Toxins. (2020) 12:340. doi: 10.3390/toxins12050340
43. Jurk D, Wilson C, Passos JF, Oakley F, Correia-Melo C, Greaves L, et al. Chronic inflammation induces telomere dysfunction and accelerates ageing in mice. Nat Commun. (2014) 2:4172. doi: 10.1038/ncomms5172
44. Duggal NA, Niemiro G, Harridge SDR, Simpson RJ, Lord JM. Can physical activity ameliorate immunosenescence and thereby reduce age-related multi morbidity? Nat Rev. (2019) 19:563–72. doi: 10.1038/s41577-019-0177-9
45. Lee J-Y, Paik Y, Kin JY. Voluntary exercise reverses immune aging induced by oxidative stress in aging mice. Exp Gerontol. (2019) 115:148–54. doi: 10.1016/j.exger.2018.08.009
46. Duggal NA, Pollock RD, Lazarus NR, Harridge S, Lord JM. Major features of immunesenescence, including reduced thymic output, are ameliorated by high levels of physical activity in adulthood. Aging Cell. (2018) 17:e12750. doi: 10.1111/acel.12750
47. Woods JA, Keylock KT, Lowder T, Vieira VJ, Zelkovich W, Dumich S, et al. Cardiovascular exercise training extends influenza vaccine seroprotection in sedentary older adults: the immune function intervention trial. J Am Geriatr Soc. (2009) 57:2183–91. doi: 10.1111/j.1532-5415.2009.02563.x
48. Kohut ML, Arntson BA, Lee W, Rozeboom K, Yoon K-J, Cunnick JE, et al. Moderate exercise improves antibody response to influenza immunization in older adults. Vaccine. (2004) 22:2298–306. doi: 10.1016/j.vaccine.2003.11.023
49. Haugen F, Norheim F, Lian H, Wensass AJ, Dueland S, Berg O, et al. IL-7 is expressed and secreted by human skeletal muscle cells. Am J Physiol Cell Physiol. (2010) 298:C807–16. doi: 10.1152/ajpcell.00094.2009
50. Rinnow A, Yfanti C, Nielsen S, Akerström TCA, Peijs L, et al. Endurance training enhances skeletal muscle interleukin-15 in human male subjects. Endocrine. (2014) 45:271–8. doi: 10.1007/s12020-013-9969-z
51. Whitham M, Chan MH, Pal M, Matthews VB, Prelovsek O, et al. Contraction- induced interleukin-6 gene transcription in skeletal muscle is regulated by c- Jun terminal kinase/activator protein-1. J Biol Chem. (2012) 287:10771–9. doi: 10.1074/jbc.M111.310581
52. Munoz-Canoves P, Scheele C, Pedersen BK, Serrano AL. Interleukin-6 myokine signaling in skeletal muscle: a double- edged sword? FEBS J. (2013) 280:4131–48. doi: 10.1111/febs.12338
53. Starkie R, Ostrowski SR, Jauffred S, Febbraio M, Pedersen BK. Exercise and IL-6 infusion inhibit endotoxin-induced TNF-a production in humans. FASEB. (2003) 17:884–6. doi: 10.1096/fj.02-0670fje
54. Ban E, Gagnerault MC, Jammes H, Postel-Vinay MC, Haour F, Dardenne M. Specific binding sites for growth hormone in cultured mouse thymic epithelial cells. Life Sci. (1991) 48:2141–8. doi: 10.1016/0024-3205(91)90147-4
55. Geenen V, Achour I, Robert F, Vandersmissen E, Sodoyez JC, Defresne MP, et al. Evidence that insulin-like growth factor 2 (IGF2) is the dominant thymic peptide of the insulin superfamily. Thymus. (1993) 21:115–27.
