- 1IHU Méditerranée Infection, Marseille, France
- 2Aix-Marseille University, IRD, MEPHI, IHU Méditerranée Infection, Marseille, France
- 3Unité parasitologie et entomologie, Département microbiologie et maladies infectieuses, Institut de recherche biomédicale des armées, Marseille, France
- 4Aix-Marseille University, IRD, SSA, AP-HM, VITROME, Marseille, France
- 5Centre national de référence du paludisme, Marseille, France
- 6Aix-Marseille University, CNRS, EFS, ADES, Marseille, France
Endemic malaria, which claimed 229 million new cases and 409,000 deaths in 2019 mainly in Africa, was eradicated from Europe by the mid-20th century. Historical descriptions of intermittent tertian and quartan fever reported in texts of Hippocrates in Greece and Celsus in Italy suggest malaria. A few paleomicrobiology investigations have confirmed the presence of malarial parasite Plasmodium falciparum in 1st, 2nd, and 5th century infected individuals in diverse regions of Italy, and Plasmodium sp. later in Bavaria. The causative Plasmodium pathogens, discovered in the 19th century in Algeria, were controversially used as therapeutic agents in the European pharmacopeia more than two centuries after effective quinine-based treatments had been introduced in Europe. How Europe managed to eradicate malaria and what the history of malaria was in Europe are of medical interest, and this review traces research pathways for a renewed understanding of malaria eradication in Europe through combined historical and paleomicrobiological investigations.
Introduction
Malaria is a vector-borne disease in which Plasmodium spp. causative pathogens are transmitted via the bite of the infected female Anopheles mosquito (1). Malaria remains the single most prevalent life-threatening infectious disease in the world according to the World Health Organization (WHO) (2). The genus Plasmodium is composed of more than 250 species, but only five species Plasmodium falciparum, Plasmodium vivax, Plasmodium ovale Wallikeri, Plasmodium ovale curtisi, and Plasmodium malariae (3) are shown to be involve in human-to-human transmission after Anopheles bites (4). Three simian parasites Plasmodium cynomolgi, Plasmodium inui, and particularly Plasmodium knowlesi (5) are known to be responsible for human malaria (4).This last species is the most common cause of human malaria in Malaysia. Malaria reservoirs have been eradicated in Europe since the 20th century, which currently experiences only imported cases. However, according to historical texts and medical, paleomicrobiology and paleogenetic data, malaria may have played a key role in the history of past Europeans.
The oldest evidence for the Plasmodium parasite (i.e., dominicana n. sp.) was found in amber dating back 30 million years in the Dominican Republic (6). Most probably malaria then co-evolved with non-human primates in Africa: P. falciparum may have emerged from gorilla parasites about 10,000 years ago, while P. vivax may have emerged much earlier from non-specific Apes hosts (4, 7–9). On the other hand, P. knowlesi probably arose in Southeast Asia among macaque monkeys about 478,000–98,000 years ago (10), while the origins of P. malariae and P. ovale remain uncertain, although these parasites are currently associated with gorilla, chimpanzee, and bonobos in Africa (4). Malaria co-evolved over millennia alongside humans and reached tropical and temperate climatic areas of the Old World (9, 11). Malaria may have moved westward from India to Europe along with prehistoric populations (12). During the Upper Paleolithic, malaria may not have had a significant selection force, in agreement with its putative recent introduction in European populations (12). Another hypothesis regarding the introduction of malaria in Italy, relies on the introduction of Plasmodium vectors from North Africa (13) in the frame of Sardinia invasion by Carthaginians, 7th to 2nd century BC (13, 14).
As for the term malaria, it derives from the Italian word mal'aria, meaning “bad air” (15). In the Middle Ages, malaria was thought to be transmitted by humid and stale air, especially in swamps and marshes. Interestingly, the Greek word for malaria (elonosia) literally means “the disease of the marsh” (16). The term malaria was introduced in England by Horace Walpole in 1740, following his letters from Italy, but it was not until 1827 that the term “malaria” was first used in an English scientific publication (17).
Discovery of Plasmodium Pathogens
The first microbiological studies of malaria began at the end of the 19th century, during the birth of microbiology. The interest of European scientists in malaria was reinforced by the development of large colonial empires and the need to protect colonists and populations from this deadly infection (11). A French army surgeon, Alphonse Laveran, first observed Plasmodium gametocytes in patients' blood by optical microscopy in 1878 in Bône, Algeria (18). On November 6th, 1880, A. Laveran observed gametocyte exflagellation while working at the hospital in Constantine, Algeria; he noted the presence of Plasmodium parasites in the fresh blood of a 24-year-old soldier who had been febrile since October 10th, 1880 (19, 20) and this observation is regarded as the first one of P. vivax (18) (Figure 1). Laveran demonstrated that “l'impaludisme,” as it was called at the time, was not of bacterial but of parasitic origin (18). He wrote: “From then on I no longer had any doubts about the parasitic nature of the elements that I had found in palustrine blood” (18). Further discovery of the mosquito as the exclusive vector of malaria followed from the intuition of Patrick Manson, who had previously demonstrated that other blood parasites, such as certain filarial worms, were transmitted by mosquitoes (22). From this initial discovery, Manson assumed that mosquitoes could also be the vector for malaria parasites, thus associating the relationship between disease and swampy areas overpopulated by mosquitoes (22). In 1897 in India, British army surgeon Ronald Ross discovered that Plasmodium relictum was transmitted by culicine mosquitoes (20), and demonstrated 2 years later that human malaria parasites could be transmitted by Anopheles mosquitoes. In fact, in 1898 Italian malariologists Giovanni Battista Grassi, Amico Bignami and Giuseppe Bastianelli had already provided the formal proof that malaria parasites could be transmitted from man to man via Anopheles mosquitoes (23, 24). Within 2 years following this discovery, they further understood that only Anopheles females could transmit malaria parasites and described the complete cycle of P. vivax, P. falciparum and P. malariae in the mosquito (20). In 1947, Henry Shortt and Cyril Garnham demonstrated pre-erythrocytic schizonts of Plasmodium cynomolgi in the liver of a Rhesus monkey, and finally in 1982, Krotosky deciphered the dormant exo-erythrocytic hypnozoites of P. vivax, explaining malaria relapses (20). It thus took more than one century of work carried out by hundreds of scientists to elucidate and fully understand the complete cycle of human malaria parasites (24, 25).
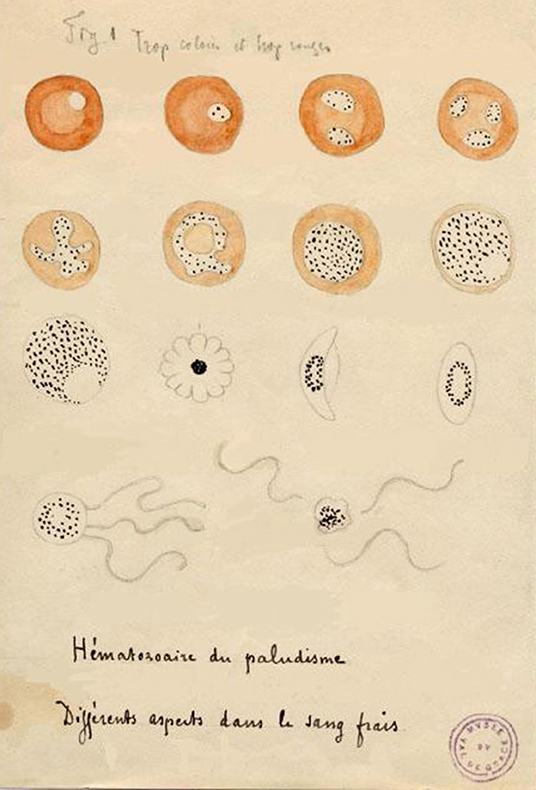
Figure 1. Drawing by Alphonse Laveran of the different aspects of hematozoan malaria' (21).
History of Fever in Europe
Antiquity (Before 476 AD)
According to historical sources, malaria may have been described for the first time during the fourth and fifth centuries BC in ancient texts, such as the Hippocratic Corpus, which mentioned a disease characterized by intermittent fevers (26). Hippocrates (460-370 BC) described episodes of benign tertian fever that have been interpreted as P. vivax malaria, while the term quartan fever could refer to P. malariae malaria (27). The Greek physician used the term “dangerous fumes” emanating from the ground that were carried by the wind to describe the mist which he believed caused serious illness (26). Hippocrates then linked the occurrence of climatic phenomena and the variation of environmental conditions to the occurrence of episodes of intermittent fevers and classified fevers according to their periodicity: tertian fever from the Greek tritaios pyretos (intense fever every 3 days) and quartan fever from Greek tetartaios pyretos (intense fever every 4 days) (26). He reported the seasonality of the disease, the proximity of cases to standing water and the use of antimalarial agents (28). These semi-tertian fevers, like other intermittent fevers, were common during the fifth century BC in certain localities of northern Greece such as Macedonia, and in Laconia (13, 16), before spreading throughout Western Europe. Apart from historical sources, there were very few reliable data concerning the possible presence of malaria during this period. However, malaria parasites may have exerted an evolutionary selection shaping the human genome, including genes for immunity, cell adhesion, and inflammation (29). However, genomic analysis of 224 human European genomes dating from the Upper Paleolithic to Roman periods have not detected specific malaria resistance mutations in genomes, such as mutations affecting the G6PD gene (glucose-6-phosphate dehydrogenase), HBB gene (hemoglobin subunit beta), or Duffy blood group, suggesting a weak adaptation to malaria or a mild form caused by P. vivax in Europe during the Prehistory, Protohistory, and Antiquity periods (12). During the Roman period, many outbreaks of deadly fever were reported, the most significant of which took place in the 1st−2nd century AD and which may have been caused by P. falciparum circulating in Italy at that time (30). Spleen remedies created by the physician Pedanius Dioscorides may have been developed in response to the endemic malaria plaguing Roman populations (31).
