- 14th Department of Internal Medicine, Medical School, National and Kapodistrian University of Athens, “Attikon” University Hospital, Athens, Greece
- 2Department of Medicine, David Geffen School of Medicine, University of California, Los Angeles, Los Angeles, CA, United States
The advent of highly sensitive molecular diagnostic techniques has improved our ability to detect viral pathogens leading to severe and often fatal infections that require admission to the Intensive Care Unit (ICU). Viral infections in the ICU have pleomorphic clinical presentations including pneumonia, acute respiratory distress syndrome, respiratory failure, central or peripheral nervous system manifestations, and viral-induced shock. Besides de novo infections, certain viruses fall into latency and can be reactivated in both immunosuppressed and immunocompetent critically ill patients. Depending on the viral strain, transmission occurs either directly through contact with infectious materials and large droplets, or indirectly through suspended air particles (airborne transmission of droplet nuclei). Many viruses can efficiently spread within hospital environment leading to in-hospital outbreaks, sometimes with high rates of mortality and morbidity, thus infection control measures are of paramount importance. Despite the advances in detecting viral pathogens, limited progress has been made in antiviral treatments, contributing to unexpectedly high rates of unfavorable outcomes. Herein, we review the most updated data on epidemiology, common clinical features, diagnosis, pathogenesis, treatment and prevention of severe community- and hospital-acquired viral infections in the ICU settings.
Introduction
The incidence of viral infections in critical care settings varies widely across different studies and geographical regions, but it has unambiguously increased over the last years as modern diagnostics have become more sensitive, rapid and accurate (1, 2). The advent of sophisticated molecular tools has facilitated the detection of emerging viral pathogens, such as the novel Severe Acute Respiratory Syndrome coronavirus 2 (SARS-CoV-2), leading to the identification of viruses as culprits in previously undiagnosed conditions. Due to the fact that antiviral therapies are still scarce, the mainstay of treatment for many life-threatening viral infections remains supportive, and therefore critical care is of paramount importance for the management of severe viral infections (3).
In this narrative review we aim to present an updated overview of the epidemiology, geographic distribution, clinical features, diagnosis, treatment and specific preventative measures for viral pathogens associated with (i) severe community acquired respiratory tract infections and nosocomial pneumonia (ii) neurological and neuromuscular diseases requiring intensive care unit (ICU) admission, (iii) different types of shock and multi-organ injury, and finally, (iv) re-activation, in critically ill patients.
Epidemiology of Viral Pathogens Causing Critical Illness
Respiratory Viruses
Lower respiratory tract infections (LRTIs) are among the leading causes of ICU resource utilization (4). Before the advent of the SARS-CoV-2 pandemic, the incidence of viral-associated LRTIs requiring ICU admission varied greatly among different studies, and ranged between 16 and 49%, with the most frequently isolated viruses being influenza, human rhinoviruses (HRhV), human coronaviruses (HCoV), respiratory syncytial virus (RSV), human metapneumovirus (HMPV), human parainfluenza viruses (HPIV), and adenoviruses (HAdV) (4–7). Common viruses are detected in respiratory samples of patients with nosocomial pneumonia with an incidence ranging from 13.8 to 32% in adults, and as high as 69.2% in pediatric population (8–13).
Current seasonal influenza epidemics are caused by influenza A strains (A/H3N2 and the 2009 pandemic strain H1N1—A/H1N1pdm09) and influenza B lineages (B/Yamagata and B/Victoria), resulting in 3–5 million cases of severe infections annually around the world (14). The annual casualties from influenza epidemics are estimated up to 650,000 people globally, while the respiratory excess mortality rate is estimated between 0.1 and 6.4/100,000 for patients younger than 65 years, 2.9 and 44/100,000 for people 65 and 74 years old, and as high as 17.9–223.5/100,000 for those older than 75 years old (15). The Centers for Disease Control and Prevention (CDC) reported that annual estimates of flu cases, hospitalizations and deaths in the United States (U.S.) ranged among 9–45 million, 140,000–810,000 and 12,000–61,000, respectively during 2010–2020, with the 2017–2018 flu season bearing the highest disease burden (16). A U.S. study estimated that among 114,000–633,000 influenza-hospitalizations during three flu seasons (2010–2013), 18,000–96,000 patents required ICU admission (17). The same study demonstrated that the risk of death during influenza admissions increased with age (0.2–0.9% in children<18 years old, 1.8–2.8% of adults 18–64 years old, and 3.4–4.7% of those ≥65 years old), and the majority of hospitalizations (54–70%) and deaths (71–85%) occurred in patients aged ≥65 years old (17).
HCoVs are ubiquitous viruses with worldwide distribution and yearlong transmissibility [Figure 1; (18, 19)]. Among them, three betacoronoviruses have caused epidemics of severe pneumonia within the last 20 years. Severe acute respiratory syndrome coronavirus (SARS-CoV) and Middle East respiratory syndrome coronavirus (MERS-CoV) caused outbreaks and epidemics of severe acute respiratory infections mainly in China and the Arabian peninsula (19–22). SARS-CoV-2 emerged in China in December 2019 and has led to an unprecedented pandemic with more than 90 million confirmed cases of the so-called Coronavirus Disease 2019 (COVID-19) and nearly 2 million deaths across the globe (23). Since the onset of the pandemic, cumulative data from the U.S. demonstrate that the majority of SARS-CoV-2 infections (54%) are diagnosed among people aged 18–49 years old; while only 10% of the total diagnoses are made in older adults (≥65 years old), this age group represents the majority of symptomatic cases and COVID-19-associated hospitalizations (90 and 46%, respectively) (24). Both symptomatic illness and hospitalization rates are very low among children aged 0–4 years old (3 and 1%, respectively) (24). The current global case fatality rate ranges between 1.1 and 4.4% among different countries, although during the first wave of the pandemic this rate was much higher in Italy and Spain, reaching up to 14.5 and 11.4%, respectively (25). Finally, the number of COVID-19 patients requiring ICU admission varies greatly among countries and among the different phases of the pandemic, but it ranges between 0.2 and 108 cases per million population (26). MERS-CoV causes outbreaks in Middle East mainly within hospital settings (27). Since the first confirmed case in 2012, the World Health Organization (WHO) has reported 2,494 cases of MERS-CoV in 27 countries and 858 deaths among them, leading to a case fatality rate of 36% (28, 29).
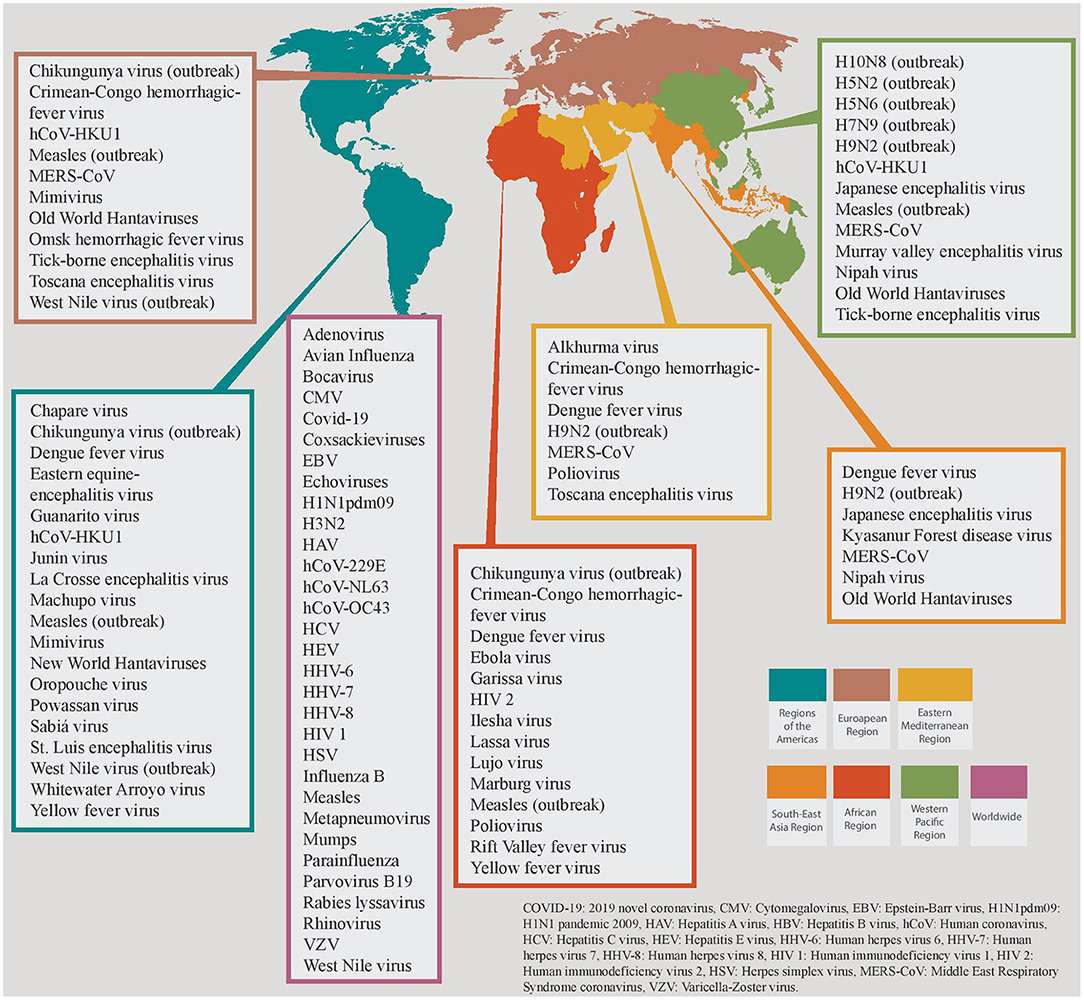
Figure 1. Global distribution of viruses causing critical illness divided in the 6 World Health Organization (WHO) Regions. Viruses that are restricted in specific WHO regions are grouped together, and different colors are attributed to each region. Viruses that share a worldwide distribution are grouped in the magenta colored box.
