- 1Department of Bioengineering, Denver, Anschutz Medical Campus, University of Colorado, Aurora, CO, United States
- 2Division of Pulmonary, Critical Care and Sleep Medicine, Department of Medicine, Hastings Center for Pulmonary Research, University of Southern California, Los Angeles, CA, United States
- 3Department of Stem Cell Biology and Regenerative Medicine, University of Southern California, Los Angeles, CA, United States
- 4Department of Pediatrics, Anschutz Medical Campus, University of Colorado, Aurora, CO, United States
- 5Division of Pulmonary Sciences and Critical Care Medicine, Department of Medicine, Anschutz Medical Campus, University of Colorado, Aurora, CO, United States
Biomaterials intentionally designed to support the expansion, differentiation, and three-dimensional (3D) culture of induced-pluripotent stem cells (iPSCs) may pave the way to cell-based therapies for chronic respiratory diseases. These conditions are endured by millions of people worldwide and represent a significant cause of morbidity and mortality. Currently, there are no effective treatments for the majority of advanced lung diseases and lung transplantation remains the only hope for many chronically ill patients. Key opinion leaders speculate that the novel coronavirus, COVID-19, may lead to long-term lung damage, further exacerbating the need for regenerative therapies. New strategies for regenerative cell-based therapies harness the differentiation capability of human iPSCs for studying pulmonary disease pathogenesis and treatment. Excitingly, biomaterials are a cell culture platform that can be precisely designed to direct stem cell differentiation. Here, we present a closer look at the state-of-the-art of iPSC differentiation for pulmonary engineering, offer evidence supporting the power of biomaterials to improve stem cell differentiation, and discuss our perspective on the potential for tissue-informed biomaterials to transform pulmonary regenerative medicine.
Introduction
Chronic respiratory diseases are the third leading cause of global morbidity and mortality, impacting an astonishing 7.4% of the world's population (1). Despite progress in therapeutic development for these conditions, current treatments merely control symptoms and exacerbations. The urgency for new treatment options cannot be underestimated as escalating urban environmental risk factors and tobacco use, have caused a substantial increase in the exacerbation and mortality rates of chronic lower respiratory diseases (2). This growth is exemplified by the 39.8% increase chronic respiratory disease cases since 1990, which includes a 3.9% global increase in chronic obstructive pulmonary disease (COPD) and a 3.6% increase in asthma (2). Increased air pollution exposure also heightens the risk for exacerbations in COPD and idiopathic pulmonary fibrosis (IPF) (3, 4). Furthermore, researchers have projected that survivors of severe acute respiratory syndrome coronavirus 2 (SARS-CoV-2), responsible for the recent global pandemic, will have an increased prevalence of chronic respiratory conditions due to severe lung damage caused by acute respiratory distress syndrome (ARDS) (5). Collectively, airway damage and scarring of gas-exchange surfaces in the lungs will require innovative therapeutic strategies for airway regeneration. Nevertheless, progression of new therapeutic approaches for these diseases is hindered by a lack of reproducible in vitro models that closely reflect in vivo physiology.
Respiratory diseases present with significant heterogeneity among patients, creating considerable variability in disease onset, severity, and progression (6). Patient-specific regenerative cell-based therapies provide an attractive avenue to study pulmonary disease pathology and evaluate effective treatment regimes. However, progress is currently limited by materials that are not conducive to optimization through controlled modification. To support the complex cell culture processes required to build these models, we present here the perspective that precisely engineered biomaterials will increase the reproducibility and efficiency of patient-derived induced pluripotent stem cells (iPSCs) differentiation, facilitating the fabrication of three-dimensional (3D), patient-specific models of pulmonary regeneration and disease. Figure 1 depicts a long-term vision for how tissue-informed biomaterials can improve pulmonary regenerative medicine and discovery of new therapeutic targets. First, patient-specific cells can be isolated, expanded, reprogrammed into pluripotent stem cells, and differentiated into mature lung cells using engineered biomaterial cell culture platforms. Biomaterial microenvironments can be tailored to differentiate cells to either healthy lung cells/tissues for transplantation, or diseased lung phenotypes for modeling and evaluating the impact of treatments on chronic respiratory conditions. We predict that this versatility will enable researchers to tissue-informed biomaterials and patient-derived cells to address the unique challenges of each individual respiratory disease.
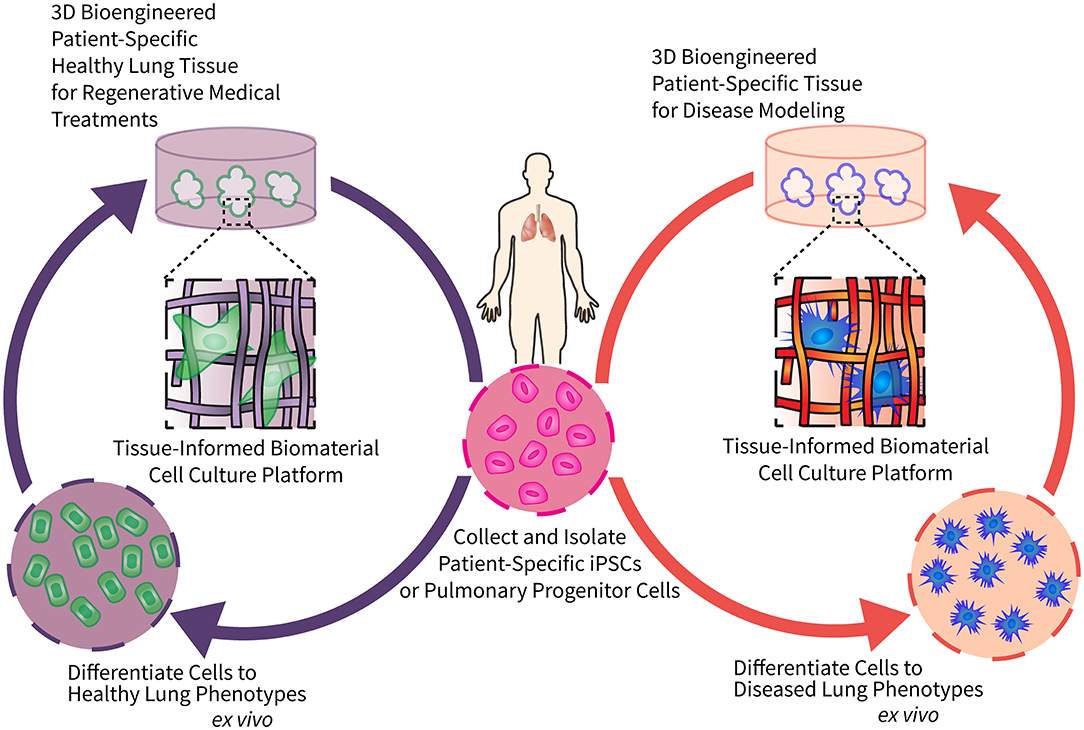
Figure 1. Engineering tissue-informed biomaterials to advance pulmonary regenerative medicine and model respiratory disease. This schematic illustrates the potential for tissue-informed biomaterials to advance pulmonary regenerative medicine through two complementary pathways: (1) by using tissue-informed biomaterial cell culture platforms to differentiate patient-specific stem cells into healthy, implantable lung cells and tissue (left); and (2) engineering biomaterial-based platforms to induce differentiation of these same patient-specific stem cells into diseased phenotypes for disease modeling and evaluation of precision medical treatments (right).