56. Savino W, Postel-Vinay MC, Smaniotto S, Dardenne M. The thymus gland: a target organ for growth hormone. Scand J Immunol. (2002) 55:442–52. doi: 10.1046/j.1365-3083.2002.01077.x
57. Hirokawa K, Utsuyama M, Kikuchi Y. Trade off situation between thymus and growth hormone: age-related decline of growth hormone is a cause of thymic involution but favorable for elongation of lifespan. Biogerontology. (2016) 17:55–9. doi: 10.1007/s10522-015-9590-z
58. Chen BJ, Cui X, Sempowski GD, Chao NJ. Growth hormone accelerates immune recovery following allogeneic T-cell-depleted bone marrow transplantation in mice. Exp Hematol. (2003) 31:953–8. doi: 10.1016/S0301-472X(03)00196-6
59. Hansen BH, Kolte L, Haugaard SB, Dirksen C, Jensen FK, Ryder LP, et al. Improved thymic index, density and output in HIV-infected patients following low-dose growth hormone therapy: a placebo controlled study. AIDS. (2009) 23:2123–31. doi: 10.1097/QAD.0b013e3283303307
60. Morrhaye G, Kermani H, Legros JJ, Baron F, Beguin Y, Moutschen M, et al. Impact of growth hormone (GH) deficiency and GH replacement upon thymus function in adult patients. PLoS ONE. (2009) 22:e5668. doi: 10.1371/journal.pone.0005668
61. Garcia-Mayor RV, Perez AJ, Gandara A, et al. Metabolic clearance rate of biosynthetic growth hormone after endogenous growth hormone suppression with a somatostatin analogue in chronic renal failure patients and control subjects. Clin Endocrinol. (1993) 39:337–43. doi: 10.1111/j.1365-2265.1993.tb02374.x
62. Mahesh S, Kaskel F. Growth hormone axis in chronic kidney disease. Pediatr Nephrol. (2008) 23:41–8. doi: 10.1007/s00467-007-0527-x
63. Rabkin R, Sun DF, Chen Y, Schaefer F. Growth hormone resistance in uremia, a role for impaired JAK/STAT signaling. Pediatr Nephrol. (2005) 20:313–8. doi: 10.1007/s00467-004-1713-8
64. Kamenicky P, Mazziotti G, Lombes M, Giustina A, Chanson P. Growth hormone, insulin-like growth factor-1, and the kidney: pathophysiological and clinical implications. Endocr Rev. (2014) 35:234–81. doi: 10.1210/er.2013-1071
65. Frystyk J, Djurhuus CB, Johansen T, Lange M, Smidt K, Christiansen JS. Measurement of free GH and bioactive IGF-1 in non-diabetic haemodialysis patients treated with GH for 7 days. Nephrol Dial Transplant. (2012) 27:4211–8. doi: 10.1093/ndt/gfs364
66. Kopple JD, Cheung AK, Christiansen JS, Djurhuus CB, El Nahas M, Feldt-Rasmussen B, et al. Opportunity: a large-scale randomized clinical trial of growth hormone in hemodialysis patients. Nephrol Dial Transplant. (2011) 26:4095–103. doi: 10.1093/ndt/gfr363
67. Heng TS, Goldberg GL, Gray DH, Sutherland JS, Chidgey AP, Boyd RL. Effects of castration on thymocyte development in two different models of thymic involution. J Immunol. (2005) 175:2982–93. doi: 10.4049/jimmunol.175.5.2982
68. Sutherland JS, Goldberg GL, Hammett MV, Uldrich AP, Berzins SP, Heng TS, et al. Activation of thymic regeneration in mice and humans following androgen blockade. J Immunol. (2005) 175:2741–53. doi: 10.4049/jimmunol.175.4.2741
69. Velardi E, Tsai JJ, Holland AM, Wertheimer T, Yu VW, Zakrzewski JL, et al. Sex steroid blockade enhances thymopoiesis by modulating Notch signaling. J Exp Med. (2014) 211:2341–9. doi: 10.1084/jem.20131289
70. Rao LV, Cleveland RP, Kimmel RJ, Ataya KM. Gonadotropin-releasing hormone agonist influences absolute levels of lymphocyte subsets in vivo in male mice. Immunol Cell Biol. (1996) 74:134–43. doi: 10.1038/icb.1996.18
71. Goldberg GL, King CG, Nejat RA, Suh DY, Smith OM, Bretz JC, et al. Luteinizing hormone-releasing hormone enhances T cell recovery following allogeneic bone marrow transplantation. J Immunol. (2009) 182:5846–54. doi: 10.4049/jimmunol.0801458