HPIVs are common causes of RTIs, targeting primarily young children and causing nearly 40% of all acute RTIs in this age group (30). Adults can also be infected; up to 11.5% of adult hospitalizations for RTIs attributed to HPIVs (31–33). An epidemiological study in Kuwait demonstrated that HPIVs were isolated from 2.6% of immunocompetent patients with viral infections requiring admission in pediatric and adult ICUs (34). Concerning HRhV, a recent epidemiological study in mechanically ventilated adult patients in five ICUs demonstrated that HRhV were the second most prevalent virus (5%) after HPIV (6%), independently of the cause of ICU admission (35). Moreover, the prevalence of HRhVs was significantly higher in patients with severe RTIs (9.6%) compared to those admitted for other reasons (2.6%), thus signifying their contribution in severe LRTIs (35). Another retrospective study reported a prevalence of HRhV of 7.5% and a mortality rate of 18.2% among adult critically ill patients, although not all these deaths were directly connected to HRhV detection; however, among all HRhV-positive patients, 59.1% fulfilled the definition of pneumonia (36). The incidence of RSV infections in adults is surprisingly higher than what it was previously believed. Notably, RSV is detected in 12% of all medically attended adults aged ≥50 years old with RTIs (37–39). In 2015, about 1.5 million older adults in industrialized countries suffered from RSV-associated acute RTIs, and 214,000 (14.5%) were admitted to hospital; the same year, RSV was associated with 14,000 in-hospital deaths in older adults globally (40). According to data from the U.S., the annual incidence of RSV infection in elderly adults has been reported about 3–7% and 4–10% of healthy and high-risk patients, respectively (41). Moreover, this study found similar clinical outcomes for both RSV and influenza A infections in terms of length of hospital stay, need for ICU and mortality rates (41). Specific adult groups are at high risk for severe infections and intubation form HMPV infections, but younger patients may also develop severe LRTI and ARDS requiring intubation and mechanical ventilation (35, 42–44). A recent multicenter study in France found an incidence of HMPV-positive patients of 3% among 3,148 hospitalized adults with acute RTIs (45). In this study, 21% required admission in ICU and 4% died during hospitalization among HMPV-positive patients (45). Other studies have reported HMPV-related mortality rates in adults comparable with or slightly lower than those of influenza and RSV (43, 44).
Other Viruses
The most commonly identified viral pathogens among critically ill patients with neurological infections (mainly encephalitis and meningoencephalitis) include herpes simplex virus (HSV), varicella zoster virus (VZV), and arboviruses like West Nile Virus (WNV). The epidemiology of the viruses that are implicated in central and/or peripheral nervous system infections is outlined in Table 1.
Ebola virus is among the deadliest pathogens of viral hemorrhagic fever (VHF) syndrome, with a case fatality rate varying from 25 to 90% and an average mortality rate of 50% (59). Between 2014 and 2016, an Ebola epidemic resulted in more than 28,000 cases with more than 11,300 casualties in Sierra Leone, Liberia and Guinea, while an outbreak is currently ongoing in West Africa [Figure 1; (59)]. Marburg virus has a case fatality rate ranging from 24% to as high as 88% (average 50%) (60). The virus was initially discovered during a European outbreak caused by imported African monkeys in 1967, and since then is responsible for sporadic cases and outbreaks mainly in Central and South Africa (60). Dengue virus is currently endemic in more than 100 countries across 5 WHO regions, and is accountable for approximately 390 million infections annually; although Asia has about 70% of the global dengue burden, a steep worrisome rise in cases and associated deaths was observed in 2019 across several countries (61). The disease has also caused outbreaks in the European continent, representing the second most common cause of fever (after malaria) in returning travelers from low- and middle-income countries, although these data were drawn before the onset of SARS-CoV-2 pandemic (61). Severe dengue requiring hospitalization is estimated to affect 500 000 people annually, with a case fatality rate of 2.5% (62). Lassa virus is currently endemic in Western Africa (63). In contrast to other VHF pathogens, 80% of infected people remain asymptomatic with an overall mortality rate being as low as 1%; however, case fatality rate among those who develop VHF is 15% (63). Of note, pregnant women at the third trimester are at increased risk for severe infection with Lassa virus with high mortality rates (maternal death and/or fetal loss occurring in more than 80% of the cases) (63). Hantaviruses are divided into the “New World” strains, that cause sporadic cases and outbreaks of “hantavirus cardiopulmonary syndrome” (HCPS) mainly in the Americas, and the “Old World” strains that cause the “hemorrhagic fever with renal syndrome” (HFRS) in Europe and Asia (64, 65). HCPS is a potentially fatal disease with a case fatality rate of 40% (66). One third of the HFRS-deaths are associated with fulminant unresponsive shock. However, case fatality rate in HFRS is not as high as the HCPS, which ranges from <1% to 5–15% (67). Finally, the Crimean-Congo hemorrhagic fever (CCHF) virus is distributed in countries of the Africa, the Balkans, the Middle East and the Asia, and causes outbreaks with mortality rates up to 40% (68).
Pathogenesis of Severe de novo Viral Infections and Viral Reactivation
Physiological Host Responses to Viruses
Adequate innate and adaptive immune responses play a pivotal role in controlling viral infections. Innate immune response begins with the recognition of pathogen-associated molecular patterns (PAMPs) by the pathogen recognition receptors (PRRs) of the host cells (69). The activation of these receptors via signaling pathways induces the production of pro-inflammatory cytokines and anti-viral molecules, notably type I and III interferons (IFNs), as well as the migration of innate immune cells at the site of infection (69). Dendritic cells bridge innate and adaptive immunity by mediating antigen presentation in lymphoid tissues (70). Viral peptides are presented to CD8+ T cells by major histocompatibility complex (MHC) class I molecules, leading to their transformation into effector cells. CD4+ helper T cells are activated by the recognition of antigen-MHC class II complexes on antigen-presenting cells and induce both CD8+ T cell and B cell responses, the latter leading to specific antibody formation (71).
Factors Associated With Severe Viral Infections and Immune Dysregulation
Viral Immune Evasion
Failure of host defense against viral pathogens may be caused either by direct viral-immune evasion or by predisposing host factors. HSV and measles viruses, for example, directly inhibit the maturation of dendritic cells (72). Other viruses, such as Human Immunodeficiency virus (HIV), Hepatitis B virus (HBV) and Hepatitis C virus (HCV), produce anti-inflammatory molecules, especially interleukin-10 (IL-10). Studies have also pointed out the role of PRR inhibition, like retinoic acid-induced gene I (RIG-I) in influenza A, paramyxovirus, and HCV infection (72). Moreover, HSV, Cytomegalovirus (CMV), Epstein Barr virus (EBV), and HIV are able to disrupt the crucial step of antigen presentation. Some viruses, especially those who belong in the Herpesviridae family, become latent, and can reactivate later in response to stress or immunosuppression [Table 2; (73)].
Host Factors Associated With Immune Dysregulation
Numerous host factors contribute to a dysregulated immune response and augment viral virulence leading to severe viral infections with multi-system involvement [Figure 2; (74–83)]. Iatrogenic immunosuppression, HIV-related and stress-induced immunosuppression, comorbidities such as diabetes mellitus, cardiovascular disease and chronic obstructive pulmonary disease, as well as factors like gene polymorhisms, age extremes, and pregnancy are risk factors for severe viral infections like influenza and COVID-19 (84–86).
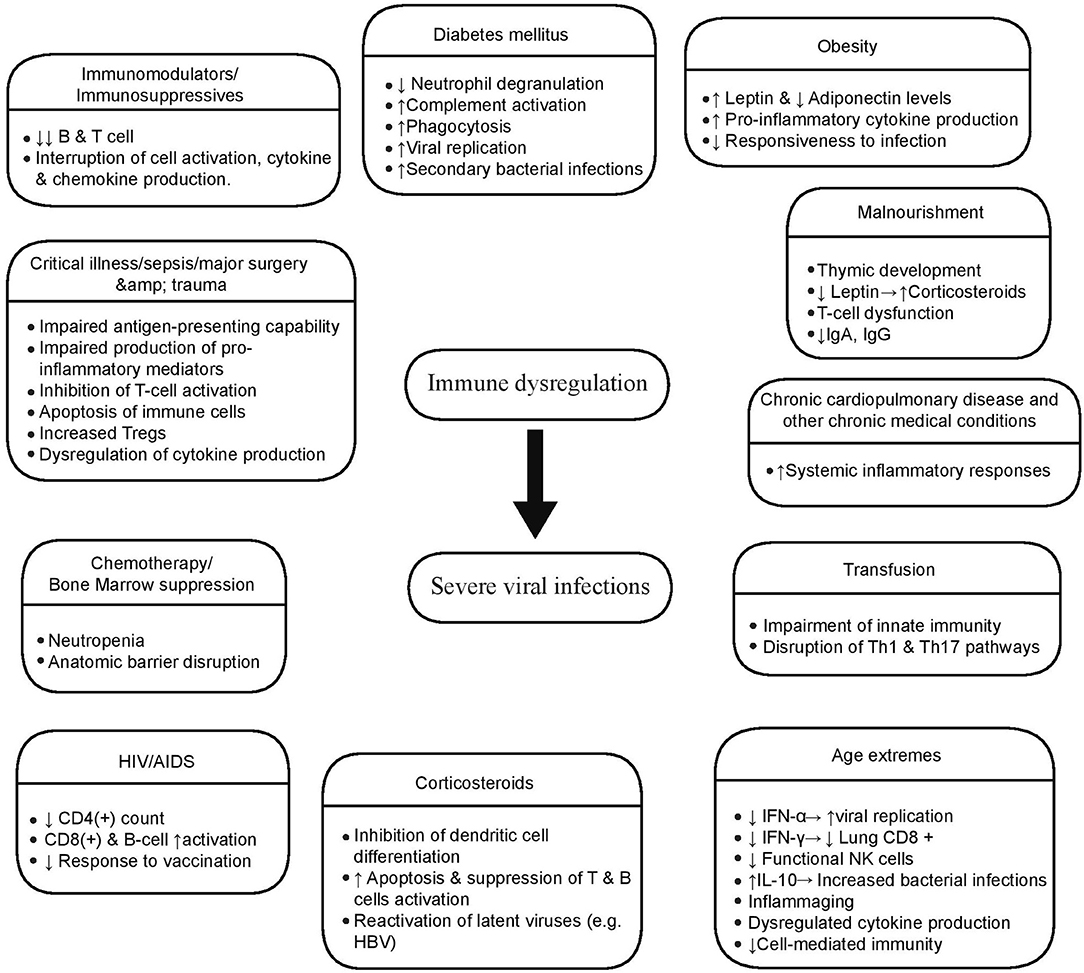
Figure 2. Host factors associated with severe viral infections. Multiple host conditions produce a dysregulated immune state that posits the host in risk of developing a serious viral infection. These factors affect both innate and adaptive immune mechanisms in various ways, the most significant of whom are illustrated here.
Age extremes significantly affect immune responses, and this has an impact on morbidity and mortality of viral infections, such as influenza and SARS-CoV-2 (87). Immature responsiveness of immune system in the first years of life leads to increased risk of severe RSV and influenza infection (88). In old age, serious disturbances in both innate and adaptive immunity lead to dysregulated responses, affecting viral clearance and disease severity, as dramatically seen in COVID-19 disease. Age changes in the immune system include, among others, the impaired production of type I IFNs from dendritic cells, hampered natural killer cell function, reduced responsiveness of T and B cells to antigen, and decreased repertoire of CD8+ T cells, partly due to expansion of inflationary CD8+ T cells caused by chronic viral infection, such as CMV (87). Additionally, inflame-aging, a well-described pro-inflammatory cytokine excess state in old age, contributes to the immune dysregulation (87, 89).