Current Strategies for Modeling Human Lung Development and Regeneration
Animal Models for Studying Respiratory Disease
Animal models are widely used to study lung development and disease pathology. Murine models, for example, have been fundamental in examining physiological processes such as branching morphogenesis, which develops lung architecture and tubular structures (7). Nevertheless, there are discrepancies between rodent and human lung development, physiology, and pathophysiology which have led to numerous successful preclinical animal therapeutic successes that later fail in human clinical trials (8). Murine models, although capable of developing some lung disease phenotypes, such as goblet cell hyperplasia and airway mucus obstruction in COPD, do not develop spontaneous bacterial infections or have equivalent levels of disease severity (9). One example of the limitations of rodent models are those created to study cystic fibrosis (CF), a respiratory disease caused by mutations within the cystic fibrosis transmembrane conductance regulator (CFTR) gene, resulting in the inability to effectively transport chloride across the cell membrane and the accumulation of thick mucus that obstructs airways. In these models, mice generally present a less-severe lung phenotype due to activity of alternative chloride channels that can mitigate the burden of mutated CFTR (10). A transition to large-animal model systems, specifically ferrets and pigs, has improved recapitulation of human lung disease (11, 12). Pigs and ferrets more closely resemble human lung anatomy and morphology, with airways that exhibit similar bacteria and immune cell infiltration (13, 14). Moreover, they are capable of developing spontaneous lung disease and similar lung pathologies such as airway obstruction, inflammation, and mucus buildup to humans (12, 14). While these models have been beneficial in studying disease onset and pathogenesis, they are costly and not ideal for high-throughput therapeutic studies or screening.
Patient-Derived Cells for Studying Respiratory Disease
Patient-derived primary lung epithelial cells have been used as an in vitro alternative to animal models due to their ability to replicate in vivo cell morphology, physiology, and functionality (15, 16). Significant advances have been made in the development of procedures for procuring lung tissue explants and bronchoscopy samples, isolating human airway epithelial cells (HAECs), and optimizing media and culture conditions for their expansion. The cell types that comprise the airway epithelium are summarized in Table 1. Protocols, such as those developed by (32), support the growth and mucociliary differentiation of HAECs at the air-liquid interface (ALI) (33). Similar methods have grown patient primary epithelial cells for therapeutic testing. For example, Neuberger et al., isolated human bronchial epithelial cells (HBECs) from CF patients to perform preclinical tests for CFTR modulators (15). Nevertheless, there are many challenges to working with primary HAECs, including limited access to patient samples, particularly those with rare diseases and genotypes. Furthermore, in vitro expansion of primary cells is limited due to decreased proliferation over time, changes in morphology, and loss of multipotency of the predominant airway epithelium progenitor cell, the basal cell, which has the capacity to regenerate the airway epithelium of the trachea to the bronchioles (34, 35).
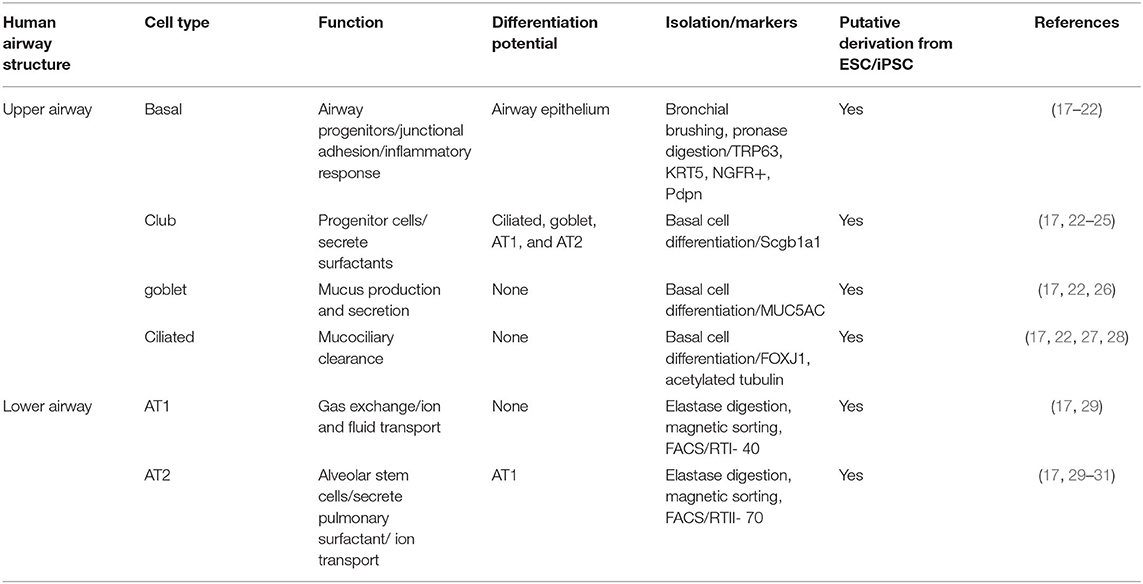
Table 1. Summary of human airway structure, cellular composition, cellular characteristics, differentiation potential, and derivation from embryonic stem cells (ESC) and induced pluripotent stem cells (iPSC).
The invention of iPSCs in 2007 created an alternative approach to obtaining patient-specific cells capable of self-renewal, large-scale expansion, and multilineage differentiation (36–38). Investigation of effective methods to differentiate human iPSCs into mature airway epithelium has been crucial to developing functional cells for effective disease modeling and therapeutic screening. iPSCs have been directed to mimic embryonic lung development and differentiate into functional airway epithelium (17, 18, 27, 39, 40) through sequential addition of factors that regulate activin/nodal, bone morphogenic protein (BMP), fibroblast growth factor (FGF), transforming growth factor-beta (TGFβ), wingless (Wnt) and sonic hedgehog (Shh) signaling (Table 1). These strategic methods of differentiation to lung cell lineages have primarily focused on two-dimensional (2D) monolayer cultures and have varying levels of efficiency based on induction of NK2 homeobox 1 (NKX2.1) expressing primordial lung progenitor cells. Currently, 2D systems are not capable of proper spatial tissue organization and epithelial-mesenchymal associations (41).