Middle Ages (476- 1492 AD)
Climatic and environmental variations with successive periods of warming and cooling may have influenced the emergence of certain infectious diseases on the European continent (32). Growing urbanization in Eurasia at the beginning of the Middle Ages favored pandemics through increased density of human contacts (32). The medieval population undoubtedly experienced malaria epidemics, not only in the Mediterranean region but also in Northern France, Germany and England. The most interesting historical sources are from England and Italy, while French sources suggest that certain regions were endemic for malaria in France and Italy between the 6th and 9th centuries (33). Old texts citing intermittent fevers, whether tertian or quartan, are interesting clues in tracing the history of malaria in medieval Europe. According to descriptions in these texts, researchers refer to the notion of benign tertian fever as an indicator of infection with P. vivax, and quartan fever of P. malariae, while the term malignant tertian fever may have referred to P. falciparum malaria (13, 34). However, the unique European species (Italy) of malaria identified by paleomicrobiology belonged to P. falciparum during Antiquity. In addition, the reservoirs of malaria in Europe are poorly identified and differ depending on the period and climatic variations.
Malaria probably took root in certain niches around marshes, as in Italy, creating an environment conducive to the spread and development of P. falciparum between the end of Antiquity and the beginning of the Middle Ages (35).
Modern Era (1492- 19th Century)
Due to the accumulation of several factors, such as climatic variations and the occurrence of several wars during the 17th and 18th centuries, the incidence of malaria believed to be caused by P. vivax and P. malariae was high (36). In France, the use of quinquina powder since the 17th century to treat intermittent fever may trace malaria epidemics (Box 1–3). A study carried out by the Royal Society of Medicine counted 820 cases of tertian fever, 230 cases of daily fever and 127 cases of quartan fever in the marshy areas of the Paris region from 1783 to 1785 (37).
Box 1. Quinine, a historical treatment of malaria.
Quinine, a natural compound extracted from the bark of Cinchona trees, was used historically in the high plains of South America. The genus Cinchona now includes 21 different species and the precise composition of quinine varies, depending on the tree species from which it has been extracted (44). Quinquina powder was cited by Europeans under different names, including Peruvian bark, Jesuit powder, Cinchona, quinquina, kinquina, kinkina, and the English remedies. The use of quinquina as an antipyretic drug was reported in the early 17th century by Father Antonio de la Calancha in a book he published in 1638 in Barcelona (44). Use of quinquina powder then declined, as it was believed to be ineffective or dangerous because of the confusion between real Cinchona bark and different barks of different species of the genus Cinchona (some species are devoid of quinine and others have very little quinine) (45). The apogee of quinquina powder followed its use by the English pseudo-apothecary Robert Talbot (also known as Talbor) who gained notoriety after he cured Charles II, King of England (46, 47) (Figure 2). The active ingredient of the quinquina bark, named quinine, was first isolated in Paris in 1820 by two French pharmacists, Pierre-Joseph Pelletier (1788–1842) and Joseph Bienaimé Caventou (1795–1877) from the bark of Cinchona succirubra (red Cinchona) (48) (Figure 3). Quinine was heavily used during World War I (1914–1918) by British, French and German physicians, both as a prophylaxis and as a treatment for malaria (49).
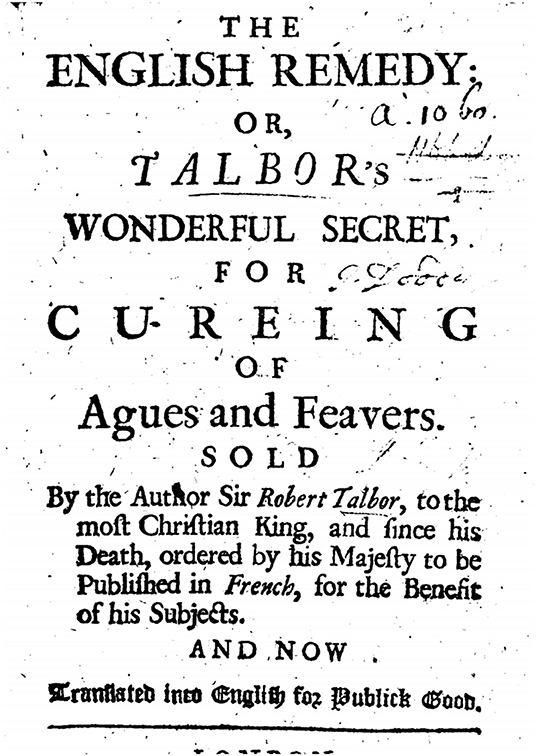
Figure 2. The english remedy: Talbor's wonderful secret for curing of agues and feavers (47).
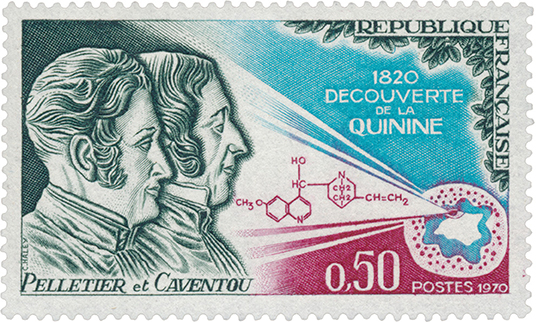
Figure 3. Postage stamp edited in 1970 with the images of Pelletier and Caventou, who first isolated the active ingredient “quinine”.
Box 2. Malarial fevers at the court of french king louis XIV.
More than 36,000 people worked in the construction of the royal palace in the swampy site of Versailles, and some contracted intermittent fevers. According to historical sources, several thousand workers may have died of malaria working on the construction of the basins in the park of Versailles (50).
The Duke Saint-Simon evoked in his memoirs the marshes surrounding the castle of Versailles during the construction and speaks of an unhealthy humidity (51). Under the reign of Louis XIV, swamps were already considered as sources of disease, and the term “miasmas” began to be used to describe “bad air” carrying disease (51). In Versailles, several inhabitants of the palace experienced intermittent fevers, notably in 1680 when the eldest son of the Sun King, Le Grand Dauphin and his daughter La Dauphine had attacks. The King summoned Robert Talbot, who thanks to the administration his remedy based on Cinchona powder, cured Le Grand Dauphin in 4 days. Very impressed by this cure, the king paid him 48,000 pounds, in addition to an annual annuity of 2,000 pounds, for the secret recipe for Talbot's remedy (51). The close relatives of Louis XIV used to drink quinquina too, and Saint-Simon refers in his memoirs to the taking of quinquina in Versailles by M. de Beauvilliers to stop a stubborn fever before going to join the new king of Spain, Philip V (52). Saint-Simon was cautious using quinquina (53), and advised his host M. de Chevreuse, who was taking a glass of quinquina to relieve gastric pains, on the risk of perforating his stomach if he took quinquina without eating (52).
During the 16th to 19th centuries in southern and eastern England, known to be very marshy areas, a high mortality rate occurred in the population, which according to old texts was due to fevers, termed “marsh fever” (38). One study suggested that swamp fever was actually malaria, assuming that the malaria species during this time were P. vivax and P. malariae (38). These fevers were often reported in temperate regions in Europe during the summer and autumn, agreeing with the hypothesis of malaria (39). The English word for malaria in the Middle Ages was “agues” and this term was continuously used until the causative Plasmodium sp. was discovered (39). William Shakespeare (1564–1616), mentioned fever in several of his plays and in the poem of Venus and Adonis: “As burning fevers, agues pale and faint, life-poisoning pestilence and frenzies wood, the marrow-eating sickness, whose attaint disorder breeds by heating of the blood; Surfeits, imposthumes, grief, and damn'd despair, swear nature's death for framing thee so fair,” as a testimony of the possible impact of malaria in England. In the 19th century malaria reached Northern Europe, affecting Finland, most probably from foreign workers during construction of the Saimaa canal and railway (40). P. vivax malaria became common in South Finland and Scandinavia until the 20th century (40). The Italian island of Sardinia had a reputation to be unhealthy because of malaria, recording a death rate six times higher than in the rest of Italy with around 300 deaths per 100,000 inhabitants during the period from 1887 to 1902 (41).