Obesity has also been associated with increased susceptibility to infections leading to high rates of poor outcomes, including influenza and COVID-19 (90, 91). In this patient group, contributing factors are considered to be mechanical, such as increased airway resistance and impaired gas exchange, as well as the underlying immune dysfunction driven by decreased adiponectin production (91). Adiponectin, an adipokine that is reduced in obesity, normally downregulates macrophage activation and pro-inflammatory cytokine production (91). Thus, low adiponectin, high leptin levels, and high leptin-resistance contribute to a heightened pro-inflammatory cytokine production in obese patients, and a subsequent blunted immune response to infection by influenza (83). Low adiponectin levels have been reported during pregnancy as well; however, whether this is a key element of immune dysregulation observed in pregnant women with severe influenza infection, is still not clear (83). Besides baseline host risk factors, other iatrogenic interventions in critically ill patients may contribute to immune dysregulation leading to increased rates of (severe) viral infections, like immunomodulators and blood transfusion. Blood transfusion, especially in the peri-operative period for surgical patients, confers an additional degree of immunosuppression (81). The transfusion of allogeneic blood has known immunosuppressive effects and its impact on the emergence of nosocomial infections, including viremia, viral pneumonia and viral reactivation, has been documented (92–94). The adoption of leukoreduction as an institutional policy for all critically ill patients irrespective of prior immunodeficiency status may be an efficient method in reducing the consequences of transfusion-related immunomodulation.
Stress-Induced Immunosuppression and Viral Reactivation
After primary infection, multiple viruses fall into latency within the host cells; characteristic examples are the members of Herpesviridae family, which become dormant inside neurons (VZV, HSV-1, HSV-2), B cells (EBV), or myeloid cells (CMV). The capacity of latency allows these viruses to reactivate in response to triggering factors, like stress-induced immune paresis leading to clinical manifestations that vary from minor infections to serious complications (Table 2). A prospective cohort study demonstrated that non-immunocompromised patients with sustained sepsis exhibited considerably high frequencies of detectable viral DNA from a variety of viruses like CMV (24.2%), EBV (53.2%), HSV (14.1%), human herpesvirus 6 (HHV-6) (10.4%), and Transfusion transmitted virus (TTV) (77.5%), with two or more viruses being detected in 42.7% of these patients (95).
CMV infection is defined by the isolation of CMV antigens or nucleic acid in body fluids or tissue specimens, as opposed to CMV disease that necessitates the presence of concurrent symptoms and/or signs (96). According to multiple meta-analyses, the incidence of CMV infection in non-immunocompromised critically ill patients has been reported as high as 35%, occurring usually between the 14th and 21st day of ICU admission (97, 98). The highest incidence is observed among patients with prior seropositivity, indicating that reactivation rather than primary infection is the main mode of CMV infection in this population (98). The flawed balance between exaggerated inflammation and transient immunosuppression in the subset of patients with sepsis sets the grounds for CMV reactivation. Tumor necrosis factor alpha (TNF-α) promotes directly CMV gene expression (99). As T cell immunity plays a major role in suppressing the CMV replication, both CMV-induced immunodeficiency and sepsis-induced immune dysfunction may contribute in CMV reactivation (100–102). A meta-analysis by Lachance et al. reported that CMV reactivation in immunocompetent critically ill patients is associated with adverse outcomes, including higher ICU and overall mortality, prolonged duration of mechanical ventilation and ICU stay, more nosocomial infections and increased need for renal replacement therapy (103).
HSV reactivation has been frequently reported in non-immunocompromised critically ill patients (104). Isolation of HSV from bronchoalveolar lavage fluid specimens has been associated with adverse outcomes, like longer duration of mechanical ventilation, prolonged ICU stay and increased mortality (105). HSV detection has been associated with several risk factors in critically ill patients, such as intubation, prolonged mechanical ventilation, ARDS, extensive burns, advanced age, HSV-IgG seropositivity, use of corticosteroids and herpetic mucocutaneous lesions (97). However, it remains unclear whether HSV isolation in mechanically ventilated patients equals to actual HSV infection (either as reactivation or as a de novo infection) or it is just a surrogate marker of underlying disease severity (106). Hence, it is still controversial whether, how or when treatment should be applied in these cases (107, 108).
VZV reactivation has been associated with prolonged duration of fever in immunocompetent children in the ICU (109). EBV DNA, detected in blood samples of ICU patients, has been correlated with increased morbidity and mortality (110). Nonetheless, the clinical significance and the need of pre-emptive treatment of these reactivations remain obscure and will only be answered by large, well-designed, randomized controlled trials in the diverse population of immunocompetent critically ill patients.
Immunocompromised Patients and Viral Reactivation
The advent of potent immunosuppressive drugs has increased the survival rate of previously bleak diagnoses, especially in hematological patients and solid organ transplant recipients (SOTRs), but at the same time has increased the incidence of opportunistic viral infections and viral reactivations leading to an upsurge in the need for higher level of care.
Hematopoietic Stem Cell Transplant Recipients and Neutropenic Patients
Viral reactivation in hematopoietic stem-cell transplant recipients (HSCTRs) is associated with significant morbidity and mortality. Pre-transplantation evaluation guidelines have been designed to prevent post-transplant infections. Seropositivity screening for CMV, HBV, HCV, VZV, HSV, EBV, and HIV in HSCT donors or candidates outlines the infection control policies and prophylactic regimens necessitated in the post-transplant period (111, 112). According to published guidelines (111) for the prevention of HSV, VZV, and CMV re-activation, seropositive HSV HSCTRs should receive antiviral prophylaxis with intravenous aciclovir from conditioning until engraftment or until mucositis resolves, seropositive VZV HSCTRs should receive antiviral prophylaxis for at least 1 year, preferably with oral valaciclovir while CMV seropositive HSCTRs of allografts from seronegative or seropositive donors, and seronegative HSCTRs of allografts from seropositive donors should be monitored weekly with quantitative CMV testing. Pre-emptive treatment should be started in these patients when there is detectable CMV viremia with intravenous ganciclovir or oral valganciclovir.
Apart from the HSC transplantation, CMV reactivation has been reported in the setting of febrile neutropenia in hematologic patients (113). Neutropenia, especially when the count is <500 cells/μL, increases the risk of opportunistic infections including viral infections or reactivations. Therefore, in episodes of febrile neutropenia in patients with hematologic malignancies, viral de-novo infections or reactivations should always be considered (114, 115).
Solid Organ Transplant Recipients
Infections remain a major impediment in SOTRs; as for HSCTRs, laboratory testing is undertaken in the pre-transplant period to evaluate for past infectious exposures and exclude active infections with serology test for CMV, HSV, VZV, EBV, HIV, HBV, and HCV (116). The highest incidence of viral infections occurs in the subset of immunologically naïve patients who receive grafts from infected donors.
Viral reactivation in SOTRs leads to a wide array of clinical manifestations. Examples include neutropenic fever, colitis, adrenal insufficiency, meningoencephalitis (CMV), cholangitis (VZV), and encephalitis (HSV, JC virus). Apart from specific clinical syndromes, viral reactivation has collateral immune effects including further immunosuppression, that predisposes to the emergence of other opportunistic infections as well as an upsurge of cancer risk (117–120). Improvement in graft function and inhibition of allograft rejection by CMV prophylaxis and antiviral treatment provides evidence for a possible CMV implication (121). Although the exact immunologic mechanisms of this process remain unknown, it has been suggested that heterologous immunity via CMV-specific T cells producing proinflammatory cytokines as well as MHC class II and adhesion molecules upregulation leading to increased alloimmunity, may explain the association between CMV infection and graft rejection (122–124). Implemented strategies for the prevention of viral reactivation in this population include universal prophylaxis with valganciclovir or ganciclovir for patients at risk for CMV reactivation (seropositive recipients or recipients of seropositive donors) (125, 126). The type of the organ transplantation and the institutional policies determine the duration of therapy. However, late CMV infection can be manifested after discontinuation of prophylaxis (127, 128). Extending prophylaxis or a pre-emptive approach with viral load monitoring are possible ways to overcome this problem (115, 129). Patients not on CMV prophylaxis should receive HSV and VZV prophylaxis for the first 3–6 months and at any point of immunosuppression increments. Since no effective antiviral therapy exists for EBV, monitoring of high-risk patients (e.g., seronegative recipients) at predetermined intervals is the approach of choice.
Human Immunodeficiency Virus Associated Viral Reactivations
Members of the Herpesviridae family, including CMV, EBV, and HSV, may reactivate causing various clinical syndromes in people living with HIV (PLWH). The presence of positive heterophile antibody tests in the setting of acute retroviral syndrome suggests a possible reactivation of EBV (130). Late presenters with a CD4+ count <50 cells/μL are at high risk of CMV-related disease, more commonly retinitis or encephalitis (131, 132). HSV co-infected patients exhibit increased frequency of HSV reactivation, mostly with atypical symptoms (133, 134). Unusual manifestations include esophagitis, tracheitis, meningoencephalitis, hepatitis, pneumonitis, retinal necrosis and disseminated disease (135). Reactivation of HSV in PLWH alters the natural history of HIV infection, with more efficient sexual transmission and increased rate of viral replication, during clinical or subclinical HSV reactivation (136). JC poliomavirus is implicated in the development of progressive multifocal leukoencephalopathy (PML) and possibly in various human neoplasms, in people with Acquired Immune Deficiency Syndrome (AIDS) or other severe immunosuppressive status (137).
Hepatitis B Virus Reactivation in Immunosuppressed Patients
After HBV infection, the viral DNA remains in hepatic cells of all patients irrespectively of serological recovery. The host's immune response plays a vital role in controlling viral replication (138). Critically ill patients with a history of HBV infection that are going to receive aggressive immunosuppression for their underlying diseases are at risk of HBV reactivation with grievous outcomes. Screening for HBV infection with HBV surface antigen (HBsAg) and HBV core antibody (anti-HBc) must be obtained before the initiation of immunosuppression therapy (including prednisone monotherapy ≥20 mg/day for more than 4 weeks) (139–141). The patients are risk-stratified based on the status of HBsAg and the type of immunosuppression (141). HBV reactivation may range from asymptomatic, with the only sign being a rise in HBV DNA level, to overt clinical syndrome with deranged aminotransferases and/or fulminant hepatitis (139).