Cell culture substrates with physiological mechanical properties and 3D architecture may improve differentiation efficiency and the formation of mature cells from stem or progenitor cells that are more similar to their primary cell counterparts in gene expression and DNA methylation profiles, with studies having largely focused on natural materials, such as basement membrane extracts (41), decellularized precision-cut lung slices (42), and hydrogels derived from extracellular matrix (ECM) (43) to differentiate human lung progenitor cells and evaluate the resulting cellular structure and function. A summary of lung ECM components, cellular binding regions, and common strategies for incorporating these biochemical cues into biomaterials can be found in Table 2.
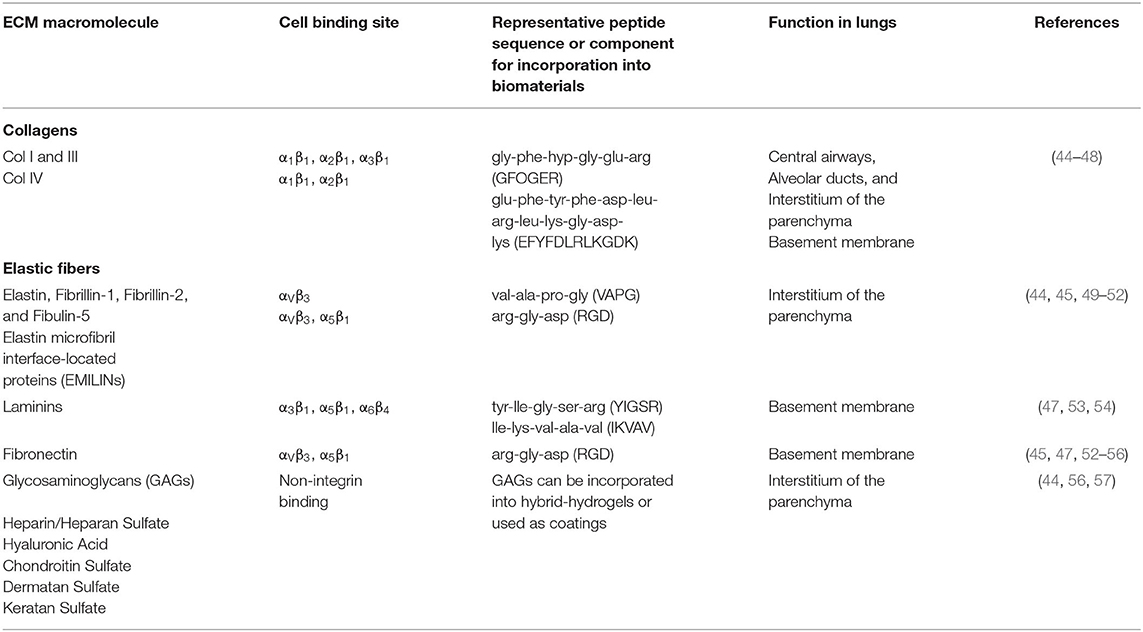
Table 2. Introduction to common ECM components found in the lung, cell binding sites, and common practices for incorporation into biomaterials.
An investigation by Young et al. compared the epithelial barrier function of human basal epithelial stem cells cultured on combinations of a variety of proteins, including collagen I, fibronectin, laminin, and decellularized extracellular matrix (dECM). The combination that produced the highest barrier function as measured by trans-epithelial electrical resistance (TEER) was dECM supplemented with laminin (58). In another study by Greaney et al. human basal progenitor cells were seeded and epithelial differentiation was compared on various platforms including sections of decellularized lung tissue from trachea and distal lung, Matrigel, and traditional ALI culture (59). The study found that cells seeded on sections of decellularized lung tissue sections exhibited regionally specific indicators of epithelial regeneration such as detection of relevant airway epithelial cell markers cytokeratin 5 (KRT5, basal cells), mucin 5AC (MUC5AC, goblet cells), and acetylated alpha tubulin (ATUB, ciliated cells) on decellularized tracheal sections using single cell RNA sequencing (scRNA-seq). These 3D culture systems outperformed 2D culture on basement membrane extracts (Matrigel) and traditional ALI culture. Another recent study investigated seeding of primary human epithelial progenitor cells into hybrid bioinks composed of alginate reinforced with dECM. This hybrid approach allowed for the creation of bioinks with higher viscosities at low shear rates when compared to normal alginate. Epithelial progenitor cells were seeded into this hybrid bioink and 3D printed as a hollow tube and then subjected to ALI differentiation for 28 days. The study found that the hybrid bioink allowed for the differentiation of progenitor cells into ATUB-expressing ciliated cells and that the constructs remained stable and patent for the full 28 days (60). In contrast, culturing human lung organoids (HLOs) derived from pluripotent stem cells within Matrigel enabled cells to spontaneously form physiologically elaborate 3D structures in vitro. These cultures were composed of both epithelial and mesenchymal lineages that corresponded to the cellular composition and structural attributes of the human fetal lung. The approach by Dye et al. produced 3D structures resembling bronchi and bronchioles using HLOs derived from human pluripotent stem cells. However, in a comparable study carried out by Goetzke et al., significant differences in the epigenetic regulation of the cells were observed in iPSCs differentiated on 2D fibrin-based hydrogels compared to 3D culture in the same material. In fact, iPSCs differentiated into induced mesenchymal stem cells (iMSCs) in the 2D condition and were a closer match to primary MSC than the 3D differentiated cells. The cells differentiated in 3D had a notable upregulation of genes related to the cardiovascular system and neurogenesis (61). These results indicate that even though 3D systems are more physiologically relevant and represent an increased complexity compared to 2D systems, these platforms still need further optimization to be more physiologically precise.
Limitations of Current Substrates for iPSC Differentiation
Currently, iPSC differentiation protocols rely extensively on natural, xenogenic materials such as Geltrex and Matrigel, which are basement membrane extracts rich in laminin-111, collagen IV, entactin, and perlecan (55). These extracts act as a substrate for cellular adhesion and present biological moieties that influence cell growth and differentiation at an epigenetic level (62). Unfortunately, these materials also exhibit major translational limitations. Geltrex and Matrigel are both derived from murine tumor tissue, resulting in a high potential for immunogenicity and poorly defined composition with reports of batch-to-batch variability from the manufacturers (63). This heterogeneity contributes to lower differentiation efficiency and limits scalability in future drug development work. Most importantly, these materials lack the capacity for tunability, or customization, required to optimize the substrates for reproducible and efficient iPSC to lung progenitor cell differentiation (64). Synthetic biomaterials designed using tissue-informed engineering strategies can overcome the limitations of traditional materials such as Matrigel and increase the efficiency of differentiating iPSCs into mature cells for further study (30, 65). Specifically, the following section highlights the implementation of tissue-informed hydrogels to support iPSC differentiation protocols for pulmonary regenerative medicine.