72. Scalea JR, Torabi R, Tena A, Tasaki M, Gillon BC, Moran S, et al. The rejuvenating effects of leuprolide acetate on the aged baboon's thymus. Transpl Immunol. (2014) 31:134–9. doi: 10.1016/j.trim.2014.09.001
73. Min H, Montecino-Rodriguez E, Dorshkind K. Reassessing the role of growth hormone and sex steroids in thymic involution. Clin Immunol. (2006) 118:117–23. doi: 10.1016/j.clim.2005.08.015
74. Griffith AV, Fallahi M, Venables T, Petrie HT. Persistent degenerative changes in thymic organ function revealed by an inducible model of organ regrowth. Aging Cell. (2012) 11:169–77. doi: 10.1111/j.1474-9726.2011.00773.x
75. Page ST, Plymate SR, Bremner WJ, Matsumoto AM, Hess DL, Lin DW, et al. Effect of medical castration on CD4+ CD25+ T cells, CD8+ T cell IFN- gamma expression, and NK cells: a physiological role for testosterone and/ or its metabolites. Am J Physiol Endocrinol Metab. (2006) 290:E856–63. doi: 10.1152/ajpendo.00484.2005
76. Dragin N, Bismuth J, Cizeron-Clairac G, Biferi MG, Berthault C, Serraf A, et al. Estrogen-mediated downregulation of AIRE influences sexual dimorphism in autoimmune diseases. J Clin Invest. (2016) 126:1525–37. doi: 10.1172/JCI81894
77. Chaudhry MS, Velardi E, Dudakov JA, van den Brick MRM. Thymus: the next(re)generation. Immunol Rev. (2016) 271:56–71. doi: 10.1111/imr.12418
78. Puel A, Ziegler SF, Buckley RH, Leonard WJ. Defective IL7R expression in T(-)B(-)NK(+) severe combined immunodeficiency. Nat Genet. (1998) 20:394–7. doi: 10.1038/3877
79. Schluns KS, Kieper WC, Jameson SC, Lefrançois L. Interleukin-7 mediates the homeostasis of naïve and memory CD8 T cells in vivo. Nat Immunol. (2000) 1:426–32. doi: 10.1038/80868
80. Lundström W, Fewkes N, Mackall CL. IL-7 in human health and disease. Semin Immunol. (2012) 24:218–24. doi: 10.1016/j.smim.2012.02.005
81. de Roquetaille C, Monneret G, Gossez M, Venet F. IL-7 and its beneficial role in sepsis-induced T lymphocyte dysfunction. Crit Rev Immunol. (2018) 38:433–51. doi: 10.1615/CritRevImmunol.2018027460
82. Thiebaut R, Jarne A, Routy JP, Sereti I, Fischl M, Ive P, et al. Repeated cycles of recombinant human interleukin 7 in HIV-infected patients with low CD4 T-cell reconstitution on antiretroviral therapy: results of 2 phase II multicenter studies. Clin Infect Dis. (2016) 62:1178–85. doi: 10.1093/cid/ciw065
83. Sheikh V, Porter BO, DerSimonian R, Kovacs SB, Thompson WL, Perez-Diez A, et al. Administration of interleukin-7 increases CD4 T cells in idiopathic CD4 lymphocytopenia. Blood. (2016) 127:977–88. doi: 10.1182/blood-2015-05-645077
84. Sportes C, Hakim FT, Memon SA, Zhang H, Chua KS, Brown MR, et al. Administration of rhIL-7 in humans increases in vivo TCR repertoire diversity by preferential expansion of naive T cell subsets. J Exp Med. (2008) 205:1701–14.
85. Levy Y, Lacabaratz C, Weiss L, Viard JP, Goujard C, Lelievre JD, et al. Enhanced T cell recovery in HIV1-infected adults through IL-7 treatment. J Clin Invest. (2009) 119:997–1007. doi: 10.1172/JCI38052
86. Pahl MV, Gollapudi S, Sepassi L, Gollapudi P, Elahimehr R, Vaziri ND. Effect of end-stage renal disease on B-lymphocyte subpopulations, IL-7, BAFF, and BAFF-receptor expression. Nephrol Dial Transplant. (2010) 25:205–12. doi: 10.1093/ndt/gfp397
87. Duhen T, Geiger R, Jarrossay D, Lanzavecchia A, Sallusto F. Production of interleukin 22 but not interleukin 17 by a subset of human skin-homing memory T cells. Nat Immunol. (2009) 10:857–63. doi: 10.1038/ni.1767
88. Wolk K, Kunz S, Witte E, Friedrich M, Asadullah K, Sabat R. IL-22 increases the innate immunity of tissues. Immunity. (2004) 21:241–54. doi: 10.1016/j.immuni.2004.07.007
89. Dudakov JA, Hanash AM, Jenq RR, Young LF, Ghosh A, Singer NV, et al. Interleukin-22 drives endogenous thymic regeneration in mice. Science. (2012) 336:91–5. doi: 10.1126/science.1218004