Other Viral Pathogens Reactivated in Immunosuppressed Patients
Adenoviruses can exhibit a variety of clinical syndromes in immunocompromised hosts (142). The concept of adenoviral persistence leading to a subclinical state of infection has only recently been reported (143). In vitro studies have shown that reduced interferon levels promote viral reactivation (144). There is sufficient evidence showing that viral reactivation of endogenous adenoviruses is the major route of infection acquisition in HSCTRs and SOTRs (145). HSCTRs can present with a wide array of organ-specific adenoviral disease, including pneumonia, colitis, hepatitis, hemorrhagic cystitis, encephalitis, and disseminated disease (146). Adenoviral disease in SOTRs involves more commonly the donor organ, thus generating organ-related clinical manifestations (147). Since few data are available for effective antiviral agents against adenoviruses, contact, and droplet precautions remain the mainstay of infection control in the population of critical care transplanted patients (111).
Diagnosis
Pathogen Detection Techniques
Diagnosis of viral infections cannot be solely based on symptoms and signs, especially among critically ill patients who may have atypical clinical presentations. Laboratory-based techniques like viral cultures, electron microscopy and serology have been largely substituted by nucleic acid amplification tests (NAATs), sequencing (including next generation sequencing) and antigen detection methods, due to their increased accuracy and faster turnaround times (148, 149). Many commercially available rapid tests using nucleic acid amplification or antigen-based techniques are currently available for respiratory viruses (148, 150, 151). NAATs have advanced the sensitivity and specificity of influenza detection, as well as the identification of mutant variants that may be associated with increased disease severity, like the NA V263I and NS1 K196E for the influenza A H3N2 strain and the D222G for the influenza H1N1 pandemic strain 2009 (1, 152–154). Similarly, the diagnosis of SARS-CoV-2 infection is based on real time reverse transcription polymerase chain reaction (rRT-PCR) in respiratory tract specimens, although sensitivity of rRT-PCR is not 100% (155). Many factors may jeopardize the accuracy of the currently utilized SARS-CoV-2 NAATs, like inappropriate specimen collection techniques, handling of specimens, presence of interfering substances or antiviral treatment, manual errors, sample contamination, and testing outside the diagnostic window; all these factors should be considered when interpreting a negative result, especially during an ongoing pandemic (156). Although novel techniques have advanced our capacity to detect viruses, one should keep in mind that detection does not always correlate with pathogenicity (157). Results of such tests should guide clinical decisions in the context of a compatible clinical syndrome. Although antigen-based diagnostics for SARS-CoV-2 are generally less sensitive than molecular techniques, their utilization is rising globally, as they are usually less expensive than NAATs and provide results within minutes of sample acquisition (158).
Diagnosis of central nervous system (CNS) infections is typically made by detecting the viral genome in cerebrospinal fluid (CSF) by PCR, which yields high sensitivity and specificity rates (159). False negative results may occur early in the course of the infection (<72 h), in which case it is prudent to repeat PCR a few days later (159). Intrathecal serology may complement nucleic acid detection in some viruses like in VZV and arboviral CNS infection (159, 160).
Imaging Modalities
Imaging studies in viral pneumonias have low specificity compared to molecular tests, but can complement and guide both diagnosis and management of critically ill patients. Distinct patterns of chest computed tomography (CT) scan, may distinguish between some viral families that tend to induce specific radiological patterns (161). For SARS-CoV-2-induced pneumonia specifically, chest CT was found sensitive and moderately specific for the diagnosis of COVID-19 in patients with suspected infection, thus offering limited capacity of distinguishing between SARS-CoV-2 infection and other causes of acute lung injury (162).
Brain imaging may be highly characteristic in certain viral CNS infections. High signal on T2-weighted and fluid-attenuated inversion recovery (FLAIR) sequences in temporal lobes, especially when asymmetrical, are highly characteristic magnetic resonance imaging (MRI) findings of HSV encephalitis (163). MRI findings in arboviruses are not specific, but may show a predilection for deep gray matter (basal ganglia) and the brainstem (160).
Viral Clinical Syndromes in Critically Ill
Respiratory Infections
Clinical manifestations of viruses causing severe respiratory infections may range from asymptomatic or mild upper RTI to severe pneumonia with respiratory failure, septic shock-like syndrome, multi-organ dysfunction, and numerous other complications (Table 3), depending on both viral and host factors. The WHO has designated the terms of influenza-like illness (ILI) and severe acute respiratory infection (SARI) to embrace the typical presentation of viral pneumonias (188). Since there are no major differences in the initial management of most viral pneumonias (especially those who not have a specific treatment) these umbrella-terms allow healthcare professionals to quickly identify and manage these patients accordingly.
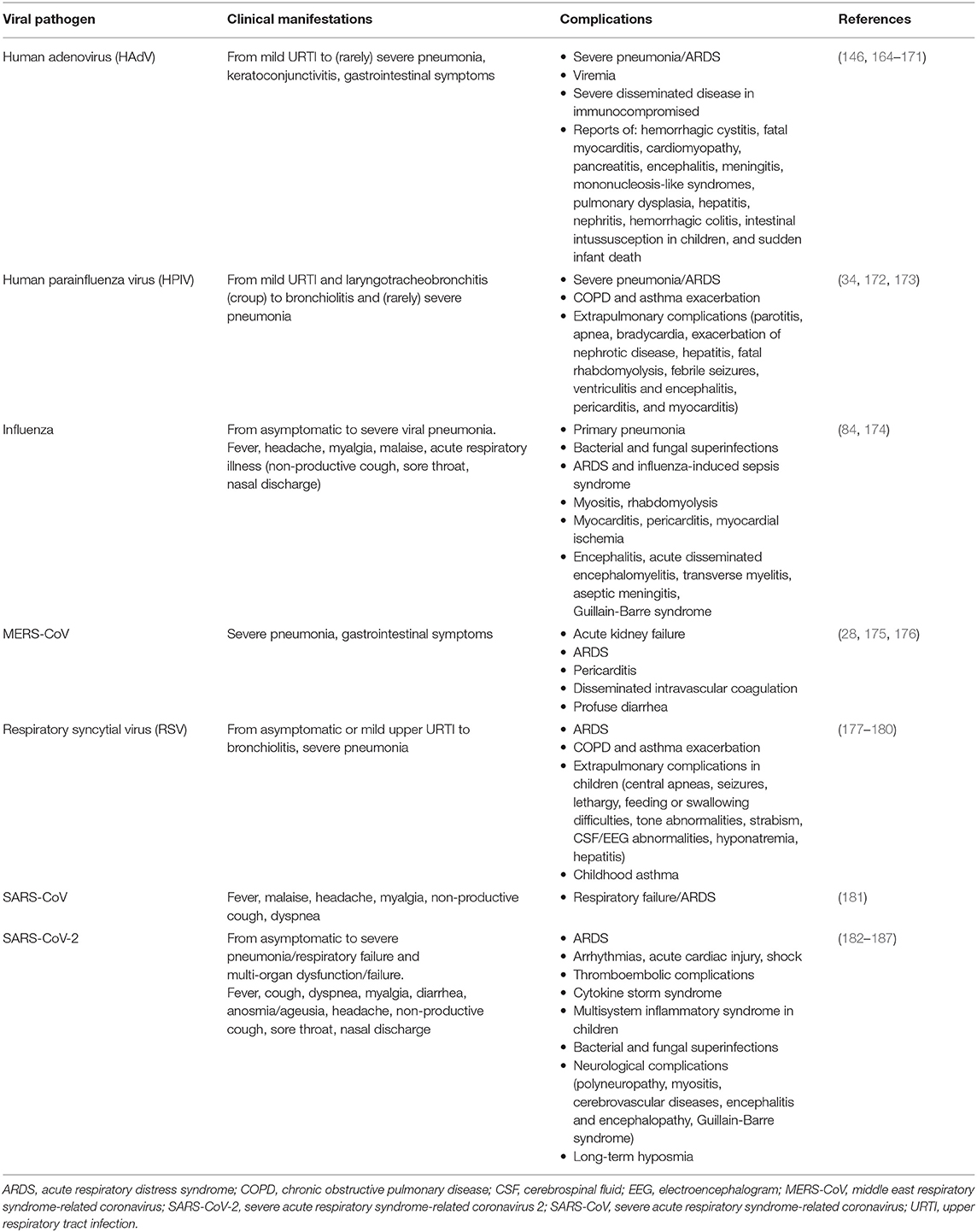
Table 3. Clinical manifestations and complications of viruses causing severe respiratory infections.
Central and Peripheral Nervous System Infections
A wide array of viral pathogens causes encephalitis (Table 4). In affected patients, a detailed travel, exposure and sexual history should be obtained, along with meticulous clinical examination for rashes and/or focal neurologic deficits that could suggest a certain viral pathogen (e.g., HSV). Geographic and epidemiologic clues are frequently the only suggestive features of a specific viral pathogen (Figure 1 and Table 1). Examples include current mosquito activity for arboviral encephalitis, regions with high tick population for tick-borne encephalitides and animal bite wounds for rabies. HSV-1 is the most common cause of sporadic viral encephalitis (189). Prompt and accurate diagnosis of HSV CNS infection is crucial as the timely initiation of targeted treatment has a significant impact on the outcome.
Fever, headache, lethargy and clinical signs of meningismus are features of aseptic meningitis. Abnormal sensorium is the hallmark of encephalitis, and although not often, signs of both entities can be found concurrently leading to the diagnosis of meningoencephalitis. Viral encephalitis and meningoencephalitis frequently lead to altered mental status and decreased level of consciousness that warrants intubation and management in ICU. Acute disseminated encephalomyelitis (ADEM) is a post-infectious sequela of viral encephalitis, precipitated by numerous possible viruses that may necessitate the need of critical care due to coma, seizures, or tetraplegia (190). The absence of viral detection along with histological findings of perivascular inflammation and demyelination suggest an immune-mediated disease (191). Other viral illnesses, not necessarily confined in the brain, can produce post-viral neurologic syndromes with prominent clinical manifestation from the CNS leading to severe morbidity and mortality (Table 5).
Shock and Multi-Organ Failure
Viral Hemorrhagic Fever
VHF is a group of syndromes that involve fever and hemorrhage of varying severity, caused by four distinct families of RNA viruses: the Arenaviridae family, the Bunyaviridae family, the Filoviridae family, and the Flaviviridae family [Table 6; (192)]. Each virus within these families has a distinct geographical distribution as shown in Figure 1. VHF represents the most severe and often fatal form of the infection by these pathogens, with a variable frequency among other clinical syndromes (192). It is characterized by fever, malaise, increased vascular permeability, reduced intravascular volume, coagulopathy and hemorrhagic features (193, 194). As these viruses do not use the same replication strategies inside the hosts, it is unclear how they concur to similar clinical syndromes (195). However, the brisk induction of pro-inflammatory cytokine expression and the fluid distribution imbalance due to (micro)vascular endothelium injury leading to capillary leak and disseminated intravascular coagulation seem to be common pathways leading to VHF development and often to multi-organ failure and death for most of the culprit viruses (195–197).