Opportunities for Tissue-Informed Biomaterials to Advance Pulmonary Regenerative Medicine
Tissue-informed engineering strategies are a bottom-up approach to engineering materials meant to elicit specific cellular responses (66). First, key facets of tissues are characterized, including the extracellular matrix structure, mechanics, and composition. Next, the intrinsic and extrinsic properties of biomaterials are specifically engineered to replicate the tissues that support cellular expansion, differentiation, and maintenance within a 3D tissue-like architecture. Hydrogels, such as poly(ethylene glycol) PEG, have emerged as a promising candidate for the tissue-informed engineering process. Hydrogels are a single molecule network composed of cross-linked polymer chains. This cross-linking confers an advantage to hydrogels: the ability to swell in water without dissolving allows these materials to closely mimic the mechanical properties and water content of human tissue. Hydrogels have intrinsic and extrinsic properties that can be engineered and optimized using an iterative design process to achieve cellular responses appropriate for each application (67, 68). Intrinsic properties include stiffness (elastic modulus), degradability, and viscoelasticity. Extrinsic properties include dimensionality, topography, and presentation of biomolecules (69).
Tuning the intrinsic and extrinsic properties of these materials takes various forms, ranging from optimizing a static 2D hydrogel cell culture substrate to designing a stimuli-responsive, 3D material system that can be altered by user-controlled inputs or endogenous signals from embedded cells (70, 71). The elastic modulus (E) or stiffness of the hydrogel microenvironment is one physical (intrinsic) property that has been modified by exploiting user-controlled stimuli including light, temperature, or even ultrasound (71). A photodegradable PEG-based crosslinker developed by the Anseth research group, for instance, facilitated dynamic hydrogel softening from E > 30 kPa to E <3 kPa upon exposure to ultraviolet (UV) light (72, 73). This material was used to study gastrointestinal crypt formation by iPSCs embedded in 3D PEG hydrogels compared to 3D Matrigel constructs. The study found that crypt formation, size, and number per colony were functions of matrix softening. It also showed that colony survival was dependent on elastic modulus, with the greatest survival occurring in matrices with a modulus of 1.3 kPa (74). Similarly, one extrinsic property to leverage when designing synthetic materials is the incorporation and release of biological molecules with spatial and temporal control. These moieties include but are not limited to growth factors (75), peptides (76), protein fragments (77), and small molecules (78). For example, Ovadia et al. fabricated PEG-norbornene hydrogel matrices crosslinked with a cell-degradable peptide that presented pendant peptides inspired by proteins and integrins found in Matrigel to cells grown within these constructs. This research found that certain peptide combinations, specifically YIGSR (mimicking laminin) and PHSRNG10RGDS (replicating fibronectin-binding sites) enhanced viability of iPSCs and allowed for differentiation into neural progenitor cells (NPCs) when cultured in 3D over 1 week (79). Another study by Lam et al. used a design of experiments approach to optimize peptide concentrations in engineered biomaterials to maximize the differentiation of iPSCs to NPCs (67). These examples highlight significant progress in the differentiation of iPSCs using biomaterials for non-pulmonary engineering applications.
Tuning the intrinsic and extrinsic properties of biomaterials using a tissue-informed approach has the potential to pave the way for a transformation in pulmonary regenerative medicine. Although there are currently fewer examples, engineered biomaterials have been incorporated into pulmonary medical research platforms for tissue regeneration and disease modeling. Bailey et al. systematically evaluated PEG-based hydrogels for supporting extended ex vivo culture of precision-cut lung slices, specifically the maintenance of alveolar epithelial type II (ATII) cells, a progenitor cell capable of differentiation into alveolar epithelial type I (ATI) cells, the cells lining the gas exchange surfaces of the lung. This research demonstrated that incorporation of two short peptide sequences that bind β1-class integrins (0.2 mM YIGSR and 0.1 mM RGDS) supported production of surfactant protein c, that is, maintained the functionality of ATII cells within PLCS for up to 21 days, in contrast to unembedded controls that only survive ~7 days in culture (53). In a recent example of pulmonary disease modeling, a dynamically responsive PEG-α-methacrylate (PEGαMA) hybrid-hydrogel containing proteins from decellularized lung extracellular matrix was stiffened in situ using light to increase the elastic modulus of the material from healthy (E = 3.6 ± 0.24 kPa) to fibrotic ranges (E = 13.4 ± 0.82 kPa). These stiffened hydrogels induced a significant increase in the expression of myofibroblast transgenes within primary murine fibroblasts (80). Likewise, Lewis et al. exploited photodegradable PEG-based hydrogel microspheres to template lung epithelial cells within a biomaterial platform to create open cyst-like structures (81). These 3D model systems were used to demonstrate that fibroblasts in the surrounding hydrogel matrix responded to changes in epithelial cell activity by increasing proliferation and migration when co-cultured with a human tumor-derived epithelial cell line (A549) (82).
It is exciting to imagine a future where tissue-informed biomaterials can incorporate and release biomolecules to sequentially guide stem cell differentiation pathways such as integrin-binding peptides, cytokines, or small molecules (79, 83). This tunability of intrinsic material properties could enable more efficient patient stem cell differentiation toward mature lung tissue. During the progression of many chronic respiratory diseases, considerable changes to the mechanical properties of the lung tissue have been characterized (66). In fibrotic diseases such as idiopathic pulmonary fibrosis and pulmonary arterial hypertension, an aberrant healing response and excess collagen deposition lead to increases in lung stiffness from 1–5 kPa (healthy) to over 10 kPa (fibrotic) (84, 85), while COPD results in an overall decrease in tissue organization and stiffness (85). Biomaterials mimicking these dynamic changes in extracellular matrix mechanics could be readily designed to provide sophisticated in vitro models of patient-specific disease and treatment (80, 86). Currently, the strength of tissue-informed biomaterials has not been harnessed in pulmonary medicine, but the opportunities are substantial and should continue to be investigated in the future.