90. Pan B, Zhang F, Lu Z, Li L, Shang L, Xia F, et al. Donor T-cell-derived interleukin-22 promotes thymus regeneration and alleviates chronic graft-versus-host disease in murine allogeneic hematopoietic cell transplant. Int Immunopharmacol. (2019) 67:194–201. doi: 10.1016/j.intimp.2018.12.023
91. Pan B, Wang D, Li L, Shang L, Xia F, Zhang F, et al. IL-22 accelerates thymus regeneration via stat3/Mcl-1 and decreases chronic graft-versus-host disease in mice after allotransplants. Biol Blood Marrow Transplant. (2019) 25:1911–9. doi: 10.1016/j.bbmt.2019.06.002
92. Shang L, Duah M, Xu Y, Liang Y, Wang D, Xia F, et al. Dynamic plasma Il-22 is an indicator of thymic output after allogeneic hematopoietic cell transplantation. Life Sci. (2021) 265:118849. doi: 10.1016/j.lfs.2020.118849
93. Alpdogan O, Hubbard VM, Smith OM, Patel N, Lu S, Goldberg GL, et al. Keratinocyte growth factor (KGF) is required for postnatal thymic regeneration. Blood. (2006) 107:2453–60. doi: 10.1182/blood-2005-07-2831
94. Min D, Panoskaltsis-Mortari A, Kuro-O M, Holländer GA, Blazar BR, Weinberg KI. Sustained thymopoiesis and improvement in functional immunity induced by exogenous KGF administration in murine models of aging. Blood. (2007) 109:2529–37. doi: 10.1182/blood-2006-08-043794
95. Seggewiss R, Lore K, Guenaga FJ, Pittaluga S, Mattapallil J, Chow CK, et al. Keratinocyte growth factor augments in dose-dependent manner immune reconstitution after autologous hematopoietic progenitor cell transplantation in rhesus macaques. Blood. (2007) 110:441–9. doi: 10.1182/blood-2006-12-065623
96. Wils E-J, Aerts-Kaya FSF, Rombouts EJC, van Mourik I, Rijken-Schelen A, Visser TP, et al. Keratinocyte growth factor and stem cell factor to improve thymopoiesis after autologous CD34+ cell transplantation in rhesus macaques. Biol Blood Marrow Transplant. (2012) 18:55–65. doi: 10.1016/j.bbmt.2011.09.010
97. Coles AJ, Azzopardi L, Kousin-Ezewu O, Kaur Mullay H, Thompson SAJ, Jarvis L, et al. Keratinocyte growth factor impairs human thymic recovery from lymphopenia. JCI Insight. (2019) 5:e125377. doi: 10.1172/jci.insight.125377
98. Jacobson JM, Wang H, Bordi R, Zheng L, Gross BH, Landay AL, et al. A randomized controlled trial of palifermin (recombinant human keratinocyte growth factor) for the treatment of inadequate CD4+ T-lymphocyte recovery in patients with HIV-1 infection on antiretroviral therapy. J Acquir Immune Defic Syndr. (2014) 66:399–406. doi: 10.1097/QAI.0000000000000195
99. McAuley DF, Cross LjM, Hamid U, Gardner E, Elborn JS, Cullen KM, et al. Keratinocyte growth factor for the treatment of the acute respiratory distress syndrome (KARE): a randomised, double-blind, placebo-controlled phase 2 trial. Lancet Respir Med. (2017) 5:484–91. doi: 10.1016/S2213-2600(17)30171-6
100. Kim MJ, Miller CM, Shadrach JL, Wagers AJ, Serwold T. Young, proliferative thymic epithelial cells engraft and function in aging thymuses. J Immunol. (2015) 194:4784–95. doi: 10.4049/jimmunol.1403158
101. Bredenkamp N, Ulyanchenko S, O'Neill KE, Manley NR, Vaidya HJ, Blackburn CC. An organized and functional thymus generated from FOXN1-reprogrammed fibroblasts. Nat Cell Biol. (2014) 16:902–8. doi: 10.1038/ncb3023
102. Oh J, Wang W, Thomas R, Su D-M. Thymic rejuvenation via FOXN1-reprogrammed embryonic fibroblasts (FREFs) to counteract age-related inflammation. JCI Insight. (2020) 5:e140313. doi: 10.1172/jci.insight.140313
103. Bredenkamp N, Nowell CS, Blackburn CC. Regeneration of the aged thymus by a single transcription factor. Development. (2014) 141:1627–37. doi: 10.1242/dev.103614
104. Song Y, Su M, Zhu J, Di W, Liu Y, Hu R, et al. FOXN1 recombinant preotein enhances T-cell regeneration after hematopoietic stem cell transplantation in mice. Eur J Immunol. (2016) 46:1518–28. doi: 10.1002/eji.201546196