Hyperacute and Acute Liver Failure Due to Viral Hepatitis
Acute liver failure (ALF) is defined by the European Association for the Study of the Liver as a rare syndrome characterized by liver injury (elevated serum aminotransferases 2–3 times above the upper limit of normal), impaired liver function (jaundice and coagulopathy with international normalized ratio of >1.5) and hepatic encephalopathy developing within 7 days for hyperacute liver failure (HALF) and 8–28 days for ALF, in patients without pre-existent liver disease (198). Hepatitis E virus (HEV), hepatitis HBV and hepatitis A virus (HAV) are the most common viral pathogens of HALF/ALF, whereas other rare viral causes include CMV, VZV, HSV, and Dengue virus (Table 6) (198). The mortality rate of HEV-induced HALF is generally low, but specific HEV genotypes and patient groups (like the immunocompromised, the elderly, the pregnant women and those with underlying chronic liver diseases) are associated with significant mortality (198, 199). HAV may cause hyperacute ALF, but generally in <1% of the infected, with elderly being at higher risk for HAV-induced HALF and poor survival (200). ALF is a life-threatening condition caused by distributive shock due to generalized vasodilation; electrolyte and metabolic imbalance along with organ hypoperfusion lead to multi-organ dysfunction (198). Therefore, treatment requires admission to critical care settings, close monitoring, tight fluid management, neuroprotective measures, and preparedness for emergency transplantation (198).
Treatment
General Preventive Measures
Infection control measures are of paramount importance for prevention of viral infection transmission, especially within hospital settings. Modes of viral transmission can be either direct or indirect depending on the viral strain: direct transmission occurs through contact with infectious materials such as skin, secretions, blood, body fluids, contaminated surfaces and materials, etc. as well as via large droplets (diameter >5 μm), while indirect modes involve transmission through suspended air particles (airborne transmission of droplet nuclei, <5 μm) (201). Typically, droplet and contact precaution measures (for example in influenza and SARS-CoV-2 cases) involve patient isolation, source control (e.g., placement of face mask in non-intubated patients) and donning of level two personal protective equipment (PPE) (202, 203). Patients with airborne viruses such as measles and VZV require isolation in a specialized airborne infection isolation room and source control (as above); furthermore, donning of a level 3 PPE is prerequisite for contacting patients infected with airborne viruses, as well as when aerosol producing procedures are performed in patients with other respiratory viruses like influenza, SARS-CoV-2, and MERS-CoV (202). Ebola and other VHF pathogens are contracted via direct contact with patient's body fluids, contaminated medical supplies and equipment, or contaminated environmental surfaces (204). Therefore, although most blood-borne and contact-transmitted viruses do not require high level self-protective measures, donning of level 3 or higher PPE is essential when caring for patients with Ebola or other human-to-human transmitted VHF viruses (205). Imperatively, proper donning and doffing of PPE as well as meticulous hand hygiene are of paramount importance for infection control and reduction of in-hospital/in-ICU viral transmissions.
Treatment of CMV and HSV Reactivations in Immunocompetent Critically Ill
Although severe de novo viral infections necessitating ICU admission or viral reactivations in immunocompromised patients generally require treatment, provided that an effective therapy or management plan actually exists (Table 7), data on when and how to treat immunocompetent critically ill patients with viral reactivation are still inconclusive. To date, there is no evidence-based guidance for CMV or HSV reactivation treatment in critically ill immunocompetent patients. Regarding HSV reactivation (especially when it is clinically evident, e.g., in the oropharynx), treatment with aciclovir has been proposed in immunocompromised patients, not only because of cytotoxic therapies but also due to conditions such as extensive burns; moreover, treatment should probably be administered in patients with positive HSV test in respiratory samples who have evidence of pulmonary invasion or clinical deterioration that cannot be attributed to other etiology (206, 207). Besides clinical features and organ involvement-directed decision making, it has been proposed that patients with high viral loads (≥105 copies/mL) may also be entitled to treatment for HSV reactivation (208).
The effects of CMV reactivation on adverse clinical outcomes in immunocompetent critically patients have been associated with higher blood viral loads (208). Although no studies have robustly responded to whether and when CMV reactivation should be treated in this patient population (209), it has been suggested that therapy should be administered if CMV reactivation is associated with organ-involvement and/or if high viral loads are detected (≥103 copies/mL) (208).
Two randomized controlled trials assessing the use of prophylactic or preemptive treatment with aciclovir for HSV reactivation in mechanically ventilated patients, found that aciclovir administration did not improve clinical outcomes (207). As for HSV, two randomized controlled studies (the GRAIL-trial and the CCCC-trial) evaluating the efficacy of preemptive treatment for CMV reactivation in seropositive patients, failed to show benefit in IL-6 levels changes and in clinical outcomes, respectively (209–211). The GRAIL-trial, however, demonstrated that ganciclovir group had significantly more ventilator-free days in the sepsis subgroup analysis (209, 211). Based on expert-opinion, it has been suggested that preemptive treatment may be given in patients either at high-risk for HSV or CMV reactivation or in those who already have reactivation but at an early stage, before the advent of clinically evident infection (208). On the other hand, both aciclovir and ganciclovir have potentially serious adverse effects, thus the need for their administration in critically ill patients should be weighed against patient safety and cost-effectiveness (212).
Specific Treatments and Vaccines
Antivirals
The cornerstone of severe influenza management is the prompt initiation of antivirals (for at least 5 days, but a 10-days course is generally recommended in severe pneumonia) combined with high level of supportive care [Table 7; (213)]. The recommended antivirals are neuraminidase inhibitors (NAIs), i.e., oseltamivir, zanamivir, and peramivir. Baloxavir, a novel inhibitor of the cap-dependent endonuclease activity, is currently recommended only for uncomplicated influenza; adamantanes are no longer recommended due to development of resistance (214, 215). Although high-dose oseltamivir (300 mg/day) had been proposed for critically ill adults with severe pneumonia, no studies have proven its superiority against standard dose (150 mg/day) (216, 217). NAIs reduce mortality in severe influenza patients irrespective of the time of initiation, but early initiation (i.e., within 48 h of symptoms onset) reduces significantly the mortality (218). Oseltamivir and zanamivir may also be effective against selected avian influenza strains, but more studies are needed.
There are still no effective antivirals available for the MERS-CoV infection; however, two combinations [lopinavir/ritonavir/interferon beta (IFNβ) and remdesivir/IFNβ] are currently being tested in vivo and in animal models (219). Data from randomized controlled trials examining remdesivir in COVID-19 patients, show that remdesivir might be effective in reducing the time to recovery in hospitalized adults, and based on these trials it is currently the only antiviral agent that has gained authorization by the U.S. Food and Drug Administration (FDA) for use in hospitalized patients requiring supplemental oxygen (220–224). However, in November 2020, the WHO issued a conditional recommendation against the use of remdesivir in hospitalized patients irrespective of disease severity, due to the lack of sufficient data supporting that remdesivir actually improves clinical outcomes, including mortality (225). As evidence from current trials is still limited, robust data for safe and efficacious antivirals against SARS-CoV-2 infection are urgently required (226).
Targeted antiviral agents for other respiratory viruses are limited (Table 7). Ribavirin (inhaled or intravenous) and intravenous immunoglobulin have been used with conflicting results in cases of HPIV-3 pneumonia in severely immunocompromised patients (227). Although supportive care is the mainstay of treatment of RSV infection, inhaled (or oral) ribavirin can be used in patients with significant risk factors for severe disease, like transplantation (228). For HMPV infection, treatment is mainly supportive, although ribavirin, intravenous immunoglobulin, monoclonal antibodies, and fusion inhibitory peptides against specific viral proteins have been used in isolated cases or in experimental models (229–234). Cidofovir and its oral prodrug brincidofovir are administered in severe HAdV infections (164, 235–237).
In contrast to respiratory infections, targeted antivirals are available for major CNS pathogens like HSV and VZV viruses (Table 7). Indeed, the mainstay of treatment for HSV encephalitis is aciclovir (10 mg/kg intravenously every 8 h), though dose adjustments are required for impaired renal function. The duration of treatment ranges from 14 to 21 days, and should be administered as early as possible. Similarly, treatment of choice for severe VZV CNS infection is intravenous aciclovir for 14–21 days, but in higher doses than those administered in HSV encephalitis [i.e., up to 15 mg/kg three times a day; (238)]. Of note, aciclovir can cause crystal nephropathy and acute renal failure in 12–49% of patients, with those in ICU being at greater risk (239). Treatment of CMV encephalitis consists of dual therapy with foscarnet and ganciclovir, as no survival benefit has been observed with either agent alone; duration of treatment is 3 weeks for immunocompetent patients and 6 weeks for those with immunodeficiency (240). Secondary prophylaxis is required until immune restoration (CD4+ >100 cells/ml) in AIDS patients and antiretroviral treatment should start as soon as possible (241).
Finally, patients with severe acute HBV hepatitis should be treated with a nucleos(t)ide analog reverse-transcriptase inhibitor like entecavir or tenofovir, while ribavirin may be considered for severe acute HEV hepatitis (242, 243).
Corticosteroids
Corticosteroids have been used in various severe viral infections as immunomodulators abrogating the cytokine-release syndrome effects, with conflicting outcomes and results. In severe influenza, corticosteroids are associated with unfavorable outcomes like increased mortality and prolonged viral shedding as well as increased incidence of bacterial superinfections and possibly prolonged duration of mechanical ventilation (78, 244); thus, their administration is not recommended in severe flu (213).
In contrast to influenza trials that failed to show benefit in mortality and improved outcomes, the Randomized Evaluation of COVid-19 thERapY (RECOVERY) trial for COVID-19 treatment reported that low dose dexamethasone (6 mg once per day either orally or by intravenous injection for 10 days) along with standard of care reduced significantly the incidence of death among mechanically ventilated patients (29.3 vs. 41.4%; rate ratio, 0.64; 95% CI, 0.51–0.81) and among those on supplemental oxygen (23.3 vs. 26.2%; rate ratio, 0.82; 95% CI, 0.72–0.94) compared to those who received standard treatment, thus being the first agent that was found to reduce mortality in moderate, severe and critical COVID-19 cases (245). However, no mortality benefit was found in patients not requiring oxygen support (245). Additionally, the CoDEX trial found that among patients with moderate and severe COVID-19-associated ARDS, the intravenous administration of dexamethasone (in higher doses than RECOVERY trial) resulted in a statistically significant increase of the number of ventilator-free days over 28 days (246). Nonetheless, randomized controlled trials using other corticosteroids, such as methylprednisolone and hydrocortisone, failed to show similar survival benefits (247, 248). As of January 2021, dexamethasone is the only therapeutic agent being strongly recommended for COVID-19 patients requiring (non-invasive) supplemental oxygen or mechanical ventilation by the international stakeholders (220, 249). In other viral infections, corticosteroids are used only for certain indications, like in HPIV-induced croup to reduce airway edema or in VZV-induced CNS vasculopathy, whereby a short course of high-dose corticosteroids is recommended (46). The use of corticosteroids in HSV encephalitis is debatable, but it may be useful in selected cases with vasogenic edema and mass effect (46). Intravenous immunoglobulin, and in refractory cases methylprednisolone, have been used in refractory cases of enteroviral encephalitis (54, 250).