Outlook
The number of persons affected by chronic respiratory disease worldwide has grown significantly in the last three decades. The COVID-19 pandemic has provided clinical data showing pulmonary fibrosis in those that survive the infection (5, 87). It is hypothesized that this fibrotic response will not regress, leading to a latent burgeoning of chronic respiratory disease in the future. To improve quality of life in patients with chronic diseases, we must understand the disease so that we may engineer the proper treatments. As of now, lung transplantation is the only effective treatment for patients with severe chronic respiratory disease, and the current need far outweighs the available supply. We envision that the solution sits at the intersection of patient-derived stem cells and tissue-informed biomaterials. By engineering biomaterials that can mimic human tissue, we can guide patient stem cells in differentiation toward regeneration of healthy lung tissue or disease models for studying precision medical treatments.
Data Availability Statement
The original contributions presented in the study are included in the article/supplementary material, further inquiries can be directed to the corresponding author/s.
Author Contributions
DC, CS, AR, and CM worked together to conceptualize the content of this perspective. CS and AR wrote the current strategies for modeling human lung development and regeneration section. DC and CM wrote the Opportunities for Tissue-Informed Biomaterials to Advance Pulmonary Regenerative Medicine section, and conceptualized and designed Figure 1. AR and CM shared responsibility for writing and editing the Introduction and Outlook. CM oversaw final edits of the manuscript. All authors contributed extensively to the work presented in this manuscript.
Funding
This work was supported by funding from the Department of Defense (W81XWH-20-1-0037) to DC and CM and the National Institutes of Health, National Institute of Heart, Lung, and Blood (R01 HL153096) to CM. AR was supported by the Cystic Fibrosis Foundation (FIRTH17XX0, FIRTH19XX0), National Institute of Health: National Heart, Lung, Blood Institute (NIH: NHLBI, R01HL139828) and the Hastings Foundation.
Conflict of Interest
The authors declare that the research was conducted in the absence of any commercial or financial relationships that could be construed as a potential conflict of interest.
References
1. Ritchie H, Roser M. Causes of Death. (2018). Retrieved from: https://ourworldindata.org/causes-of-death
2. Soriano JB, Kendrick PJ, Paulson KR, Gupta V, Abrams EM, Adedoyin RA, et al. Prevalence and attributable health burden of chronic respiratory diseases, 1990–2017: a systematic analysis for the Global Burden of Disease Study 2017. Lancet Respir Med. (2020) 8:585–96. doi: 10.1016/S2213-2600(20)30105-3
3. Burnett RT, Brook JR, Yung WT, Dales RE, Krewski D. Association between Ozone and Hospitalization for Respiratory Diseases in 16 Canadian Cities. Environ Res. (1997) 72:24–31. doi: 10.1006/enrs.1996.3685
4. Johannson KA, Vittinghoff E, Lee K, Balmes JR, Ji W, Kaplan GG, et al. Acute exacerbation of idiopathic pulmonary fibrosis associated with air pollution exposure. Eur Respir J. (2014) 43:1124–31. doi: 10.1183/09031936.00122213
5. Spagnolo P, Balestro E, Aliberti S, Cocconcelli E, Biondini D, Casa GD, et al. Pulmonary fibrosis secondary to COVID-19: a call to arms? Lancet Respir Med. (2020) 8:750–2. doi: 10.1016/S2213-2600(20)30222-8
6. Hobbs BD, Cho MH. Dissecting respiratory disease heterogeneity through the genetics of diffusing capacity. Eur Respir J. (2018) 52:1801468. doi: 10.1183/13993003.01468-2018
7. Metzger RJ, Klein OD, Martin GR, Krasnow MA. The branching programme of mouse lung development. Nature. (2008) 453:745–50. doi: 10.1038/nature07005
8. Perrin S. Preclinical research: make mouse studies work. Nat News. (2014) 507:423. doi: 10.1038/507423a
9. Zhou Z, Duerr J, Johannesson B, Schubert SC, Treis D, Harm M, et al. The ENaC-overexpressing mouse as a model of cystic fibrosis lung disease. J Cyst Fibros. (2011) 10:S172–S182. doi: 10.1016/S1569-1993(11)60021-0
10. Lavelle GM, White MM, Browne N, McElvaney NG, Reeves EP. Animal models of cystic fibrosis pathology: phenotypic parallels and divergences. BioMed Res Int. (2016) 2016:1–14. doi: 10.1155/2016/5258727
11. Raju SV, Kim H, Byzek SA, Tang LP, Trombley JE, Jackson P, et al. A ferret model of COPD-related chronic bronchitis. JCI Insight. (2016) 1:e87536. doi: 10.1172/jci.insight.87536
12. McCarron A, Donnelley M, Parsons D. Airway disease phenotypes in animal models of cystic fibrosis. Respir Res. (2018) 19:54. doi: 10.1186/s12931-018-0750-y
13. Rogers CS, Abraham WM, Brogden KA, Engelhardt JF, Fisher JT, McCray PB, et al. The porcine lung as a potential model for cystic fibrosis. Am J Physiol Lung Cell Mol Physiol. (2008) 295:L240–L263. doi: 10.1152/ajplung.90203.2008
14. Sun X, Sui H, Fisher JT, Yan Z, Liu X, Cho H-J, et al. Disease phenotype of a ferret CFTR-knockout model of cystic fibrosis. J Clin Invest. (2010) 120:3149–60. doi: 10.1172/JCI43052
15. Neuberger T, Burton B, Clark H, Van Goor F. Use of primary cultures of human bronchial epithelial cells isolated from cystic fibrosis patients for the pre-clinical Testing of CFTR modulators. In: Amaral MD, K Kunzelmann K, editors. Cystic Fibrosis: Diagnosis and Protocols, Volume I: Approaches to Study and Correct CFTR Defects. Totowa, NJ: Humana Press (2011). p. 39–54.
16. Randell SH, Fulcher ML, O'Neal W, Olsen JC. Primary epithelial cell models for cystic fibrosis research. In: Amaral MD, Kunzelmann K, editors. Cystic Fibrosis: Diagnosis and Protocols, Volume II: Methods and Resources to Understand Cystic Fibrosis. Totowa, NJ: Humana Press (2011). p. 285–310.