Malaria and World War I
In addition to bullets and gas, soldiers from both sides involved in World War I had to deal with invisible enemies such as infectious diseases. In addition to Bartonella quintana, malaria affected more than 1.5 million for a fatality case of 0.2–0.5%. Problems inherent to this conflict, such as such as long periods of immobility in trenches, troop movements, and soldiers' activities have amplified the numbers of epidemics in endemic malaria zone such as Italy, Macedonia, Mesopotamia, Palestine, or England (42). In 1917, 70,000 cases of malaria were reported in the British forces alone (43). After the war, malaria spreader-emerged among the civil population in malaria free areas like northern Germany, eastern England and Italy due to the return of soldiers to their homeland and to refugee movements (42, 43).
Paleomicrobiological Confirmation of Historical Malaria
Paleomicrobiology is a demonstrative field of research and practice, allowing the analysis of microorganisms responsible for past epidemics (54). Ancient texts provide starting points for the investigation of past infectious diseases, putting paleomicrobiological researches in an appropriate historical, ecological and social context (55). Paleomicrobiology was born in 1993 thanks to the work of Spigelman and Lemma with the detection by molecular biology of Mycobacterium tuberculosis DNA from an ancient human skeleton (56). The objectives of paleomicrobiology are, of course, the molecular diagnosis of ancient infectious diseases, but also tracing the genetic evolution of microorganisms, the temporal, and geographical reconstitution of ancient microbes (57).
Ancient DNA (aDNA) may persist until 1.6 Millions of years (58) with an estimated half-life of 521 years (59, 60); depending on environmental conditions which can reduce or inhibit the degradation of aDNA such as freezing or rapid postmortem desiccation. Chemical modifications that degrade aDNA are depurination (cleavage of glycosylated N bonds eliminating the purine bases) which results in aDNA fragmentation in <100-bp fragments (61–63) and an overrepresentation of purine bases at both ends of the aDNA strands (64); and the deamination of cytosines, which results in the loss of an amino group by the cytosines then converted into uracil and sequenced as thymines (61). These specific degradations make it possible to distinguish aDNA from modern DNA and its possible contamination (65, 66).
Paleo-Immunological Diagnosis
An initial paleomicrobiological study detected the P. falciparum histidine-rich protein-2 antigen (PfHRP-2) in seven Egyptian mummies from different periods, ranging from 3200 BC to 350–500 AD (67). Likewise, by using PfHRP-2 assay, P. falciparum. was detected in 30/71 (42%) of mummies from 3200 BC preserved in the Anthropological and Ethnographic Museum of Turin, with the same magnitude of malaria prevalence in endemic regions (68). An immunochromatographic and immunohistochemical study performed in 2008 by Bianucci et al. on muscle and skin samples collected from an Egyptian mummified child aged 15–18 months yielded positive detection of P. falciparum histidine-rich protein-2. Moreover, the muscle sample yielded positive results for the MSP1-19 antigen common to the genus Plasmodium (69). In Italy, studies based on immunological tests on bone samples from the Medici family of Florence, dating from the 16th century, detected proteins of P. falciparum in four members of the family (70). Also, an immunological study targeting the P. falciparum highly specific HRP-II protein on 34 individuals dating from 14th BC to the 16th century, in Sardinia indicated that malaria may date back to the Carthaginian period (502 B-C) (14).
Biomolecular Diagnosis
In 1997, the work by Taylor et al. pioneered the field of ancient malaria, with the development of a hemi-nested PCR diagnostic method targeting the plasmodial 18S rRNA gene in tissues of a Granville mummy dating from 700 BC. This PCR assay performed on a mummy positive for P. falciparum by PfHRP-2-based immunological test yielded negative results, as did one of the two positive controls introduced in the experiment (71). The technique developed by Taylor et al. was later taken up by other researchers, notably in 2001 by Sallares and Gomzi in Italy (35). These two researchers investigated an infant cemetery in Lugnano, Italy dating from 450 AD, where archaeologists suspected that malaria was responsible for the deaths. Investigating bone samples, PCR yielded positive results for P. falciparum in two samples belonging to the same individual (35). According to these data, the authors suggested the occurrence of a massive P. falciparum infection, since a positive result requires a very high level of parasitemia. Another European study occurred in 2001 by Zink et al. in southern Germany on the bones of 20 individuals dating from 1400 to 1800 AD, where the presence of an 18S rDNA sequence of Plasmodium sp. was confirmed in one individual (72, 73). Shotgun Illumina sequencing of DNA extracted from microscopic slides prepared between 1942 and 1944 by a Catalan center from the Ebro Delta, Spain, yielded a P. vivax genome exhibiting >89% alignment with the reference strain, and demonstrated variations linked to antimalarial drug resistance in this European strain (74). Studies performed on mummies of King Tutankhamun's family identified P. falciparum malaria as the probable cause of death of Tutankhamun and his relatives Yuya and Tiyi (Figure 4). The results were positive for malaria by PCR methods specific to P. falciparum targeting the STEVOR, AMA1, and MSP1 genes and negative for other tested infectious agents (plague, tuberculosis, leprosy and leishmaniasis). These results suggest that the most probable cause of death for Tutankhamen was an avascular bone necrosis associated with P. falciparum malaria (75). In 2013, PCR-sequencing investigation of soft tissue biopsies collected from Egyptian mummies dated from 806 BC to 124 AD yielded 6/16 positive for the P. falciparum AMA1 gene, and four of these mummies also proved positive for PCR-based detection of tuberculosis (76). In addition, next generation sequencing (NGS) technology in 5 random mummies confirmed P. falciparum DNA sequences (77). In 2008, Nerlich et al. used hemi-nested PCRs targeting malaria 18S rDNA to detect P. falciparum in tissue samples from Egyptian mummies of different pharaonic dynasties (ranging from 3500 to 500 BC); the authenticity of PCR products was assessed by sequencing (72). This study yielded a lower incidence of malaria in ancient Egyptian mummies than that previously recorded using paleo-immunological techniques (Figure 5) (72). In fact, these contradictory results questioned the usefulness of these methodologies (67). In particular, this method, based on the detection of monoclonal antibodies to the soluble species-specific antigen PfHRP-2 (ParaSight-F) in blood, exhibited a high error rate after detection by positive PCR tests; 60% false positives were detected (78). False positive reactions using this test can be explained by cross reaction with rheumatoid factor (RF) in the blood (79).
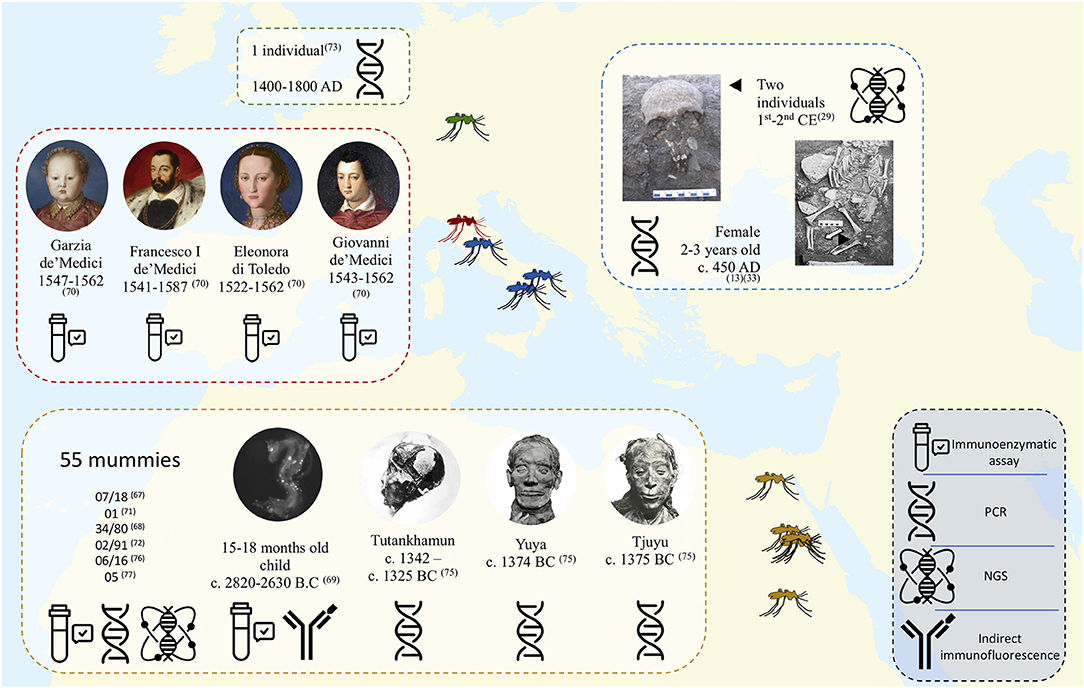
Figure 4. Map showing the major works carried out for the diagnosis of ancient malaria. all identified Plasmodium belong to the species falciparum except for one individual tested in Bavaria (73) and one Egyptian mummy (71) whose pathogen is Plasmodium sp.