105. Honda K, Littman DR. The microbiome in infectious disease and inflammation. Ann Rev Immunol. (2012) 30:759–95. doi: 10.1146/annurev-immunol-020711-074937
106. de la Cuesta-Zuluaga J, Kelley ST, Chen Y, Escobar JS, Mueller NT, Ley RE, et al. Age- and sex-dependent patterns of gut microbial diversity in human adults. Msystems. (2019) 4:e00261–19. doi: 10.1128/mSystems.00261-19
107. Ghosh TS, Das M, Jeffery IB, O'Toole PW. Adjusting for age improves identification of gut microbiome alterations in multiple diseases. Elife. (2020) 9:e50240. doi: 10.7554/eLife.50240
108. Thevaranjan N, Puchta A, Schultz C, Naidoo A, Szamosi JC, Verschoor CP, et al. Age-associated microbial dysbiosis promotes intestinal permeability, systemic inflammation, and macrophage dysfunction. Cell Host Microbe. (2017) 12:455–66. doi: 10.1016/j.chom.2017.03.002
109. Mabbott NA. A breakdown in communication? Understanding the effects of aging on the human small intestine epithelium. Clin Sci. (2015) 129:529–31. doi: 10.1042/CS20150364
110. Haghikia A, Jörg S, Duscha A, Berg J, Manzel A, Waschbisch A, et al. Dietary fatty acids directly impact central nervous system autoimmunity via the small intestine. Immunity. (2015) 43:817–29. doi: 10.1016/j.immuni.2015.09.007
111. Rosser EC, Piper CJM, Matei DE, Blair PA, Rendeiro AF, Orford M, et al. Microbiota-derived metabolites suppress arthritis by amplifying aryl-hydrocarbon receptor activation in regulatory B cells. Cell Metab. (2020) 31:837–51. doi: 10.1016/j.cmet.2020.03.003
112. Mane J, Pedrosa E, Loren V, Gassull MA, Espadaler J, Cune J, et al. A mixture of lactobacillus plantarum CECT 7315 and CECT 7316 enhances systemic immunity in elderly subjects. A dose-response, double-blind, placebo-controlled, randomized pilot trial. Nutr Hosp. (2011) 26:228–35. doi: 10.1016/j.cmet-2020-03-003
113. Finamore A, Roselli M, Donini L, Brasili E, Rami R, Carnevali P, et al. Supplementation with bifidobacterium longum Bar33 and lactobacillus helveticus Bar13 mixture improves immunity in elderly humans (over 75 years) and aged mice. Nutrition. (2019) 63–4:184–92. doi: 10.1016/j.nut.2019.02.005
114. Akatsu H, Nagafuchi S, Kurihara R, Okuda K, Kanesaka T, Ogawa N, et al. Enhanced vaccination effect against influenza by prebiotics in elderly patients receiving enteral nutrition. Geriatr Gerontol Int. (2016) 16:205–13. doi: 10.1111/ggi.12454
115. Boge T, Remigy M, Vaudaine S, Tanguy J, Bourdet-Sicard R, van der Werf S. A probiotic fermented dairy drink improves antibody response to influenza vaccination in the elderly in two randomised controlled trials. Vaccine. (2009) 27:5677–84. doi: 10.1016/j.vaccine.2009.06.094
116. Lei WT, Shih PC, Liu SJ, Lin CY, Yeh TL. Effects of probiotics and prebiotics on immune response to influenza vaccination in adults: a systematic review and meta-analysis of randomized controlled trials. Nutrients. (2017) 9:9. doi: 10.3390/nu9111175
117. Wang B, Hylwka T, Smieja M, Surrette M, Bowdish DME, Loeb M. Probiotics to prevent respiratory infections in nursing homes: a pilot randomized controlled trial. J Am Geriatr Soc. (2018) 66:1346–52. doi: 10.1111/jgs.15396
118. Zhao J, Ning X, Liu B, Dong R, Bai M, Sun S. Specific alterations in gut microbiota in patients with chronic kidney disease: an updated systematic review. Ren Fail. (2021) 43:102–12. doi: 10.1080/0886022X.2020.1864404
119. Felizardo RJ, Castoldi A, Andrade-Oliveira V, Câmera NO. The microbiota and chronic kidney disease: a double-edged sword. Clin Transl Immunol. (2016) 5:e86. doi: 10.1038/cti.2016.36