Blood-Derived Products and Cell Therapies
Passive immunization with antibodies purified from pooled sera, convalescent plasma and hyperimmune intravenous immunoglobulin has been used as a last resort measure in severe viral infections, when other treatment options have failed. Hyperimmune intravenous immunoglobulin from convalescent plasma was associated with reduced mortality in severe A/H1N1pdm09 infection in a randomized controlled trial, but its use is not universally recommended yet (251).
Results are pending for the safety and efficacy of convalescent plasma, SARS-CoV-2 immunoglobulin, non-SASRS-CoV-2 intravenous immunoglobulin, and mesenchymal stem cell therapies in COVID-19 infection; due to the lack of sufficient evidence, these agents are either non-recommended outside the context of clinical trials or have yet to receive no specific recommendations for or against their use (226, 252).
Finally, while treatment is mainly supportive in the WNV infection, intravenous immunoglobulin—either conventional or from donors with anti-WNV antibodies—has been used successfully in some cases, but there is no universal recommendation as yet (160).
Immunotherapies
As pathogenesis of severe and critical COVID-19 disease has been linked primarily to cytokine storm syndrome, many research efforts are made with trials testing various immunomodulatory agents like tocilizumab, sarilumab, anakinra, Janus kinase (JAK) inhibitors, adalimumab, anti-programmed cell death protein 1 monoclonal antibodies, bevacizumab, ixekizumab, eculizumab, human recombinant IL-2, inhibitors of NLRP3 inflammasome activation, Janus kinase inhibitors, fingolimod, a recombinant fusion protein targeting an immune pathway checkpoint (CD24Fc), leflunomide, thalidomide, and colchicine (226). Baricitinib, a JAK 1 and 2 selective inhibitor, is approved by the FDA for emergency use in combination with remdesivir in hospitalized COVID-19 patients aged ≥2 years old who require supplemental oxygen, invasive mechanical ventilation or extracorporeal membrane oxygenation (253). Additionally, three anti-SARS-CoV-2 monoclonal antibodies (bamlanivimab and the combination of casirivimab plus imdevimab) have received authorization by the FDA for emergency use in outpatients with COVID-19 who are at risk for disease progression (220).
Palivizumab (15 mg/kg intramuscularly per month), a monoclonal anti-RSV antibody, has substituted immunoglobulin products with high anti-RSV antibody titers (RSV-IGIV) in the prevention of RSV infection in high-risk infants (254, 255). Additionally, various monoclonal antibodies against other viruses like CMV, Influenza, HIV, Ebola and Rabies, are currently under development (256).
Vaccinations
Vaccines are the most important preventative measures for many serious viral infections (Table 7). Annual vaccination is of utmost importance for influenza prevention and is recommended for individuals over the age of 6 months without contraindications (257). Although several vaccines are under development for MERS-CoV, none has been licensed yet (258). On the contrary, immense research has led to the development of more than 240 vaccine candidates against SARS-CoV-2; as of January 2021, at least 8 effective SARS-CoV-2 vaccines have been officially approved in numerous countries worldwide (259). Besides the unparalleled speed of vaccine development (<1 year after the onset of COVID-19 pandemic), it is also quite impressive that this pandemic incited the utilization of next generation vaccine platforms, such as the messenger RNA and non-replicating viral vector-based vaccines, that have already been launched for public use (259, 260).
Live oral vaccines are highly effective in reducing the risk of respiratory HAdV infection, but are available only within the U.S. military settings (261). Zoster vaccination in adults has been associated with reduced incidence of herpes zoster, but the data for its efficacy in preventing CNS reactivation and complications are limited (262). Finally, post-exposure prophylaxis with rabies vaccine and immunoglobulin can prevent rabies in almost 100% of the exposed individuals (263, 264).
Conclusion
Viral infections are important determinants of adverse outcomes especially among critically ill patients. Their clinical significance as pathogens, although largely disregarded in the past, is now increasingly recognized especially due to the unprecedented COVID-19 pandemic. The advent of more sensitive and rapid molecular tools has changed the landscape in microbiological diagnosis and in identification of fastidious pathogens, including viruses that are increasingly isolated among critically ill patients. The interplay between direct viral organ evasion and the effects of the hosts' immune system dysregulation defines the clinical severity and the outcomes of viral infections. As effective therapies are currently lacking for the majority of viruses causing severe infections, more clinical trials are urgently needed to determine whether novel antivirals, steroids, or immunomodulators can impact better clinical outcomes in viral infections in the ICU.
Author Contributions
PF, EK, and CM contributed in the collection of the data, wrote parts the first draft, and the subsequent revisions of the manuscript. TK and ST critically revised and corrected the manuscript. All authors read and approved the submitted manuscript version.
Funding
PF: Doctorate scholarship by the State Scholarships Foundation (IKY), Partnership Agreement (PA) 2014–2020, co-financed by Greece and the European Union (European Social Fund—ESF) through the Operational Program Human Resources Development, Education and Lifelong Learning 2014–2020.
Conflict of Interest
The authors declare that the research was conducted in the absence of any commercial or financial relationships that could be construed as a potential conflict of interest.
Acknowledgments
The authors would like to express their gratitude to Mr. Stefanos Totis for his contribution in designing and processing the Figures.
References
1. Piralla A, Rovida F, Girello A, Premoli M, Mojoli F, Belliato M, et al. Frequency of respiratory virus infections and next-generation analysis of influenza A/H1N1pdm09 dynamics in the lower respiratory tract of patients admitted to the ICU. PLoS ONE. (2017) 12:e0178926. doi: 10.1371/journal.pone.0178926
2. Cameron RJ, De Wit D, Welsh TN, Ferguson J, Grissell TV, Rye PJ. Virus infection in exacerbations of chronic obstructive pulmonary disease requiring ventilation. Intensive Care Med. (2006) 32:1022–9. doi: 10.1007/s00134-006-0202-x
3. Kelesidis T, Mastoris I, Metsini A, Tsiodras S. How to approach and treat viral infections in ICU patients. BMC Infect Dis. (2014) 14:321. doi: 10.1186/1471-2334-14-321
4. Nguyen C, Kaku S, Tutera D, Kuschner WG, Barr J. Viral respiratory infections of adults in the intensive care unit. J Intensive Care Med. (2016) 31:427–41. doi: 10.1177/0885066615585944
5. Schnell D, Gits-Muselli M, Canet E, Lemiale V, Schlemmer B, Simon F, et al. Burden of respiratory viruses in patients with acute respiratory failure. J Med Virol. (2014) 86:1198–202. doi: 10.1002/jmv.23760
6. Walter JM, Wunderink RG. Severe respiratory viral infections: new evidence and changing paradigms. Infect Dis Clin North Am. (2017) 31:455–74. doi: 10.1016/j.idc.2017.05.004
7. Karhu J, Ala-Kokko TI, Vuorinen T, Ohtonen P, Syrjälä H. Lower respiratory tract virus findings in mechanically ventilated patients with severe community-acquired pneumonia | clinical infectious diseases | Oxford Academic. Clin Infect Dis. (2014) 59:62–70. doi: 10.1093/cid/ciu237
8. Loubet P, Voiriot G, Houhou-Fidouh N, Neuville M, Bouadma L, Lescure FX, et al. Impact of respiratory viruses in hospital-acquired pneumonia in the intensive care unit: a single-center retrospective study. J Clin Virol. (2017) 91:52–7. doi: 10.1016/j.jcv.2017.04.001
9. Micek ST, Chew B, Hampton N, Kollef MH. A case-control study assessing the impact of nonventilated hospital-acquired pneumonia on patient outcomes. Chest. (2016) 150:1008–14. doi: 10.1016/j.chest.2016.04.009
10. Kim ES, Park KU, Lee SH, Lee YJ, Park JS, Cho YJ, et al. Comparison of viral infection in healthcare-associated pneumonia (HCAP) and community-acquired pneumonia (CAP). PLoS ONE. (2018) 13:e0192893. doi: 10.1371/journal.pone.0192893
11. Shorr AF, Zilberberg MD. Going viral: importance of viral pathogens in nonventilated hospital-acquired pneumonia. Chest. (2016) 150:991–2. doi: 10.1016/j.chest.2016.05.028
12. Vaideeswar P, Bavdekar SB, Biswas P, Sarangarajan R, Bhosale A. Viral ventilator-associated pneumonia: uncovering tip of the iceberg. Indian J Pathol Microbiol. (2011) 54:339–43. doi: 10.4103/0377-4929.81633
13. Hong H-L, Hong S-B, Ko G-B, Huh JW, Sung H, Do K-H, et al. Viral infection is not uncommon in adult patients with severe hospital-acquired pneumonia. PLoS ONE. (2014) 9:e95865. doi: 10.1371/journal.pone.0095865
14. World Health Organization (WHO). Influenza (Seasonal). (2018). Available online at: https://www.who.int/news-room/fact-sheets/detail/influenza-(seasonal) (accessed June 23, 2020).
15. Iuliano AD, Roguski KM, Chang HH, Muscatello DJ, Palekar R, Tempia S, et al. Estimates of global seasonal influenza-associated respiratory mortality: a modelling study. Lancet. (2018) 391:1285–300. doi: 10.1016/S0140-6736(17)33293-2
16. Centers for Disease Control Prevention (CDC). Disease Burden of Influenza | CDC. Available online at: https://www.cdc.gov/flu/about/burden/index.html (accessed January 10, 2021).
17. Reed C, Chaves SS, Kirley PD, Emerson R, Aragon D, Hancock EB, et al. Estimating influenza disease burden from population-based surveillance data in the United States. PLoS ONE. (2015) 10:e0118369. doi: 10.1371/journal.pone.0118369
18. Gaunt ER, Hardie A, Claas ECJ, Simmonds P, Templeton KE. Epidemiology and clinical presentations of the four human coronaviruses 229E, HKU1, NL63, and OC43 detected over 3 years using a novel multiplex real-time PCR method. J Clin Microbiol. (2010) 48:2940–7. doi: 10.1128/JCM.00636-10
19. Nickbakhsh S, Thorburn F, Von Wissmann B, McMenamin J, Gunson RN, Murcia PR. Extensive multiplex PCR diagnostics reveal new insights into the epidemiology of viral respiratory infections. Epidemiol Infect. (2016) 144:2064–76. doi: 10.1017/S0950268816000339
20. de Groot RJ, Baker SC, Baric RS, Brown CS, Drosten C, Enjuanes L, et al. Middle east respiratory syndrome coronavirus (MERS-CoV): announcement of the coronavirus study group. J Virol. (2013) 87:7790–2. doi: 10.1128/jvi.01244-13
21. Peiris JSM, Yuen KY, Osterhaus ADME, Stöhr K. The severe acute respiratory syndrome. N Engl J Med. (2003) 349:2431–41. doi: 10.1056/NEJMra032498
22. Zaki AM, Van Boheemen S, Bestebroer TM, Osterhaus ADME, Fouchier RAM. Isolation of a novel coronavirus from a man with pneumonia in Saudi Arabia. N Engl J Med. (2012) 367:1814–20. doi: 10.1056/NEJMoa1211721
23. World Health Organization (WHO). COVID-19 Situation Reports. Available online at: https://www.who.int/emergencies/diseases/novel-coronavirus-2019/situation-reports/ (accessed January 9, 2021).