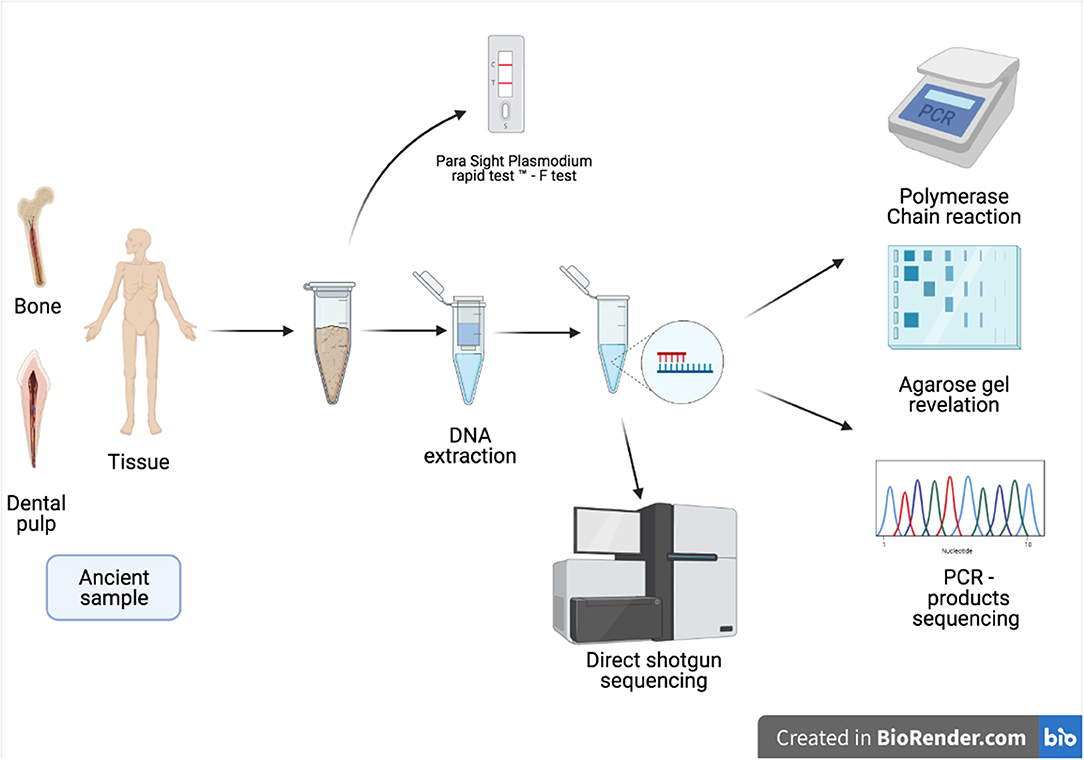
Figure 5. Schematic presentation of the techniques used in the diagnosis of malaria in ancient samples.
Paleopathology
Besides molecular diagnostic methods, direct diagnostic evidence suggests a causal link to malaria with skeletal lesions due to severe anemia resulting in a typical hyperostosis of the orbit (cribra orbitalia). Some research in paleopathology supports the hypothesis that cribra orbitalia is associated with anemia caused by infection with parasites of the genus Plasmodium, which other researchers therefore consider as a sign of malaria (80). In a study by Massa et al. in 2000 on Egyptian mummies, skeletal pathology was observed suggesting severe anemia in mummies testing positive for P. falciparum by immunological test. Anthropologists have indicated that the frequency of orbital cribra is very high (92%) in subjects positive for P. falciparum, suggesting that malaria was probably an important cause of anemia (68). In 2014, a study investigating 4,760 individuals from 29 ancient sites in the Nile Valley in Egypt, the prevalence of orbital cribra was 42.8%, with a non-correlation of proportion between age groups and sex, suggesting an infectious factor causing hemolytic anemia. These results suggest that malaria infection is responsible for cribra orbitalia, which can be proposed as an indication of possible malaria infection in ancient samples (81). Concerning Europe, skeletal investigation conducted in a Greek population dating from the 5th to 3rd centuries BC in the vicinity of the Black Sea hypothesized that malaria was endemic, which would explain the high prevalence of cribra orbitalia and porotic hyperostosis (82). In Italy, the prevalence of cribra orbitalia in Roman skeletal remains from the regions of Ravenna and Rimini dating from the 1st to the 4th century AD suggests that the observed lesions were caused by chronic acquired anemia, possibly caused by malaria. The authors have indeed argued that this area was surrounded by marshes during the Roman period (83). An osteological study conducted in Sardinia for the so-called “malaria signature” on 283 skeletons dating from 4,700 BC to 1582 AD, supported the possible introduction of malaria by Carthaginians during the 6th to 3rd century BC (84). Porotic hyperostosis is the consequence of thalassemia resulting in an overgrowth of the spongy marrow space of the skull. Accordingly, Angel supported the hypothesis that hemolytic anemia occurred under the pressure of P. falciparum (85, 86). In the regions of Cyprus, Greece, and Natalia, researchers identified the presence of Porotic hyperostosis on ten skeletons dating from 6,500 to 2,000 BC; some infantile long bones presented an internal shell of bone attached to the cortex, indicating that the red marrow hypertrophy blocked normal bone remodeling, resulting in damage caused by thalassemia (85). However, several researchers refute the hypothesis that cribra orbitalia can be caused by malaria infection. According to work conducted by Cole and Waldron in 2019, there was no direct and specific relationship in the blood dyscrasias causing anemia for which malaria agents are possibly responsible. Cribra orbitalia can then be considered as a non-specific paleopathological sign of malaria (87).
Paleogenetic approach has helped anthropologists to understand the evolution of malaria in Europe, malaria prevalence, genetic selection and ecological changes (88). Beta-thalassemia is a hemoglobinopathy with a specific mononucleotide mutation in the B-globin chain, which could confer protection against malaria following natural selection (89). The factors influencing thalassemia are diverse but the most important is undoubtedly resistance to malaria, indeed the distribution of malaria and the high prevalence of β-thalassemia are closely linked (90). The incidence rate of β-thalassemia in Sardinia is one of the highest in Europe with a majority of a cod39 mutation. Studying b-globin mutations by PCR analysis in 19 individuals dating from the Roman and Punic periods, identified the cod39 mutation in one individual, which was interpreted as clue for malaria endemicity during the Roman period in Sardinia (91). Also, complementary receptor 1 (CR1) involved in the interaction between Plasmodium and cells, is taken as an index of malaria susceptibility or protection (92). Studying CR1 single nucleotide polymorphisms (SNPs) in the Sardinian population found the prevalence of the dominant Sardinian haplotype was more closely related to that in India, than to other European ethnic groups: this observation evoked a positive selection supporting a malaria endemicity in the past (93). Another study found a high incidence of HLA-B35 in the Sardinian population, indicating that P. falciparum had probably exerted a selective and constant pressure for millennia on the Sardinian villages of low altitude (94).
Malaria Eradication Strategies in Historical Europe
Since antiquity, malaria In Europe has been associated with swamps and stale air. Consequently, many strategies have been implemented to try to clean up these high-risk areas. French authorities opted for a policy of draining marshes as early as 1599 (Edict of King Henry IV for the draining of marshes) and a grand campaign started at that time everywhere in France (95). The King called upon the Dutch engineer Hunfroy Bradleij, who was experimenting in draining marshes. In 1643, during the reign of King Louis XIV (only 5 years old at the time, so it was his regents who decided), the King granted the owners of marshlands privileges for draining their land (95). In 1767, the extent of the marshes was estimated to be equivalent to a third of the kingdom. However, when the law of September 16th, 1807 related to draining marshes was implemented, a more realistic estimate indicated the extent of the marshes at 500,000 hectares. This decreased to 299,000 hectares in 1979 following various policies for draining marshes (96). A study conducted in Great Britain of factors influencing the eradication of malaria, based on analysis of data from 1840 to 1910, an increase in the cattle population was correlated with a 20% decrease in malaria, due to the decrease in wetlands following the extension of cattle breeding (97). The 2003 British study by Kuhn et al., based on statistical studies of the occurrence of fever epidemics between 1840 and 1910, simulated the presence of malaria during this period. The results indicated that mortality due to fevers would have been 19.5% higher if the density of cattle rearing and the extent of wetlands had remained unchanged between 1840 and 1910 (97). This study confirmed the importance of these parameters in playing a role in the eradication of malaria in Great Britain (97). In the southernmost regions of Europe, including Italy, the number of cases at the end of the 19th century amounted to 2 million, mostly in the Italian islands as well as in the central and southern regions, with a mortality rate of more than 15,000 deaths per year. It was not until the beginning of the 20th century that a major effort against malaria was undertaken with the passing of a law to control the Anopheles mosquito and the free distribution of quinine to the population (98). Italy was a pioneer and can be considered the testing ground for this approach to the struggle, which was later applied by the other European countries.