120. Lau WL, Savoj J, Nakata MB, Vaziri ND. Altered microbiome in chronic kidney disease: systemic effects of gut-derived uremic toxins. Clin Sci. (2018) 132:509–22. doi: 10.1042/CS20171107
121. Vaziri ND, Wong J, Pahl M, Piceno YM, Yuan J, DeSantis TZ, et al. Chronic kidney disease alters intestinal microbial flora. Kidney Int. (2013) 83:308–15. doi: 10.1038/ki.2012.345
122. Carron C, Pais de Barros JP, Gaiffe E, Deckert V, Adda-Rezig H, Roubiou C, et al. End-stage renal disease-associated gut baterial translocation: evolution and impact on chronic inflammation and acute rejection after renal transplantation. Front Immunol. (2019) 10:1630. doi: 10.3389/fimmu.2019.01630
123. Adda-Rezig H, Carron C, Pais de Barros JP, Choubley H, Charron E, Rérole A-L, et al. New insights on end-stage renal disease and healthy individual gut bacterial translocation: different carbon composition of lipopolysaccharides and different impact on monocyte inflammatory response. Front Immunol. (2021) 10. doi: 10.3389/fimmu.2021.658404
124. Glorieux G, Gryp T, Perna A. Gut-derived metabolites and their role in immune dysfunction in chronic kidney disease. Toxins. (2020) 12:245. doi: 10.3390/toxins12040245
125. Chiu YL, Shu KH, Yang FJ, Chou TY, Chen PM, Lay FY, et al. A comprehensive characterization of aggravated aging-related changes in T lymphocytes and monocytes in end-stage renal disease. The iESRD study. Immun Ageing. (2018) 15:27. doi: 10.1186/s12979-018-0131-x
126. Di Lorio BR, Rochetti MT, De Angelis M, Cosola C, Marzoco S, Di Micco L, et al. Nutritional therapy modulates intestinal microbiota and reduces serum levls of total and free indoxyl sulfate and p-cresyl sulfate in chronic kidney disease (Medika study). J Clin Med. (2019) 8:1424. doi: 10.3390/jcm8091424
127. Crepin T, Carron C, Roubiou C, Gaugle B, Gaiffe E, Simula-Faivre D, et al. ATG-induced accelerated immune senescence: clinical implications in renal transplant recipients. Am J Transplant. (2015) 15:1028–38. doi: 10.1111/ajt.13092
128. Meijers RWJ, Litjens NHR, de Wit EA, Langerak AW, Baan CC, Betjes MGH. Uremia-associated immunological aging is stably imprinted in the T-cell system and not reversed by kidney transplantation. Transplant Int. (2014) 27:1272–84. doi: 10.1111/tri.12416
129. Kaebisch EM, Cho M-Y, Oh Y-S, Olfe Ll, Szyska M, Becker SC, et al. Cytotoxic effects of rabbit anti-thymocyte globulin preparations on primary human thymic epithelial cells. Transplantation. (2019) 103:2234–44. doi: 10.1097/TP.0000000000002799
130. Preville X, Flacher M, LeMauff B, Beauchard S, Davelu P, Tiollier J, et al. Mechanisms involved in antithymocyte globulin immunosuppressive activity in a nohuman primate model. Transplantation. (2001) 71:460–8. doi: 10.1097/00007890-200102150-00021
131. Pan H, Gazarian A, Mollet I, Mathias V, Dubois V, Sobh M, et al. Lymphodepletive effects of rabbit anti-pig thymocyte globulin in neonatal swines. Transplant Immunol. (2016) 39:74–83. doi: 10.1016/j.trim.2016.08.005
132. Ducloux D, Bamoulid J, Crepin T, Rebibou J-M, Courivaud C, Saas P. Posttransplant immune activation: innocent bystander or insidious culprit of posttransplant accelerated atherosclerosis. Cell Transplantation. (2017) 26:1601–9. doi: 10.1177/0963689717735404
133. Lambie M, Chess J, Donovan L, Kim YL, Do JY, Lee HB, et al. Independent effects of systemic and peritoneal inflammation on peritoneal dialysis survival. J Am Soc Nephrol. (2013) 24:2071–80. doi: 10.1681/ASN.2013030314
134. Pecoits-Filho R, Carvalho MJ, Stenvinkel P, Lindholm B, Heimbürger O. Systemic and intraperitoneal interleukin- 6 system during the first year of peritoneal dialysis. Perit Dial Int. (2006) 26:53–63. doi: 10.1177/089686080602600109