17. Huang SXL, Islam MN, O'Neill J, Hu Z, Yang Y-G, Chen Y-W, et al. Efficient generation of lung and airway epithelial cells from human pluripotent stem cells. Nat Biotechnol. (2014) 32:84–91. doi: 10.1038/nbt.2754
18. Hawkins FJ, Suzuki S, Beermann ML, Barillà C, Wang R, Villacorta-Martin C, et al. Derivation of airway basal stem cells from human pluripotent stem cells. Cell Stem Cell. (2020) 28:79–95. doi: 10.1016/j.stem.2020.09.017
19. Rock JR, Onaitis MW, Rawlins EL, Lu Y, Clark CP, Xue Y, et al. Basal cells as stem cells of the mouse trachea and human airway epithelium. Proc National Acad Sci. (2009) 106:12771–5. doi: 10.1073/pnas.0906850106
20. Mou H, Vinarsky V, Tata PR, Brazauskas K, Choi SH, Crooke AK, et al. Dual SMAD signaling inhibition enables long-term expansion of diverse epithelial basal cells. Cell Stem Cell. (2016) 19:217–31. doi: 10.1016/j.stem.2016.05.012
21. Gowers KHC, Hynds RE, Thakrar RM, Carroll B, Birchall MA, Janes SM. Optimized isolation and expansion of human airway epithelial basal cells from endobronchial biopsy samples. J Tissue Eng Regen Med. (2018) 12:e313–e317. doi: 10.1002/term.2466
22. Levardon H, Yonker LM, Hurley BP, Mou H. Expansion of airway basal cells and generation of polarized epithelium. Bio Protoc. (2018) 8:e2877. doi: 10.21769/BioProtoc.2877
23. Hong KU, Reynolds SD, Giangreco A, Hurley CM, Stripp BR. Clara cell secretory protein–expressing cells of the airway neuroepithelial body microenvironment include a label-retaining subset and are critical for epithelial renewal after progenitor cell depletion. Am J Respir Cell Mol Biol. (2001) 24:671–81. doi: 10.1165/ajrcmb.24.6.4498
24. Rawlins EL, Okubo T, Xue Y, Brass DM, Auten RL, Hasegawa H, et al. The role of Scgb1a1+ clara cells in the long-term maintenance and repair of lung airway, but not alveolar, epithelium. Cell Stem Cell. (2009) 4:525–34. doi: 10.1016/j.stem.2009.04.002
25. Zheng D, Soh B-S, Yin L, Hu G, Chen Q, Choi H, et al. Differentiation of club cells to alveolar epithelial cells in vitro. Sci Rep. (2017) 7:41661. doi: 10.1038/srep41661
26. Shatos MA, Ri'os JD, Horikawa Y, Hodges RR, Chang EL, Bernardino CR, et al. Isolation and characterization of cultured human conjunctival goblet cells. Investig Opthalmol Visual Sci. (2003) 44:2477. doi: 10.1167/iovs.02-0550
27. Firth AL, Dargitz CT, Qualls SJ, Menon T, Wright R, Singer O, et al. Generation of multiciliated cells in functional airway epithelia from human induced pluripotent stem cells. Proc National Acad Sci. (2014) 111:E1723–E1730. doi: 10.1073/pnas.1403470111
28. Gomi K, Arbelaez V, Crystal RG, Walters MS. Activation of NOTCH1 or NOTCH3 signaling skews human airway basal cell differentiation toward a secretory pathway. PLoS ONE. (2015) 10:e0116507. doi: 10.1371/journal.pone.0116507
29. Gonzalez RF, Dobbs LG. Isolation and culture of alveolar epithelial type i and type ii cells from rat lungs. In: Randell SH, Fulcher ML, editors. Epithelial Cell Culture Protocols. Totowa, NJ: Humana Press (2012).p. 145–59.
30. Jacob A, Morley M, Hawkins F, McCauley KB, Jean JC, Heins H, et al. Differentiation of human pluripotent stem cells into functional lung alveolar epithelial cells. Cell Stem Cell. (2017) 21:472–88.e410. doi: 10.1016/j.stem.2017.08.014
31. Barkauskas CE, Cronce MJ, Rackley CR, Bowie EJ, Keene DR, Stripp BR, et al. Type 2 alveolar cells are stem cells in adult lung. J Clin Investig. (2013) 123:3025–36. doi: 10.1172/JCI68782
32. Fulcher ML, Randell SH. Human nasal and tracheo-bronchial respiratory epithelial cell culture. In: Randell SH, Fulcher ML, editors. Epithelial Cell Culture Protocols: Second Edition. Totowa, NJ: Humana Press (2013). p. 109–21.
33. Fulcher ML, Randell SH. Human nasal and tracheo-bronchial respiratory epithelial cell culture. In: Randell SH, Fulcher ML, editors. Epithelial Cell Culture Protocols: Second Edition. Totowa, NJ: Humana Press (2013). p. 109–21.
34. Doherty GM, Christie SN, Skibinski G, Puddicombe SM, Warke TJ, de Courcey F, et al. Non-bronchoscopic sampling and culture of bronchial epithelial cells in children. Clin Exp Allergy. (2003) 33:1221–5. doi: 10.1046/j.1365-2222.2003.01752.x
35. Martinovich KM, Iosifidis T, Buckley AG, Looi K, Ling KM, Sutanto EN, et al. Conditionally reprogrammed primary airway epithelial cells maintain morphology, lineage and disease specific functional characteristics. Sci Rep. (2017) 7:17971. doi: 10.1038/s41598-017-17952-4
36. Takahashi K, Tanabe K, Ohnuki M, Narita M, Ichisaka T, Tomoda K, et al. Induction of pluripotent stem cells from adult human fibroblasts by defined factors. Cell. (2007) 131:861–72. doi: 10.1016/j.cell.2007.11.019
37. Yu J, Vodyanik MA, Smuga-Otto K, Antosiewicz-Bourget J, Frane JL, Tian S, et al. Induced pluripotent stem cell lines derived from human somatic cells. Science. (2007) 318:5. doi: 10.1126/science.1151526
38. Cherry ABC, Daley GQ. Reprogrammed cells for disease modeling and regenerative medicine. Ann Rev Med. (2013) 64:277–90. doi: 10.1146/annurev-med-050311-163324
39. Green MD, Chen A, Nostro M-C, d'Souza SL, Schaniel C, Lemischka IR, et al. Generation of anterior foregut endoderm from human embryonic and induced pluripotent stem cells. Nat Biotechnol. (2011) 29:267–72. doi: 10.1038/nbt.1788
40. Ghaedi M, Niklason LE. Human pluripotent stem cells (iPSC) generation, culture, and differentiation to lung progenitor cells. In: Turksen K, editor. Organoids. New York, NY: Springer New York (2016). p. 55–92.