In 1928–1932, Benito Mussolini organized draining and cultivating the Pontine marshes at 60 km from Rome, considered as the main historical focus of malaria in central Italy. In 1943, faced with the advance of the Allies who landed in Sicily, Germans sabotaged the water infrastructure there in order to encourage the reappearance of malaria. During the second World War dichlorodiphenyltrichloroethane (DDT) was used extensively by the military to control malaria-carrying mosquitoes, and after the war a massive use of DDT was applied in Italy to stop the proliferation of malaria vectors (99).
P. falciparum and P. vivax were endemic in Corsica, a French Mediterranean mountainous island, just after the Second World War (100). The use of DTT associated with systematic oral administration of quinine lead to malaria eradication in 1953. Mosquito control measures were gradually abandoned. P. vivax outbreak re-emerged from 1964 to 1972 with the arrival of immigrants from North Africa (101). Prophylactic drug administration and insecticide spraying were re-established until the 1980s. The last case of autochthonous P. vivax was detected in 2006 (102). However, competent mosquitos for P. falciparum malaria transmission are still present in Corsica and favorable climate may lead to the possibly re-emergence of malaria in Corsica (103).
Although the discovery of malaria parasites only occurred in the 19th century, when it was realized that malaria was not caused by bad air from the marshes but rather microorganisms in mosquitoes, this should not obscure the fact that malaria fevers existed in Europe and that they were very common and endemic in swampy areas (96).
Anopheles and Malaria
The malaria parasite is transmitted to humans through the bite of female Anopheles mosquitoes, in which the parasite develops from the gametocyte to the sporozoite stage (104). After transmission to humans by mosquito bite the parasites develop in the liver. Later, they pass into the bloodstream, where they develop inside red blood cells, which are destroyed by the release of merozoites; it is during this stage that malaria is symptomatic (104). In 1818, J.W, Meigen described for the first time mosquitoes of the genus Anopheles (105). In 1897, Ronald Ross elucidated a missing step in the cycle of malaria parasites by discovering the role of mosquitoes in the transmission of malaria parasites (20). In Europe, several species of Aedes, Culex, and Anopheles mosquitoes, which are possible vectors of parasites of the genus Plasmodium, are present on the continent (106). A 2021 study looked at modeling of the zoogeographic history of possible malaria vectors in the Mediterranean region of Europe during the Quaternary periods. The results suggest the persistence of P. vivax and the Anopheles vector in central and southern Europe, supporting a permanent survival throughout the Quaternary period until the industrial revolution and subsequent eradication campaigns (107). In Europe, An. maculipennis has been considered as the major vector of malaria. However, a 2011 study warns about the ability of another urban species, such as An. plumbeus, which, according to the authors, may be a potential source of malaria transmission in urban areas (108). The spread of malaria in Europe was probably due to the spread of its vector, the Anopheles mosquito (13) (Box 3). This was confirmed by the role played by A. gambiae in Africa for the spread of malaria in that continent (109). In Italy during the Middle Ages, the spread of malaria probably followed the spread of the A. sacharovi mosquito in central and southern Italy (13). In the region of Lugnano, where the presence of malaria in the 5th century was demonstrated by molecular biology (13), the spread of A. labranchiae was considerable in the region during this period, playing the role of vector, later replaced by A. atroparvus in the beginning of the 20th century (13). Anthropological study disclosed traditional Sardinian culture traits limiting exposure to the malaria vector Anopheles labranchiae, recalled that Sardinia was probably the European territory most affected by malaria between the end of the 19th and the beginning of the 20th century (88, 110). An. sacharovi has disappeared in Corsica but An. labranchiae, which is competent in the transmission of P. falciparum, remains currently abundant in the island (103).
Box 3. Malariotherapy.
Chronic syphilitic meningoencephalitis causes a fatal progressive degeneration of the central nervous system known as general paralysis (111, 112). In the absence of any known effective treatment, Julius Wagner-Jauregg first used so-called malariotherapy in Vienna on June 14, 1917 (113). The goal of this therapeutic approach was to provoke a controlled fever to prevent complications from neurosyphilis. At the start of the trials, Wagner used erysipelas, which was a failure (114). He later tried different fever causative agents such as tuberculin, smallpox, and typhoid before opting for P. vivax inoculation, which was judged to be more controllable and curable by quinine administration (115). When Wagner-Jauregg attended a soldier with malaria returning from the Balkans, he saw it as a sign of fate, and after confirming that the soldier's blood was infected with P. vivax, he decided to inoculate it in three patients with general paralysis. These were the first trials of malariotherapy (116). For this work Wagner-Jauregg received the Nobel Prize in Medicine in 1927.
Pyrotherapy was reinforced by Treponema pallidum sensitivity tests to hyperthermia carried out in 1932 by Boak et al., demonstrating that T. pallidum was susceptible to heat above 37.5°C (117). In New York City between 1923 and 1927, Bunker and Kirby, treating general paralysis in a total of 156 patients, reported a complete remission rate of 33 vs. 26% mortality (114). In the absence of standards and controls, the results seemed promising at the time, giving hope for a cure of general paralysis (114). In 1941, after the introduction of penicillin as a treatment, malariotherapy stopped (115). Today, malariotherapy may be seen as horrible and unethical, but at the time, given the desperate cases caused by the neurological form of syphilis, resorting to this therapy seemed to be a godsend.
Blood Groups and Malaria Susceptibility
Several studies have demonstrated a strong possible relationship between different blood groups of the different referenced grouping systems and malaria susceptibility. Epidemiological studies assessing the relationship between ABO blood grouping and malaria susceptibility indicate that individuals with group O are significantly protected against severe P. falciparum malaria (118–120).This is explained by the significant reduction in the cytoadherence of red blood cells, which is responsible for severe malaria syndromes, in individuals with group O compared to individuals with other groups (121, 122). Another grouping system most frequently used in the case of malaria is the Duffy system, and represents the presence or absence of Fy antigens (Fy a and Fy b) on the surface of red blood cells; these antigens are considered receptors for the parasites P. vivax and P. knowlesi. The absence of the Duffy antigen (Fy a - b- phenotype), which is dominant in Africa, confers therefore some resistance against P. vivax and P. knowlesi (123). Finally, another blood grouping system of interest is the Gerbich system, where studies have shown that the rate of individuals positive for P. falciparum and/or P. vivax is more frequently found in Gerbich “Ge-negative” individuals, suggesting that the negativity of the Ge antigen protects against malaria infection and confers some resistance (124). This may be reflected in the fact that the absence of c-glycophorins and thus of proteins 4.1 and P55, which leads to alterations in the skeleton of the erythrocyte membrane, limits the invasion of malaria merozoites (125).
Conclusion
Current paleomicrobiological diagnostic methods are robust enough to support and confirm symptoms suggesting the occurrence of pandemic malaria during different historical periods, as described by ancient texts, using direct detection of infectious agents in ancient specimens; and to enter the differential diagnosis of malaria with mimicking infectious such as among others, pyogenic infections, tuberculosis, schistosomiasis, leptospirosis or borreliosis (126). In Europe, studies based on molecular techniques of malaria detection are still few and mainly concentrated in Italy. However, these studies support the ability to detect ancient malaria DNA in the old World. The development of molecular techniques is necessary to improve parasite detection of Plasmodium genus from human remains. The development of direct detection of ancient malaria would be an excellent way to study the origin, evolution, and frequency of malaria in Europe over the centuries. In this objective, several archaeological sites seem interesting for the study of malaria occurrence based on ancient texts and climatic and environmental parameters, like wet and formerly swampy areas such as Versailles, Corsica, and Sologne in France.
In view of current and future climate changes and variability in the European continent, understanding the evolution of malaria, its Anopheles vectors and the climatic parameters that were conducive to its expansion, is particularly important in understanding host-pathogen interactions, modes of transmission, and frequency of spread. This would provide a good basis, given the possibility of malaria resurgence in a currently malaria-free Europe, for developing a strategy to avoid its resurgence. For instance, competent mosquitos for P. falciparum malaria transmission (An. labranchiae) are still present in Corsica and favorable climate may lead to the possibly re-emergence of malaria in Corsica (103).
Author Contributions
MB, BP, MD, and RB wrote the manuscript. MB and RB designed the figures. All authors contributed to the article and approved the submitted version.
Conflict of Interest
The authors declare that the research was conducted in the absence of any commercial or financial relationships that could be construed as a potential conflict of interest.
References
1. Meibalan E, Marti M. Biology of malaria transmission. Cold Spring Harb Perspect Med. (2017) 7:a025452. doi: 10.1101/cshperspect.a025452
2. World Health Organization. World Malaria Report 2020:20 Years of Global Progress and Challenges. Geneva: World Health Organization (2020). Available online at: https://www.who.int/publications/i/item/9789240015791 (accessed January 4, 2021).