135. Cho JH, Hur IK, Kim CD, Park SH, Ryu HM, Yook JM, et al. Impact of systemic and local peritoneal inflammation on peritoneal solute transport rate in new peritoneal dialysis patients: a year prospective study. Nephrol Dial Transplant. (2010) 25:1964–73. doi: 10.1093/ndt/gfp767
136. Oldani S, Finazzi S, Botazzi B, Garlanda C, Baldassarre E, Valaperta S, et al. Plasma pentraxin-3 as a marker of biocompatibility in hemodialysis patients. J Nephrol. (2012) 25:120–6. doi: 10.5301/JN.2011.8432
137. Yamamoto T, Nascimento MM, Hayashi SY, Qureshi AR, Waniewski J, Brodin LA, et al. Changes in circulating biomarkers during a single hemodialysis session. Hemodialysis Int. (2013) 17:59–66. doi: 10.1111/j.1542-4758.2012.00720.x
138. Bitla AR, Reddy PE, Manohar SM, Vishnubhotla SV, Pemmaraju Venkata Lakshmi Narasimha SR. Effect of a single hemodialysis session on inflammatory markers. Hemodialysis Int. (2010) 14:411–7. doi: 10.1111/j.1542-4758.2010.00491.x
139. Gollapudi P, Yoon JW, Gollapudi S, Pahl MV, Vaziri ND. Leukocyte toll-like receptor expression in end-stage kidney disease. Am J Nephrol. (2010) 31:247–54. doi: 10.1159/000276764
140. Ando M, Shibuya A, Tsuchiya K, Akiba T, Nitta K. Reduced expression of Toll-like receptor 4 contributes to impaired cytokine response of monocytes in uremic patients. Kidney Int. (2006) 70:358–62. doi: 10.1038/sj.ki.5001548
141. Ducloux D, Legendre M, Bamoulid J, Rebibou JM, Saas P, Courivaud C, et al. ESRD-associated immune phenotype depends on dialysis modality and iron status: clinical implications. Immun Ageing. (2018) 15:16. doi: 10.1186/s12979-018-0121-z
142. Xu D, Erickson S, Szeps M, Gruber A, Sangfelt O, Einhorn S, et al. Interferon alpha down-regulates telomerase reverse transcriptase and telomerase activity in human malignant and nonmalignant hematopoietic cells. Blood. (2000) 96:4313–8. doi: 10.1182/blood.V96.13.4313.h8004313_4313_4318
143. Reed JR, Vukmanovic-Stejic M, Fletcher JM, Soares MV, Cook JE, Orteu CH, et al. 2004. Telomere erosion in memory T cells induced by telomerase inhibition at the site of antigenic challenge in vivo. J Exp Med. (2004) 199:1433–43. doi: 10.1084/jem.20040178
144. Dell'Oglio MP, Simone S, Ciccone M, Corciulo R, Gesualdo M, Zito A, et al. Neutrophil-dependent pentraxin-3 and reactive oxygen species production modulate endothelial dysfunction in haemodialysis patients. Nephrol Dial Transplant. (2017) 32:1540–9. doi: 10.1093/ndt/gfw363
145. Efthymios P, Spiridoula M, Periklis K, Andreas C, Sotiris T, Theodoros E, et al. The effect of dialysis modality and membrane performance on native immunity in dialysis patients. Prilozi. (2019) 40:25–32. doi: 10.2478/prilozi-2019-0011
146. Chonchol M. Neutrophil dysfunction and infection risk in end-stage renal disease. Semin Dial. (2006) 19:291–6. doi: 10.1111/j.1525-139X.2006.00175.x
147. Poppelaars F, da Costa MG, Berger S, Assa S, Meter-Arkema AH, Daha MR, et al. Strong predictive value of mannose-binding lectin levels for cardiovascular risk of hemodialysis patients. J Transl Med. (2016) 14:236. doi: 10.1186/s12967-016-1004-8
148. Lines SW, Richardson VR, Thomas B, Dunn EJ, Wright MJ, Carter AM. Complement and cardiovascular disease—the missing link in haemodialysis patients. Nephron. (2016) 132:5–14. doi: 10.1159/000442426
149. Losappio V, Franzin R, Infante B, Godeas G, Gesualdo L, Fersini A, et al. Molecular mechanisms of premature aging in hemodialysis: the complex interplay between innate and adaptive immune dysfunction. Int J Mol Sci. (2020) 21:3422. doi: 10.3390/ijms21103422
150. Sepe V, Gregorini M, Rampino T, Esposito P, Coppo R, Galli F, et al. Vitamin e-loaded membrane dialyzers reduce hemodialysis inflammaging. BMC Nephrol. (2019) 20:412. doi: 10.1186/s12882-019-1585-6