41. Dye BR, Hill DR, Ferguson MAH, Tsai Y-H, Nagy MS, Dyal R, et al. In vitro generation of human pluripotent stem cell derived lung organoids. eLife. (2015) 4:e05098. doi: 10.7554/eLife.05098
42. Wagner DE, Bonenfant NR, Sokocevic D, DeSarno MJ, Borg ZD, Parsons CS, et al. Three-dimensional scaffolds of acellular human and porcine lungs for high throughput studies of lung disease and regeneration. Biomaterials. (2014) 35:2664–79. https://doi.org/10.1016/j.biomaterials.2013.11.078
43. Pouliot RA, Link PA, Mikhaiel NS, Schneck MB, Valentine MS, Kamga Gninzeko FJ, et al. Development and characterization of a naturally derived lung extracellular matrix hydrogel. J Biomed Mater Res Part A. (2016) 104:1922–35. https://doi.org/10.1002/jbm.a.35726
44. Suki B, Ito S, Stamenović D, Lutchen KR, Ingenito EP. Biomechanics of the lung parenchyma: critical roles of collagen and mechanical forces. J Appl Physiol. (2005) 98:1892–9. doi: 10.1152/japplphysiol.01087.2004
45. Plosa E, Zent R. Integrin regulation of the lung epithelium. In: Lung Epithelial Biology in the Pathogenesis of Pulmonary Disease. The American Society for Clinical Investigation (2017). p. 77–89. doi: 10.1172/jci.insight.129259
46. Davis-Hall D, Nguyen V, D'Ovidio TJ, Tsai E, Bilousova G, Magin CM. Peptide-functionalized hydrogels modulate integrin expression and stemness in adult human epidermal keratinocytes. Adv Biosys. (2019) 3:1900022. doi: 10.1002/adbi.201900022
47. Santoro R, Perrucci GL, Gowran A, Pompilio G. Unchain my heart: integrins at the basis of iPSC cardiomyocyte differentiation. Stem Cells Int. (2019) 2019:8203950. doi: 10.1155/2019/8203950
48. Hilderbrand AM, Ford EM, Guo C, Sloppy JD, Kloxin AM. Hierarchically structured hydrogels utilizing multifunctional assembling peptides for 3D cell culture. Biomater Sci. (2020) 8:1256–69. doi: 10.1039/C9BM01894H
49. Gobin AS, West JL. Val-ala-pro-gly, an elastin-derived non-integrin ligand: smooth muscle cell adhesion and specificity. J Biomed Mater Res Part A. (2003) 67A:255–9. https://doi.org/10.1002/jbm.a.10110
50. Almine JF, Bax DV, Mithieux SM, Nivison-Smith L, Rnjak J, Waterhouse A, et al. Elastin-based materials. Chem Soc Rev. (2010) 39:3371–9. doi: 10.1039/B919452P
51. Gomes M, Azevedo H, Malafaya P, Silva S, Oliveira J, Silva G, et al. 16—natural polymers in tissue engineering applications. In: Handbook of Biopolymers and Biodegradable Plastics. S Ebnesajjad, editor. Boston: William Andrew Publishing (2013). p. 385–425.
52. Burgstaller G, Oehrle B, Gerckens M, White ES, Schiller HB, Eickelberg O. The instructive extracellular matrix of the lung: basic composition and alterations in chronic lung disease. Eur Respir J. (2017) 50:1601805. doi: 10.1183/13993003.01805-2016
53. Bailey KE, Pino C, Lennon ML, Lyons A, Jacot JG, Lammers SR, et al. Embedding of precision-cut lung slices in engineered hydrogel biomaterials supports extended ex vivo culture. Am J Respir Cell Mol Biol. (2020) 62:14–22. doi: 10.1165/rcmb.2019-0232MA
54. Sheppard D. Functions of pulmonary epithelial integrins: from development to disease. Physiol Rev. (2003) 83:673–86. doi: 10.1152/physrev.00033.2002
55. Aisenbrey EA, Murphy WL. Synthetic alternatives to matrigel. Nat Rev Mater. (2020) 5:539–51. doi: 10.1038/s41578-020-0199-8
56. Dunsmore SE, Rannels DE. Extracellular matrix biology in the lung. Am J Physiol Lung Cell Mol Physiol. (1996) 270:L3–L27. doi: 10.1152/ajplung.1996.270.1.L3
57. Hachim D, Whittaker TE, Kim H, Stevens MM. Glycosaminoglycan-based biomaterials for growth factor and cytokine delivery: making the right choices. J Control Release. (2019) 313:131–47. doi: 10.1016/j.jconrel.2019.10.018
58. Young BM, Shankar K, Tho CK, Pellegrino AR, Heise RL. Laminin-driven Epac/Rap1 regulation of epithelial barriers on decellularized matrix. Acta Biomaterialia. (2019) 100:223–34. https://doi.org/10.1016/j.actbio.2019.10.009
59. Greaney AM, Adams TS, Brickman Raredon MS, Gubbins E, Schupp JC, Engler AJ, et al. Platform effects on regeneration by pulmonary basal cells as evaluated by single-cell RNA sequencing. Cell Rep. (2020) 30:4250–65.e4256. https://doi.org/10.1016/j.celrep.2020.03.004
60. De Santis MM, Alsafadi HN, Tas S, Bölükbas DA, Prithiviraj S, Da Silva IAN, et al. Extracellular-matrix-reinforced bioinks for 3D bioprinting human tissue. Adv Mater. (2020). 33:2005476. https://doi.org/10.1002/adma.202005476
61. Goetzke R, Keijdener H, Franzen J, Ostrowska A, Nüchtern S, Mela P, et al. Differentiation of induced pluripotent stem cells towards mesenchymal stromal cells is hampered by culture in 3D hydrogels. Sci Rep. (2019) 9:15578. doi: 10.1038/s41598-019-51911-5
62. Downing TL, Soto J, Morez C, Houssin T, Fritz A, Yuan F, et al. Biophysical regulation of epigenetic state and cell reprogramming. Nat Mater. (2013) 12:1154–62. doi: 10.1038/nmat3777
63. Hughes CS, Postovit LM, Lajoie GA. Matrigel: A complex protein mixture required for optimal growth of cell culture. Proteomics. (2010) 10:1886–90. doi: 10.1002/pmic.200900758
64. Tong Z, Solanki A, Hamilos A, Levy O, Wen K, Yin X, et al. Application of biomaterials to advance induced pluripotent stem cell research and therapy. EMBO J. (2015) 34:987–1008. doi: 10.15252/embj.201490756
65. Gilpin SE, Ren X, Okamoto T, Guyette JP, Mou H, Rajagopal J, et al. Enhanced lung epithelial specification of human iPSCs on decellularized lung matrix. Ann Thorac Surg. (2015) 98:1721–9. doi: 10.1016/j.athoracsur.2014.05.080
66. Bailey KE, Floren ML, D'Ovidio TJ, Lammers SR, Stenmark KR, Magin CM. Tissue-informed engineering strategies for modeling human pulmonary diseases. Am J Physiol Lung Cell Mol Physiol. (2019) 316:L303–L320. doi: 10.1152/ajplung.00353.2018