4. Sharp PM, Plenderleith LJ, Hahn BH. Ape origins of human malaria. Annu Rev Microbiol. (2020) 74:39–63. doi: 10.1146/annurev-micro-020518-115628
5. Antinori S, Galimberti L, Milazzo L, Corbellino M. Biology of human malaria plasmodia including Plasmodium knowlesi. Mediterr J Hematol Infect Dis. (2012) 4:201. doi: 10.4084/mjhid.2012.013
6. Poinar G. Plasmodium dominicana n. sp. (Plasmodiidae: Haemospororida) from Tertiary Dominican amber. Syst Parasitol. (2005) 61:47–52. doi: 10.1007/s11230-004-6354-6
7. Liu W, Li Y, Learn GH, Rudicell RS, Robertson JD, Keele BF, et al. Origin of the human malaria parasite Plasmodium falciparum in gorillas. Nature. (2010) 467:420–5. doi: 10.1038/nature09442
8. Liu W, Li Y, Shaw KS, Learn GH, Plenderleith LJ, Malenke JA, et al. African origin of the malaria parasite Plasmodium vivax. Nat Commun. (2014) 5:3346. doi: 10.1038/ncomms4346
9. Pagani L, Lawson DJ, Jagoda E, Mörseburg A, Eriksson A, Mitt M, et al. Genomic analyses inform on migration events during the peopling of Eurasia. Nature. (2016) 538:238–42. doi: 10.1038/nature19792
10. Lee K-S, Divis PCS, Zakaria SK, Matusop A, Julin RA, Conway DJ, et al. Plasmodium knowlesi: reservoir hosts and tracking the emergence in humans and macaques. PLoS Pathog. (2011) 7:e1002015. doi: 10.1371/journal.ppat.1002015
11. Cox FEG. History of human parasitology. Clin Microbiol Rev. (2002) 15:595–612. doi: 10.1128/CMR.15.4.595-612.2002
12. Gelabert P, Olalde I, De-DIos T, Civit S, Lalueza-Fox C. Malaria was a weak selective force in ancient Europeans. Sci Rep. (2017) 7:1377. doi: 10.1038/s41598-017-01534-5
13. Sallares R, Bouwman A, Anderung C. The spread of malaria to Southern Europe in antiquity: new approaches to old problems. Med Hist. (2004) 48:311–28. doi: 10.1017/S0025727300007651
14. Bianucci R, Fornaciari G, Montella A, Bandiera P. Origins of Malaria and leishmaniasis in Sardinia: First Results of a Paleoimmunological Study. (2014). Available online at: http://www.researchgate.net/publication/282658108 (accessed April 30, 2021).
15. Drugs I of M (US) C on the E of A, Arrow KJ, Panosian C, Gelband H. A Brief History of Malaria (2004). Available online at: https://www.ncbi.nlm.nih.gov/books/NBK215638/ (accessed February 25, 2021).
16. Kousoulis AA, Chatzigeorgiou KS, Danis K, Tsoucalas G, Vakalis N, Bonovas S, et al. Malaria in Laconia, Greece, then and now: a 2500-year-old pattern. Int J Infect Dis. (2013) 17:e8–11. doi: 10.1016/j.ijid.2012.09.013
17. Bruce-Chwatt LJ. John macculloch MD FRS. (1773–1835) (the precursor of the discipline of malariology). Med Hist. (1977) 21:156–65. doi: 10.1017/S0025727300037686
18. Petithory J-C. A propos de la découverte de l'hématozoaire du paludisme par A. Laveran Bône 1878-Constantine 1880*. Colombes.
19. Laveran A (1845-1922). A du texte. Nature Parasitaire des Accidents de l'impaludisme : Description d'un nouveau parasite trouvé dans le sang des malades atteints de fièvre palustre / par A. Laveran (1881). Available online at: https://gallica.bnf.fr/ark:/12148/bpt6k9761344p (accessed April 2, 2021).
20. Cox FE. History of the discovery of the malaria parasites and their vectors. Parasites Vectors. (2010) 3:5. doi: 10.1186/1756-3305-3-5
21. Centers for Disease Control and Prevention. CDC - Malaria - About Malaria - History - Laveran and the Discovery of the Malaria Parasite. Atlanta (2017).
22. Manson P. On the nature and significance of the crescentic and flagellated bodies in malar1al blood. Br Med J. (1894) 2:1306–8. doi: 10.1136/bmj.2.1771.1306
23. Grassi B. Studi di uno zoologo sulla malaria / Battista Grassi. 2nd ed. Roma : R. Accademia dei lincei (1901). doi: 10.5962/bhl.title.37999
24. Ross R. On some peculiar pigmented cells found in two mosquitoes fed on malarial blood. Br Med J. (1897) ii:1736–88.
25. Shortt HE, Garnham PCC. Pre-erythrocytic stage in mammalian malaria parasites [1]. Nature. (1948) 161:126. doi: 10.1038/161126a0
28. Schlesinger' PH, Krogstad DJ, Herwaldt BL. MINIREVIEWS Antimalarial Agents: Mechanisms of Action. (1988).
29. Kwiatkowski DP. How malaria has affected the human genome and what human genetics can teach us about malaria. Am J Hum Genet. (2005) 77:171–92. doi: 10.1086/432519
30. Marciniak S, Prowse TL, Herring DA, Klunk J, Kuch M, Duggan AT, et al. Plasmodium falciparum malaria in 1st−2nd century CE southern Italy. Curr Biol. (2016) 26:R1220–2. doi: 10.1016/j.cub.2016.10.016
31. Dioscorides Pedanius, Osbaldeston TA, Wood RPA. De materia medica : being an herbal with many other medicinal materials written in Greek in the first century of the common era. Johannesburg: Ibidis (2000).
32. Green MH, Green MH. Climate and disease in medieval Eurasia. In: Oxford Research Encyclopedia of Asian History. Hoboken, NJ: John Wiley & Sons; Oxford University Press. 251–300.
33. Newfield TP. Malaria and malaria-like disease in the early middle ages. Early Mediev Eur. (2017) 25:251–300. doi: 10.1111/emed.12212
35. Sallares R, Gomzi S. Biomolecular archaeology of malaria. Anc Biomol. (2001) 3:195–213. doi: 10.1093/acprof:oso/9780199248506.001.0001
36. Adams M, Alther W, Kessler M, Kluge M, Hamburger M. Malaria in the renaissance: remedies from European herbals from the 16th and 17th century. J Ethnopharmacol. (2011) 133:278–88. doi: 10.1016/j.jep.2010.10.060
38. Dobson MJ. Malaria in England: a geographical and historical perspective. Parassitologia. (1994) 36:35–60.
39. Reiter P. From Shakespeare to Defoe: Malaria in England in the little ice age. Emerg Infect Dis. (2000) 6:1–11. doi: 10.3201/eid0601.000101
40. Huldén L, Huldén L, Heliövaara K. Malaria Journal Endemic malaria: an “indoor” disease in northern Europe. historical data analysed. Malaria J. (2005) 4:19. doi: 10.1186/1475-2875-4-19
41. Tognotti E. Program to eradicate malaria in Sardinia, 1946-1950. Emerg Infect Dis. (2009) 15:1460–6. doi: 10.3201/eid1509.081317
42. Rickard Christophers S. Malaria in war. Trans R Soc Trop Med Hyg. (1939) 33:277–92. doi: 10.1016/S0035-9203(39)90190-2
45. Meshnick SR, Dobson MJ. The history of antimalarial drugs. Antimalarial Chemother. (2001) 1:15–25. doi: 10.1385/1-59259-111-6:15
46. Dock G. Annals of Medical History: Robert Talbor, Madame de Sévigné, and the Introduction of Cinchona: An Episode Illustrating the Influence of Women in Medicine. New York, NY: Paul B. Hoeber, Inc. (1927).
47. The English Remedy Or Talbor's Wonderful Secret for Cureing Agues and - Sir Robert TALBOR - Google Livres. Available online at: https://books.google.fr/books?id=7DJkAAAAcAAJ&printsec=frontcover&hl=fr&source=gbs_ge_summary_r&cad=0#v=onepage&q&f=false (accessed March 31, 2021).
48. Pai-Dhungat JV. Caventou, pelletier & - history of quinine. J Assoc Physicians India. (2015) 63:58.
49. Brabin BJ. Malaria's contribution to World War One - the unexpected adversary. Malar J. (2014) 13:497. doi: 10.1186/1475-2875-13-497
51. Bertrand PA. Fievres Intermittentes Et Quinquina A La Cour De Louis Xiv. (1999). Available online at: http://www.ac-sciences-lettres-montpellier.fr/1Seancedulundi12/04/ (accessed January 11, 2021).
53. Stanis Perez. Louis XIV et le quinquina. (2003). Available online at: https://cour-de-france.fr/vie-quotidienne/medecine-sciences-et-savoirs/etudes-modernes/article/louis-xiv-et-le-quinquina (accessed March 31, 2021).