151. Griffith AV, Venables T, Shi J, Farr A, van Remmen H, Szweda L, et al. Metabolic damage and premature thymus aging caused by stromal catalase deficiency. Cell Rep. (2015) 12:1071–9. doi: 10.1016/j.celrep.2015.07.008
152. Reis T, Anwar S, de Assis da Rocha Neves F, Ronco C. Disruptive technologies for hemodialysis: medium and high cutoff membranes. Is the future now. Braz J Nephrol. (2021) 12. doi: 10.1590/2175-8239-jbn-2020-0273
153. Zickler D, Schindler R, Willy K, Martus P, Pawlak M, Storr M, et al. Medium cut-off (MCO) membranes reduce inflammation in chronic dialysis patients-a randomized controlled clinical trial. PLoS ONE. (2017) 12:e0169024. doi: 10.1371/journal.pone.0169024
154. Aoike I. Clinical significance of protein adsorbable membranes—long-term clinical effects and analysis using a proteomic technique. Nephrol Dial Transplant. (2007) 22(Suppl. 5):vl3–9. doi: 10.1093/ndt/gfm295
155. Galli F, Benedetti S, Floridi A, Canestrari F, Piroddi M, Buoncristiani E, et al. Glycoxidation and inflammatory markers in patients on treatment with PMMA-based protein-leaking dialyzers. Kidney Int. (2005) 67:750–9. doi: 10.1111/j.1523-1755.2005.67138.x
156. Contin-Bordes C, Lacraz A, de Précigout V. Potential role of the soluble form of CD40 in deficient immunological function of dialysis patients: new findings of its amelioration using polymethylmethacrylate (PMMA) membrane. NDT Plus. (2010) 3(Suppl 1):i20–7. doi: 10.1093/ndtplus/sfq033
157. Wetmore JB, Peng Y, Monda KL, Kats AM, Kim DH, Bradbury BD, et al. Trends in anemia management practices in patients receiving hemodialysis and peritoneal dialysis: a retrospective cohort analysis. Am J Nephrol. (2015) 41:354–61. doi: 10.1159/000431335
158. Liakopoulos V, Roumeliotis S, Gomy X, Dounousi E, Mertens PR. Oxidative stress in hemodialysis patients: a review of literature. Oxid Med Cell Longev. (2017) 2017:3081856. doi: 10.1155/2017/3081856
159. Shin C, Baik I. Transferrin saturation concentrations associated with telomeric ageing: a population-based study. Br J Nutr. (2017) 117:1693–701. doi: 10.1017/S0007114517001696
160. Murillo-Ortiz B, Ramirez Emiliano J, Hernandez Vazquez WI, Martinez-Garza S, Solorio-Meza S, Albarran-Tamayo F, et al. Impact of oxidative stress in premature aging and iron overl oad in hemodialysis patients. Oxid Med Cell Longev. (2016) 2016:1578235. doi: 10.1155/2016/1578235
161. Kepinska M, Szyller J, Milnerowicz H. The influence of oxidative stress induced by iron on telomere length. Environ Toxicol Pharmacol. (2015) 40:931–5. doi: 10.1016/j.etap.2015.10.002
Keywords: immune senescence, thymus, inflammaging, end-stage renal disease, kidney transplantation
Citation: Ducloux D, Legendre M, Bamoulid J, Saas P, Courivaud C and Crepin T (2021) End-Stage Renal Disease-Related Accelerated Immune Senescence: Is Rejuvenation of the Immune System a Therapeutic Goal? Front. Med. 8:720402. doi: 10.3389/fmed.2021.720402
Received: 04 June 2021; Accepted: 17 August 2021;
Published: 03 September 2021.
Edited by:
Maria J. Stangou, Aristotle University of Thessaloniki, GreeceReviewed by:
Konstantinos Bantis, Hippokration General Hospital, Thessaloniki, GreeceXiaohong Chen, Fudan University, China
Copyright © 2021 Ducloux, Legendre, Bamoulid, Saas, Courivaud and Crepin. This is an open-access article distributed under the terms of the Creative Commons Attribution License (CC BY). The use, distribution or reproduction in other forums is permitted, provided the original author(s) and the copyright owner(s) are credited and that the original publication in this journal is cited, in accordance with accepted academic practice. No use, distribution or reproduction is permitted which does not comply with these terms.
*Correspondence: Didier Ducloux, ZGR1Y2xvdXgmI3gwMDA0MDtjaHUtYmVzYW5jb24uZnI=; orcid.org/0000-0003-3841-1211