24. Centers For Disease Control and Prevention (CDC). Estimated Disease Burden of COVID-19 | CDC. (2020). Available online at: https://www.cdc.gov/coronavirus/2019-ncov/cases-updates/burden.html (accessed January 10, 2021).
25. Our World in Data. Mortality Risk of COVID-19. (2020). Available online at: https://ourworldindata.org/mortality-risk-covid (accessed January 10, 2021).
26. Our World in Data. Number of COVID-19 Patients in ICU Per Million. (2020). Available online at: https://ourworldindata.org/grapher/covid-icu-patients-per-million?tab=chart&stackMode=absolute&time=2020-03-12.latest (accessed January 10, 2021).
27. Paules CI, Marston HD, Fauci AS. Coronavirus infections-more than just the common cold. J Am Med Assoc. (2020) 323:707–8. doi: 10.1001/jama.2020.0757
28. De Wit E, Van Doremalen N, Falzarano D, Munster VJ. SARS and MERS: recent insights into emerging coronaviruses. Nat Rev Microbiol. (2016) 14:523–34. doi: 10.1038/nrmicro.2016.81
29. World Health Organization (WHO). Middle East Respiratory Syndrome Coronavirus (MERS-CoV). Available online at: https://www.who.int/health-topics/middle-east-respiratory-syndrome-coronavirus-mers#tab=tab_1 (accessed January 10, 2021).
30. Branche AR, Falsey AR. Parainfluenza virus infection. Semin Respir Crit Care Med. (2016) 37:538–54. doi: 10.1055/s-0036-1584798
31. Gilca R, Amini R, Douville-Fradet M, Charest H, Dubuque J, Boulianne N, et al. Other respiratory viruses are important contributors to adult respiratory hospitalizations and mortality even during peak weeks of the influenza season. Open forum Infect Dis. (2014) 1:ofu086. doi: 10.1093/ofid/ofu086
32. Johnstone J, Majumdar SR, Fox JD, Marrie TJ. Viral infection in adults hospitalized with community-acquired pneumonia: prevalence, pathogens, and presentation. Chest. (2008) 134:1141–8. doi: 10.1378/chest.08-0888
33. Marcos MA, Camps M, Pumarola T, Martinez JA, Martinez E, Mensa J, et al. The role of viruses in the aetiology of community-acquired pneumonia in adults. Antivir Ther. (2006) 11:351–359.
34. Essa S, Al-Tawalah H, Alshamali S, Al-Nakib W. The potential influence of human parainfluenza viruses detected during hospitalization among critically ill patients in Kuwait, 2013-2015. Virol J. (2017) 14:19. doi: 10.1186/s12985-017-0681-0
35. Van Someren Gréve F, Juffermans NP, Bos LDJ, Binnekade JM, Braber A, Cremer OL, et al. Respiratory viruses in invasively ventilated critically ill patients-a prospective multicenter observational study. Crit Care Med. (2018) 46:29–36. doi: 10.1097/CCM.0000000000002752
36. To KKW, Lau SKP, Chan KH, Mok KY, Luk HKH, Yip CCY, et al. Pulmonary and extrapulmonary complications of human rhinovirus infection in critically ill patients. J Clin Virol. (2016) 77:85–91. doi: 10.1016/j.jcv.2016.02.014
37. McClure DL, Kieke BA, Sundaram ME, Simpson MD, Meece JK, Sifakis F, et al. Seasonal incidence of medically attended respiratory syncytial virus infection in a community cohort of adults ≥50 years old. PLoS ONE. (2014) 9:e102586. doi: 10.1371/journal.pone.0102586
38. Colosia AD, Yang J, Hillson E, Mauskopf J, Copley-Merriman C, Shinde V, et al. The epidemiology of medically attended respiratory syncytial virus in older adults in the United States: a systematic review. PLoS ONE. (2017) 12:e0182321. doi: 10.1371/journal.pone.0182321
39. Branche AR. Why making a diagnosis of respiratory syncytial virus should matter to clinicians. Clin Infect Dis. (2019) 69:204–6. doi: 10.1093/cid/ciy880
40. Shi T, Denouel A, Tietjen AK, Campbell I, Moran E, Li X, et al. Global disease burden estimates of respiratory syncytial virus-associated acute respiratory infection in older adults in 2015: a systematic review and meta-analysis. J Infect Dis. (2019) 222:S577–83. doi: 10.1093/infdis/jiz059
41. Thompson WW, Shay DK, Weintraub E, Cox N, Anderson LJ, Fukuda K. Mortality associated with influenza and respiratory syncytial virus in the United States. J Am Med Assoc. (2003) 289:179–86. doi: 10.1001/jama.289.2.179
42. Hasvold J, Sjoding M, Pohl K, Cooke CR, Hyzy RC. The role of human metapneumovirus in the critically ill adult patient. J Crit Care. (2016) 31:233–7. doi: 10.1016/j.jcrc.2015.09.035
43. Walsh EE, Peterson DR, Falsey AR. Human metapneumovirus infections in adults: another piece of the puzzle. Arch Intern Med. (2008) 168:2489–96. doi: 10.1001/archinte.168.22.2489
44. Vidaur L, Totorika I, Montes M, Vicente D, Rello J, Cilla G. Human metapneumovirus as cause of severe community-acquired pneumonia in adults: insights from a ten-year molecular and epidemiological analysis. Ann Intensive Care. (2019) 9:86. doi: 10.1186/s13613-019-0559-y
45. Loubet P, Mathieu P, Lenzi N, Galtier F, Lainé F, Lesieur Z, et al. Characteristics of human metapneumovirus infection in adults hospitalized for community-acquired influenza-like illness in France, 2012–2018: a retrospective observational study. Clin Microbiol Infect. (2020) 27:127.e1–27.e6. doi: 10.1016/j.cmi.2020.04.005
46. Kramer AH. Viral encephalitis in the ICU. Crit Care Clin. (2013) 29:621–49. doi: 10.1016/j.ccc.2013.03.011
47. Ramos-Estebanez C, Lizarraga KJ, Merenda A. A systematic review on the role of adjunctive corticosteroids in herpes simplex virus encephalitis: is timing critical for safety and efficacy? Antivir Ther. (2014) 19:133–9. doi: 10.3851/IMP2683
48. Jouan Y, Grammatico-Guillon L, Espitalier F, Cazals X, François P, Guillon A. Long-term outcome of severe herpes simplex encephalitis: a population-based observational study. Crit Care. (2015) 19:345. doi: 10.1186/s13054-015-1046-y
49. Hjalmarsson A, Blomqvist P, Sköldenberg B. Herpes simplex encephalitis in Sweden, 1990-2001: Incidence, morbidity, and mortality. Clin Infect Dis. (2007) 45:875–80. doi: 10.1086/521262
50. Lizzi J, Hill T, Jakubowski J. Varicella zoster virus encephalitis. Clin Pract Cases Emerg Med. (2019) 3:380–2. doi: 10.5811/cpcem.2019.8.43010
51. Herlin LK, Hansen KS, Bodilsen J, Larsen L, Brandt C, Andersen CØ, et al. Varicella zoster virus encephalitis in Denmark from 2015 to 2019—a Nationwide Prospective Cohort study. Clin Infect Dis. (2020) ciaa185. doi: 10.1093/cid/ciaa185. [Epub ahead of print].
52. Wang T, Winkelmann ER, Luo H. West Nile virus infection in the central nervous system. F1000Research. (2016) 5:105. doi: 10.12688/f1000research.7404.1
53. Wasay M, Khatri IA, Abd-Allah F. Arbovirus infections of the nervous system: current trends and future threats. Neurology. (2015) 84:421–3. doi: 10.1212/WNL.0000000000001177
54. Messacar K, Spence-Davizon E, Osborne C, Press C, Schreiner TL, Martin J, et al. Clinical characteristics of enterovirus A71 neurological disease during an outbreak in children in Colorado, USA, in 2018: an observational cohort study. Lancet Infect Dis. (2020) 20:230–9. doi: 10.1016/S1473-3099(19)30632-2
55. McKay SL, Lee AD, Lopez AS, Nix WA, Dooling KL, Keaton AA, et al. Increase in acute flaccid myelitis — United States, 2018. MMWR Morb Mortal Wkly Rep. (2018) 67:1273–5. doi: 10.15585/mmwr.mm6745e1
56. Guerra JA, Waters A, Kelly A, Morley U, O'Reilly P, O'Kelly E, et al. Seroepidemiological and phylogenetic characterization of neurotropic enteroviruses in Ireland, 2005-2014. J Med Virol. (2017) 89:1550–8. doi: 10.1002/jmv.24765
57. Greninger AL, Naccache SN, Messacar K, Clayton A, Yu G, Somasekar S, et al. A novel outbreak enterovirus D68 strain associated with acute flaccid myelitis cases in the USA (2012-14): a retrospective cohort study. Lancet Infect Dis. (2015) 15:671–82. doi: 10.1016/S1473-3099(15)70093-9
58. Suresh S, Rawlinson WD, Andrews PI, Stelzer-Braid S. Global epidemiology of nonpolio enteroviruses causing severe neurological complications: a systematic review and meta-analysis. Rev Med Virol. (2020) 30:e2082. doi: 10.1002/rmv.2082
59. World Health Organization (WHO). Ebola Virus Disease. (2020). Available online at: https://www.who.int/news-room/fact-sheets/detail/ebola-virus-disease (accessed January 10, 2021).
60. World Health Organization (WHO). Marburg Virus Disease. (2018). Available online at: https://www.who.int/news-room/fact-sheets/detail/marburg-virus-disease (accessed January 10, 2021).
61. World Health Orginization (WHO). Dengue and Severe Dengue. (2020). Available online at: https://www.who.int/news-room/fact-sheets/detail/dengue-and-severe-dengue (accessed January 10, 2021).
62. World Health Organization (WHO). WHO | Media Centre, Dengue and Severe Dengue. (2017). Available online at: https://apps.who.int/mediacentre/factsheets/fs117/en/index.html (accessed January 10, 2021).
63. World Health Organization (WHO). Lassa Fever. (2017). Available online at: https://www.who.int/en/news-room/fact-sheets/detail/lassa-fever (accessed January 10, 2021).