54. Raoult D, Drancourt M. Paleomicrobiology: Past Human Infections. Berlin Heidelberg: Springer. (2008) doi: 10.1007/978-3-540-75855-6
55. Bos KI, Kühnert D, Herbig A, Esquivel-Gomez LR, Andrades Valtueña A, Barquera R, et al. Paleomicrobiology: diagnosis and evolution of ancient pathogens. Annu Rev Microbiol. (2019) 73:639–66. doi: 10.1146/annurev-micro-090817-062436
56. Spigelman M, Lemma E. The use of the polymerase chain reaction (PCR) to detectMycobacterium tuberculosis in ancient skeletons. Int J Osteoarchaeol. (1993) 3:137–43. doi: 10.1002/oa.1390030211
57. Drancourt M, Raoult D. Palaeomicrobiology: current issues and perspectives. Nat Rev Microbiol. (2005) 3:23–35. doi: 10.1038/nrmicro1063
58. van der Valk T, Pečnerová P, Díez-del-Molino D, Bergström A, Oppenheimer J, Hartmann S, et al. Million-year-old DNA sheds light on the genomic history of mammoths. Nature. (2021) 591:265–9. doi: 10.1038/s41586-021-03224-9
59. Allentoft ME, Collins M, Harker D, Haile J, Oskam CL, Hale ML, et al. The half-life of DNA in bone: measuring decay kinetics in 158 dated fossils. Proc R Soc B Biol Sci. (2012) 279:4724–33. doi: 10.1098/rspb.2012.1745
60. Willerslev E, Cappellini E, Boomsma W, Nielsen R, Hebsgaard MB, Brand TB, et al. Ancient biomolecules from deep ice cores reveal a forested southern Greenland. Science. (2007) 317:111–4. doi: 10.1126/science.1141758
61. Arning N, Wilson DJ. The past, present and future of ancient bacterial DNA. Microb Genomics. (2020) 6:1–19. doi: 10.1099/mgen.0.000384
62. Namouchi A, Guellil M, Kersten O, Hänsch S, Ottoni C, Schmid BV, et al. Integrative approach using Yersinia pestis genomes to revisit the historical landscape of plague during the Medieval Period. Proc Natl Acad Sci USA. (2018) 115:E11790–7. doi: 10.1073/pnas.1812865115
63. Bos KI, Schuenemann VJ, Golding GB, Burbano HA, Waglechner N, Coombes BK, et al. A draft genome of Yersinia pestis from victims of the Black Death. Nature. (2011) 478:506–10. doi: 10.1038/nature10549
64. Dabney J, Meyer M, Pääbo S. Ancient DNA damage. Cold Spring Harb Perspect Biol. (2013) 5:a012567. doi: 10.1101/cshperspect.a012567
65. Spyrou MA, Bos KI, Herbig A, Krause J. Ancient pathogen genomics as an emerging tool for infectious disease research. Nat Rev Genet. (2019) 20:323–40. doi: 10.1038/s41576-019-0119-1
66. Sawyer S, Krause J, Guschanski K, Savolainen V, Pääbo S. Temporal patterns of nucleotide misincorporations and DNA fragmentation in ancient DNA. PLoS ONE. (2012) 7:e34131. doi: 10.1371/journal.pone.0034131
67. Miller RL, Ikram S, Armelagos GJ, Walker R, Harer WB, Shiff CJ, et al. Diagnosis of Plasmodium falciparum infections in mummies using the rapid manual ParaSightTM-F test. Trans R Soc Trop Med Hyg. (1994) 88:31–2. doi: 10.1016/0035-9203(94)90484-7
68. Massa ER, Ceruttr N, Savoia AMD. Malaria in ancient Egypt: Paleoimmunological investigation on predynastic mummified remains. Chungara. (2000) 32:7–9. doi: 10.4067/S0717-73562000000100003
69. Bianucci R, Mattutino G, Lallo R, Charlier P, Jouin-Spriet H, Peluso A, et al. Immunological evidence of Plasmodium falciparum infection in an Egyptian child mummy from the Early Dynastic Period. J Archaeol Sci. (2008) 35:1880–85. doi: 10.1016/j.jas.2007.11.019
70. Fornaciari G, Giuffra V, Ferroglio E, Gino S, Bianucci R. Plasmodium falciparum immunodetection in bone remains of members of the Renaissance Medici family (Florence, Italy, sixteenth century). Trans R Soc Trop Med Hyg. (2010) 104:583–7. doi: 10.1016/j.trstmh.2010.06.007
71. Taylor G, Rutland P, Molleson T. A sensitive polymerase chain reaction method for the detection of plasmodium species DNA in ancient human remains. Anc Biomol (1997) 1:193–203.
72. Nerlich AG, Schraut B, Dittrich S, Jelinek T, Zink AR. Plasmodium falciparum in ancient Egypt. Emerg Infect Dis. (2008) 14:1317–9. doi: 10.3201/eid1408.080235
73. Bianucci R, Araujo A, Pusch CM, Nerlich AG. The identification of malaria in paleopathology-An in-depth assessment of the strategies to detect malaria in ancient remains. Acta Trop. (2015) 152:176–80. doi: 10.1016/j.actatropica.2015.09.002
74. van Dorp L, Gelabert P, Rieux A, de Manuel M, de-Dios T, Gopalakrishnan S, et al. Plasmodium vivax malaria viewed through the lens of an eradicated european strain. Mol Biol Evol. (2020) 37:773–85. doi: 10.1093/molbev/msz264
75. Hawass Z, Gad YZ, Ismail S, Khairat R, Fathalla D, Hasan N, et al. Ancestry and pathology in King Tutankhamun's family. J Am Med Assoc. (2010) 303:638–47. doi: 10.1001/jama.2010.121
76. Lalremruata A, Ball M, Bianucci R, Welte B, Nerlich AG, Kun JFJ, et al. Molecular identification of falciparum malaria and human tuberculosis co-infections in mummies from the fayum depression (Lower Egypt). PLoS ONE. (2013) 8:e60307. doi: 10.1371/journal.pone.0060307
77. Khairat R, Ball M, Chang CCH, Bianucci R, Nerlich AG, Trautmann M, et al. First insights into the metagenome of Egyptian mummies using next-generation sequencing. J Appl Genet. (2013) 54:309–325. doi: 10.1007/s13353-013-0145-1
78. Grobusch MP, Jelinek T, Hänscheid T. False positivity of rapid antigen detection tests for diagnosis of Plasmodium falciparum malaria: issue appears to be more complicated than presented. J Clin Microbiol. (1999) 37:3781–2. doi: 10.1128/JCM.37.11.3781-3782.1999
79. Laferi H, Kandel K, Pichler H. False positive dipstick test for malaria. N Engl J Med. (1997) 337:1635–6. doi: 10.1056/NEJM199711273372219
80. Gowland RL, Western AG. Morbidity in the marshes: using spatial epidemiology to investigate skeletal evidence for malaria in Anglo-Saxon England (AD 410-1050). Am J Phys Anthropol. (2012) 147:301–11. doi: 10.1002/ajpa.21648
81. Smith-Guzmán NE. Cribra orbitalia in the ancient Nile Valley and its connection to malaria. Int J Paleopathol. (2015) 10:1–12. doi: 10.1016/j.ijpp.2015.03.001
82. Keenleyside A, Panayotova K. Cribra orbitalia and porotic hyperostosis in a Greek colonial population (5th to 3rd centuries BC) from the Black Sea. Int J Osteoarchaeol. (2006) 16:373–84. doi: 10.1002/oa.831
83. Facchini F, Rastelli E, Brasili P. Cribra orbitalia and cribra cranii in Roman skeletal remains from the Ravenna area and Rimini(I–IV century AD). Int J Osteoarchaeol. (2004) 14:126–36. doi: 10.1002/oa.717
84. Tognotti E, Montella A, Brown PJ, Bandiera P. New osteological data on malaria in sardinia from antiquity to the modern era. Adv Infect Dis. (2017) 7:37–44. doi: 10.4236/aid.2017.72005
85. Angel JL. Porotic hyperostosis, anemias, malarias, and marshes in the prehistoric Eastern Mediterranean. Science. (1966) 153:760–3. doi: 10.1126/science.153.3737.760
86. Walker PL, Bathurst RR, Richman R, Gjerdrum T, Andrushko VA. The causes of porotic hyperostosis and cribra orbitalia: a reappraisal of the iron-deficiency-anemia hypothesis. Am J Phys Anthropol. (2009) 139:109–25. doi: 10.1002/ajpa.21031
87. Cole G, Waldron T. Cribra orbitalia: dissecting an ill-defined phenomenon. Int J Osteoarchaeol. (2019) 29:oa.2757. doi: 10.1002/oa.2757
88. Brown PJ. Cultural adaptations to endemic malaria in Sardinia. Med Anthropol. (1981) 5:313–39. doi: 10.1080/01459740.1981.9986991
89. Flint J, Harding RM, Boyce AJ, Clegg JB. The population genetics of the haemoglobinopathies. Baillieres Clin Haematol. (1998) 11:1–51. doi: 10.1016/S0950-3536(98)80069-3
90. De Sanctis V, Kattamis C, Canatan D, Soliman AT, Elsedfy H, Karimi M, et al. β-thalassemia distribution in the old world: An ancient disease seen from a historical standpoint. Mediterr J Hematol Infect Dis. (2017) 9:e2017018. doi: 10.4084/mjhid.2017.018
91. Viganó C, Haas C, Rühli FJ, Bouwman A. 2,000 Year old β-thalassemia case in Sardinia suggests malaria was endemic by the Roman period. Am J Phys Anthropol. (2017) 164:362–70. doi: 10.1002/ajpa.23278
92. Gandhi M. Complement receptor 1 and the molecular pathogenesis of malaria. Indian J Hum Genet. (2007) 13:39–47. doi: 10.4103/0971-6866.34704
93. Kosoy R, Ransom M, Chen H, Marconi M, MacCiardi F, Glorioso N, et al. Evidence for malaria selection of a CR1 haplotype in Sardinia. Genes Immun. (2011) 12:582–8. doi: 10.1038/gene.2011.33
94. Contu L, Carcassi C, Orrù S, Mulargia M, Arras M, Boero R, et al. HLA-B35 frequency variations correlate with malaria infection in Sardinia. Tissue Antigens. (1998) 52:452–61. doi: 10.1111/j.1399-0039.1998.tb03072.x
95. texte FA du. Code des dessèchemens, ou Recueil des règlemens rendus sur cette matière depuis le règne d'Henry IV jusqu'à nos jours; suivi d'un commentaire sur la loi du 16 septembre 1807 et d'un tableau général des marais du royaume. (1817). Available online at: https://gallica.bnf.fr/ark:/12148/bpt6k5749713m (accessed February 4, 2021).