67. Lam J, Carmichael ST, Lowry WE, Segura T. Hydrogel design of experiments methodology to optimize hydrogel for iPSC-NPC culture. Adv Healthc Mater. (2015) 4:534–9. doi: 10.1002/adhm.201400410
68. Gjorevski N, Sachs N, Manfrin A, Giger S, Bragina ME, Ordóñez-Morán P, et al. Designer matrices for intestinal stem cell and organoid culture. Nature. (2016) 539:560–4. doi: 10.1038/nature20168
69. Lenzini S, Devine D, Shin J-W. Leveraging biomaterial mechanics to improve pluripotent stem cell applications for tissue engineering. Front Bioeng Biotechnol. (2019) 7:260. doi: 10.3389/fbioe.2019.00260
70. Burdick JA, Murphy WL. Moving from static to dynamic complexity in hydrogel design. Nat Commun. (2012) 3:1269. doi: 10.1038/ncomms2271
71. Kirschner CM, Anseth KS, Kirschner CM, Kirschner C. Hydrogels in healthcare: from static to dynamic material. Microenvironments. (2013) 26:931–94. doi: 10.1016/j.actamat.2012.10.037
72. Kloxin AM, Benton JA, Anseth KS. In situ elasticity modulation with dynamic substrates to direct cell phenotype. Biomaterials. (2010) 31:1–8. doi: 10.1016/j.biomaterials.2009.09.025
73. Kloxin AM, Tibbitt MW, Anseth KS. Synthesis of photodegradable hydrogels as dynamically tunable cell culture platforms. Nat Protoc. (2010) 5:1867–87. doi: 10.1038/nprot.2010.139
74. Hushka EA, Yavitt FM, Brown TE, Dempsey PJ, Anseth KS. Relaxation of extracellular matrix forces directs crypt formation and architecture in intestinal organoids. Adv Healthc Mater. (2020) 9:1901214. doi: 10.1002/adhm.201901214
75. McCall JD, Luoma JE, Anseth KS. Covalently tethered transforming growth factor beta in PEG hydrogels promotes chondrogenic differentiation of encapsulated human mesenchymal stem cells. Drug Deliv Transl Res. (2012) 2:305–12. doi: 10.1007/s13346-012-0090-2
76. DeForest CA, Anseth KS. Cytocompatible click-based hydrogels with dynamically tunable properties through orthogonal photoconjugation and photocleavage reactions. Nat Chem. (2011) 3:925–31. doi: 10.1038/nchem.1174
77. Sargeant TD, Desai AP, Banerjee S, Agawu A, Stopek JB. An in situ forming collagen–PEG hydrogel for tissue regeneration. Acta Biomaterialia. (2012) 8:124–32. doi: 10.1016/j.actbio.2011.07.028
78. Koehler KC, Alge DL, Anseth KS, Bowman CN. A Diels–Alder modulated approach to control and sustain the release of dexamethasone and induce osteogenic differentiation of human mesenchymal stem cells. Biomaterials. (2013) 34:4150–8. doi: 10.1016/j.biomaterials.2013.02.020
79. Ovadia EM, Colby DW, Kloxin AM. Designing well-defined photopolymerized synthetic matrices for three-dimensional culture and differentiation of induced pluripotent stem cells. Biomater Sci. (2018) 6:1358–70. doi: 10.1039/C8BM00099A
80. Petrou CL, D'Ovidio TJ, Bölükbas DA, Tas S, Brown RD, Allawzi A, et al. Clickable decellularized extracellular matrix as a new tool for building hybrid-hydrogels to model chronic fibrotic diseases in vitro. J Mater Chem B. (2020) 8:6814–26. doi: 10.1039/D0TB00613K
81. Lewis KJR, Tibbitt MW, Zhao Y, Branchfield K, Sun X, Balasubramaniam V, et al. In vitro model alveoli from photodegradable microsphere templates. Biomater Sci. (2015) 3:821–32. doi: 10.1039/C5BM00034C
82. Lewis KJR, Hall JK, Kiyotake EA, Christensen T, Balasubramaniam V, Anseth KS. Epithelial-mesenchymal crosstalk influences cellular behavior in a 3D alveolus-fibroblast model system. Biomaterials. (2018) 155:124–34. doi: 10.1016/j.biomaterials.2017.11.008
83. Smagul S, Kim Y, Smagulova A, Raziyeva K, Nurkesh A, Saparov A. Biomaterials loaded with growth factors/cytokines and stem cells for cardiac tissue regeneration. Int J Mol Sci. (2020) 21:5952. doi: 10.3390/ijms21175952
84. Liu F, Haeger CM, Dieffenbach PB, Sicard D, Chrobak I, Coronata AMF, et al. Distal vessel stiffening is an early and pivotal mechanobiological regulator of vascular remodeling and pulmonary hypertension. JCI Insight. (2016) 1:e86987. doi: 10.1172/jci.insight.86987
85. de Hilster RHJ, Sharma PK, Jonker MR, White ES, Gercama EA, Roobeek M, et al. Human lung extracellular matrix hydrogels resemble the stiffness and viscoelasticity of native lung tissue. Am J Physiol Lung Cell Mol Physiol. (2020) 318:L698–L704. doi: 10.1152/ajplung.00451.2019
86. Matera DL, DiLillo KM, Smith MR, Davidson CD, Parikh R, Said M, et al. Microengineered 3D pulmonary interstitial mimetics highlight a critical role for matrix degradation in myofibroblast differentiation. Sci Adv. (2020) 6:eabb5069. doi: 10.1126/sciadv.abb5069
Keywords: disease modeling, regenerative medicine, pulmonary, hydrogel, tissue-informed engineering, biomaterials
Citation: Campbell DR Jr, Senger CN, Ryan AL and Magin CM (2021) Engineering Tissue-Informed Biomaterials to Advance Pulmonary Regenerative Medicine. Front. Med. 8:647834. doi: 10.3389/fmed.2021.647834
Received: 30 December 2020; Accepted: 09 March 2021;
Published: 08 April 2021.
Edited by:
Fernanda Ferreira Cruz, Federal University of Rio de Janeiro, BrazilReviewed by:
Koshika Yadava, University of Oxford, United KingdomPai-Chien Chou, Taipei Medical University Hospital, Taiwan
Copyright © 2021 Campbell, Senger, Ryan and Magin. This is an open-access article distributed under the terms of the Creative Commons Attribution License (CC BY). The use, distribution or reproduction in other forums is permitted, provided the original author(s) and the copyright owner(s) are credited and that the original publication in this journal is cited, in accordance with accepted academic practice. No use, distribution or reproduction is permitted which does not comply with these terms.
*Correspondence: Chelsea M. Magin, Y2hlbHNlYS5tYWdpbkBjdWFuc2NodXR6LmVkdQ==