96. Derex JM. Pour une histoire des zones humides en France (XVIIe-XIX e siècle): Des paysages oubliés, une histoire à écrire. Hist Soc Rural. (2001) 15:11–36. doi: 10.3917/hsr.015.36
97. Kuhn KG, Campbell-Lendrum DH, Armstrong B, Davies CR. Malaria in Britain: Past, present, and future. Proc Natl Acad Sci USA. (2003) 100:9997 LP−10001. doi: 10.1073/pnas.1233687100
98. Majori G. Short history of malaria and its eradication in Italy with short notes on the fight against the infection in the Mediterranean basin. Mediterr J Hematol Infect Dis. (2012) 4:201. doi: 10.4084/mjhid.2012.016
99. Majori G. The long road to malaria eradication. Lancet. (1999) 354:SIV31. doi: 10.1016/S0140-6736(99)90374-4
100. Toumanoff C. Contribution à létude de lanophélisme et du paludisme en Corse. Inst. Nat. Hygiène. (1954). Available online at: https://scholar.google.com/scholar?q=Contribution à létude de lanophélisme et du paludisme en Corse (accessed April 2, 2021).
101. Ambroise T, Pat MQ, PR. Reapparition Du Paludisme En Corse. Interet du depistage seroepidemiologique. Reapparition du palud en corse interet du depist seroepidemiologique (1972).
102. Armengaud A, Legros F, Quatresous I, Barre H, Valayer P, Fanton Y, et al. A case of autochthonous Plasmodium vivax malaria, Corsica, August 2006. Wkly Releases. (2006) 11:3081. doi: 10.2807/esw.11.46.03081-en
103. Toty C, Barré H, Le Goff G, Larget-Thiéry I, Rahola N, Couret D, et al. Malaria risk in Corsica, former hot spot of malaria in France. Malar J. (2010) 9:1–8. doi: 10.1186/1475-2875-9-231
105. Meigen JW, Meigen JW. Systematische Beschreibung der bekannten europäischen zweiflügeligen Insekten. Aachen : Bei Friedrich Wilhelm Forstmann : Gedrukt bei Beaufort Sohn. (1818). Available online at: https://www.biodiversitylibrary.org/item/45833 (accessed March 2, 2021). doi: 10.5962/bhl.title.13731
106. Trájer A, Padisák J. Exploration of the main types of biome-scale culicid entomofauna (Diptera: Culicidae) in Europe and its relationship to the occurrence of mosquito-borne arboviruses. Acta Zool Acad Sci Hungaricae. (2019) 65:299–322. doi: 10.17109/AZH.65.3.299.2019
107. Trájer AJ. The potential persistence of ancient malaria through the Quaternary period in Europe. Quat Int. (2021) 586:1–13. doi: 10.1016/j.quaint.2021.02.014
108. Bueno-Marí R, Jiménez-Peydró R. Anopheles plumbeus Stephens, 1828: a neglected malaria vector in Europe. Malar Rep. (2011) 1:2. doi: 10.4081/malaria.2011.e2
109. Coluzzi M. The clay feet of the malaria giant and its African roots: hypotheses and inferences about origin, spread and control of Plasmodium falciparum. Parassitologia. (1999) 41:277–83.
111. Daey Ouwens IM, Lens CE, Fiolet ATL, Ott A, Koehler PJ, Kager PA, et al. Malaria fever therapy for general paralysis of the insane: a historical cohort study. Eur Neurol. (2017) 78:56–62. doi: 10.1159/000477900
112. Mickle WJ. General paralysis of the insane. Am J Psychiatry. (1880) 37:103–9. doi: 10.1176/ajp.37.1.103
113. Waddington K, Thomas R, Willis M. General paralysis of the insane. Pract Neurol. (2011) 11:366–9. doi: 10.1136/practneurol-2011-000112
114. Austin SC, Stolley PD, Lasky T. The history of malariotherapy for neurosyphilis: modern parallels. J Am Med Assoc. (1992) 268:516–9. doi: 10.1001/jama.268.4.516
115. Raju TNK. Hot brains: manipulating body heat to save the brain. Pediatrics. (2006) 117:e320–e1. doi: 10.1542/peds.2005-1934
116. Wagner-Jauregg J. The history of the malaria treatment of general paralysis. Am J Psychiatry. (1946) 102:577–82. doi: 10.1176/ajp.102.5.577
117. Boak RA, Carpenter CM, Warren Sl. Studies on the physiological effects of fever temperatures : iii. the thermal death time of treponema pallidum in vitro with special reference to fever temperatures. J Exp Med. (1932) 56:741–50. doi: 10.1084/jem.56.5.741
118. Lell B, May J, Schmidt-Ott RJ, Lehman LG, Luckner D, Greve B, et al. The role of red blood cell polymorphisms in resistance and susceptibility to malaria. Clin Infect Dis. (1999) 28:794–9. doi: 10.1086/515193
119. Rowe A, Obeiro J, Newbold CI, Marsh K. Plasmodium falciparum rosetting is associated with malaria severity in Kenya. Infect Immun. (1995) 63:2323–6. doi: 10.1128/IAI.63.6.2323-2326.1995
120. Pathirana SL, Alles HK, Bandara S, Phone-Kyaw M, Perera MK, Wickremasinghe AR, et al. ABO-blood-group types and protection against severe, Plasmodium falciparum malaria. Ann Trop Med Parasitol. (2005) 99:119–24. doi: 10.1179/136485905X19946
121. Williams TN. Human red blood cell polymorphisms and malaria. Curr Opin Microbiol. (2006) 9:388–94. doi: 10.1016/j.mib.2006.06.009
122. Ho M, Hickey MJ, Murray AG, Andonegui G, Kubes P. Visualization of Plasmodium falciparum- endothelium interactions in human microvasculature: mimicry of leukocyte recruitment. J Exp Med. (2000) 192:1205–11. doi: 10.1084/jem.192.8.1205
123. Miller LH, Mason SJ, Clyde DF, Mcginniss MH. The Resistance factor to Plasmodium vivax in blacks: the duffy-blood-group genotype, FyFy. N Engl J Med. (1976) 295:302–4. doi: 10.1056/NEJM197608052950602
124. Serjeantson SW. A selective advantage for the Gerbich-negative phenotype in malarious areas of Papua New Guinea. P N G Med J. (1989) 32:5–9.
125. Chishti AH, Palek J, Fisher D, Maalouf GJ, Liu SC. Reduced invasion and growth of Plasmodium falciparum into elliptocytic red blood cells with a combined deficiency of protein 4.1, glycophorin C, and p55. Blood. (1996) 87:3462–9. doi: 10.1182/blood.V87.8.3462.bloodjournal8783462
Keywords: Malaria, Plasmodium, intermittent fever, European, paleopathology, paleomicrobiology, quinquina, quinine
Citation: Boualam MA, Pradines B, Drancourt M and Barbieri R (2021) Malaria in Europe: A Historical Perspective. Front. Med. 8:691095. doi: 10.3389/fmed.2021.691095
Received: 05 April 2021; Accepted: 20 May 2021;
Published: 30 June 2021.
Edited by:
Andreas Nerlich, Klinikum Bogenhausen, GermanyReviewed by:
Helen Donoghue, University College London, United KingdomTeddi Setzer, Oakland University, United States
Copyright © 2021 Boualam, Pradines, Drancourt and Barbieri. This is an open-access article distributed under the terms of the Creative Commons Attribution License (CC BY). The use, distribution or reproduction in other forums is permitted, provided the original author(s) and the copyright owner(s) are credited and that the original publication in this journal is cited, in accordance with accepted academic practice. No use, distribution or reproduction is permitted which does not comply with these terms.
*Correspondence: Rémi Barbieri, remibarbieri@hotmail.fr