64. Tian H, Stenseth NC. The ecological dynamics of hantavirus diseases: from environmental variability to disease prevention largely based on data from China. PLoS Negl Trop Dis. (2019) 13:e0006901. doi: 10.1371/journal.pntd.0006901
65. Centers for Disease Control and Prevention (CDC). CDC - Hantavirus. Available online at: https://www.cdc.gov/hantavirus/index.html (accessed January 10, 2021).
66. MacNeil A, Nichol ST, Spiropoulou CF. Hantavirus pulmonary syndrome. Virus Res. (2011) 162:138–47. doi: 10.1016/j.virusres.2011.09.017
67. Avšič-Županc T, Saksida A, Korva M. Hantavirus infections. Clin Microbiol Infect. (2019) 21:e6–16. doi: 10.1111/1469-0691.12291
68. World Health Organization (WHO). Crimean-Congo Haemorrhagic Fever. (2013). Available online at: https://www.who.int/news-room/fact-sheets/detail/crimean-congo-haemorrhagic-fever (accessed January 10, 2021).
69. Iwasaki A, Pillai PS. Innate immunity to influenza virus infection. Nat Rev Immunol. (2014) 14:315–28. doi: 10.1038/nri3665
70. Ng CT, Sullivan BM, Oldstone MB. The role of dendritic cells in viral persistence. Curr Opin Virol. (2011) 1:160–6. doi: 10.1016/j.coviro.2011.05.006
71. Nakayama M. Antigen presentation by MHC-dressed cells. Front Immunol. (2014) 5:672. doi: 10.3389/fimmu.2014.00672
72. Mueller SN, Rouse BT. Immune responses to viruses. In: Rich R, Fleisher T, Shearer W, Schroeder H, Frew A, Weyand C, editors. Clinical Immunology. Amsterdam: Elsevier Ltd. (2008). p. 421–31. doi: 10.1016/B978-0-323-04404-2.10027-2
73. Nash AA, Dutia BM. The immune response to viral infections. In: Mahy BWJ, ter Meulen V, Borriello SP, Murray PR, Merz WG, Hay RJ, Cox FEG, Despommier DD, Kaufmann SHE, Steward MW, editors. Topley & Wilson's Microbiology and Microbial Infections. Chichester: John Wiley & Sons, Ltd. (2010). p. 385–91. doi: 10.1002/9780470688618.taw0220
74. Salvana EMT, Salata RA. Infectious complications associated with monoclonal antibodies and related small molecules. Clin Microbiol Rev. (2009) 22:274–90. doi: 10.1128/CMR.00040-08
75. Zhang Q, Frange P, Blanche S, Casanova JL. Pathogenesis of infections in HIV-infected individuals: insights from primary immunodeficiencies. Curr Opin Immunol. (2017) 48:122–33. doi: 10.1016/j.coi.2017.09.002
76. Hulme KD, Gallo LA, Short KR. Influenza virus and glycemic variability in diabetes: a killer combination? Front Microbiol. (2017) 8:861. doi: 10.3389/fmicb.2017.00861
77. Schaible UE, Kaufmann SHE. Malnutrition and infection: complex mechanisms and global impacts. PLoS Med. (2007) 4:0806–12. doi: 10.1371/journal.pmed.0040115
78. Hamers L, Kox M, Pickkers P. Sepsis-induced immunoparalysis: mechanisms, markers, and treatment options. Minerva Anestesiol. (2015) 81:426–39.
79. Coates BM, Staricha KL, Wiese KM, Ridge KM. Influenza a virus infection, innate immunity, and childhood. JAMA Pediatr. (2015) 169:956–3. doi: 10.1001/jamapediatrics.2015.1387
80. McElhaney JE, Kuchel GA, Zhou X, Swain SL, Haynes L. T-cell immunity to influenza in older adults: a pathophysiological framework for development of more effective vaccines. Front Immunol. (2016) 7:41. doi: 10.3389/fimmu.2016.00041
81. Fragkou PC, Torrance HD, Pearse RM, Ackland GL, Prowle JR, Owen HC, et al. Perioperative blood transfusion is associated with a gene transcription profile characteristic of immunosuppression: a prospective cohort study. Crit Care. (2014) 18:541. doi: 10.1186/s13054-014-0541-x
82. Oppong E, Cato ACB. Effects of glucocorticoids in the immune system. Adv Exp Med Biol. (2015) 872:217–33. doi: 10.1007/978-1-4939-2895-8_9
83. Honce R, Schultz-Cherry S. Impact of obesity on influenza A virus pathogenesis, immune response, and evolution. Front Immunol. (2019) 10:1071 doi: 10.3389/fimmu.2019.01071
84. Kalil AC, Thomas PG. Influenza virus-related critical illness: pathophysiology and epidemiology. Crit Care. (2019) 23:258. doi: 10.1186/s13054-019-2539-x
85. Centers for Disease Control Prevention (CDC). People at High Risk For Flu Complications | CDC. Available online at: https://www.cdc.gov/flu/highrisk/index.htm (accessed June 12, 2020).
86. Wolff D, Nee S, Hickey NS, Marschollek M. Risk factors for Covid-19 severity and fatality: a structured literature review. Infection. (2021) 49:15–28. doi: 10.1007/s15010-020-01509-1
87. Oh SJ, Lee JK, Shin OS. Aging and the immune system: the impact of immunosenescence on viral infection, immunity and vaccine immunogenicity. Immune Netw. (2019) 19:e37. doi: 10.4110/in.2019.19.e37
88. Rouse BT, Sehrawat S. Immunity and immunopathology to viruses: what decides the outcome? Nat Rev Immunol. (2010) 10:514–26. doi: 10.1038/nri2802
89. Meftahi GH, Jangravi Z, Sahraei H, Bahari Z. The possible pathophysiology mechanism of cytokine storm in elderly adults with COVID-19 infection: the contribution of “inflame-aging.” Inflamm Res. (2020) 69:825–39. doi: 10.1007/s00011-020-01372-8
90. Falagas ME, Kompoti M. Obesity and infection. Lancet Infect Dis. (2006) 6:438–46. doi: 10.1016/S1473-3099(06)70523-0
91. Tsatsanis C, Margioris AN, Kontoyiannis DP. Association between H1N1 infection severity and obesity—adiponectin as a potential etiologic factor. J Infect Dis. (2010) 202:459–60. doi: 10.1086/653842
92. Shorr AF, Jackson WL. Transfusion practice and nosocomial infection: assessing the evidence. Curr Opin Crit Care. (2005) 11:468–72. doi: 10.1097/01.ccx.0000176689.18433.f4
93. Michalopoulos A, Geroulanos S, Rosmarakis ES, Falagas ME. Frequency, characteristics, and predictors of microbiologically documented nosocomial infections after cardiac surgery. Eur J Cardiothora Surg. (2006) 29:456–60. doi: 10.1016/j.ejcts.2005.12.035
94. Taylor RW, O'Brien J, Trottier SJ, Manganaro L, Cytron M, Lesko MF, et al. Red blood cell transfusions and nosocomial infections in critically ill patients. Crit Care Med. (2006) 34:2302–8. doi: 10.1097/01.CCM.0000234034.51040.7F
95. Walton AH, Muenzer JT, Rasche D, Boomer JS, Sato B, Brownstein BH, et al. Reactivation of multiple viruses in patients with sepsis. PLoS ONE. (2014) 9:e98819. doi: 10.1371/journal.pone.0098819
96. Ljungman P, Griffiths P, Paya C. Definitions of cytomegalovirus infection and disease in transplant recipients. Clin Infect Dis. (2002) 34:1094–1097. doi: 10.1086/339329
97. López-Giraldo A, Sialer S, Esperatti M, Torres A. Viral-reactivated pneumonia during mechanical ventilation: is there need for antiviral treatment? Front Pharmacol. (2011) 2:66. doi: 10.3389/fphar.2011.00066
98. Limaye AP, Boeckh M. CMV in critically ill patients: pathogen or bystander? Rev Med Virol. (2010) 20:372–379. doi: 10.1002/rmv.664
99. Hummel M, Abecassis MM. A model for reactivation of CMV from latency. J Clin Virol. (2002) 25:123–36. doi: 10.1016/s1386-6532(02)00088-4
100. Boeckh M, Murphy WJ, Peggs KS. Recent advances in cytomegalovirus: an update on pharmacologic and cellular therapies. Biol Blood Marrow Transplant. (2015) 21:24–9. doi: 10.1016/j.bbmt.2014.11.002
101. Hotchkiss RS, Monneret G, Payen D. Sepsis-induced immunosuppression: from cellular dysfunctions to immunotherapy. Nat Rev Immunol. (2013) 13:862–74. doi: 10.1038/nri3552
102. Avdic S, McSharry BP, Slobedman B. Modulation of dendritic cell functions by viral IL-10 encoded by human cytomegalovirus. Front Microbiol. (2014) 5:337. doi: 10.3389/fmicb.2014.00337
103. Lachance P, Chen J, Featherstone R, Sligl WI. Association between cytomegalovirus reactivation and clinical outcomes in immunocompetent critically ill patients: a systematic review and meta-analysis. Open Forum Infect Dis. (2017) 4:ofx029. doi: 10.1093/ofid/ofx029
104. Luyt CE, Combes A, Deback C, Aubriot-Lorton MH, Nieszkowska A, Trouillet JL, et al. Herpes simplex virus lung infection in patients undergoing prolonged mechanical ventilation. Am J Respir Crit Care Med. (2007) 175:935–942. doi: 10.1164/rccm.200609-1322OC
105. Linssen CFM, Jacobs JA, Stelma FF, Van Mook WNKA, Terporten P, Vink C, et al. Herpes simplex virus load in bronchoalveolar lavage fluid is related to poor outcome in critically ill patients. Intensive Care Med. (2008) 34:2202–9. doi: 10.1007/s00134-008-1231-4
106. Hraiech S, Bonnardel E, Guervilly C, Fabre C, Loundou A, Forel JM, et al. Herpes simplex virus and Cytomegalovirus reactivation among severe ARDS patients under veno-venous ECMO. Ann Intensive Care. (2019) 9:142. doi: 10.1186/s13613-019-0616-6
107. Luyt CE, Forel JM, Hajage D, Jaber S, Cayot-Constantin S, Rimmelé T, et al. Acyclovir for mechanically ventilated patients with herpes simplex virus oropharyngeal reactivation: a randomized clinical trial. JAMA Intern Med. (2020) 180:263–72. doi: 10.1001/jamainternmed.2019.5713
108. Schuierer L, Gebhard M, Ruf HG, Jaschinski U, Berghaus TM, Wittmann M, et al. Impact of acyclovir use on survival of patients with ventilator-associated pneumonia and high load herpes simplex virus replication. Crit Care. (2020) 24:12. doi: 10.1186/s13054-019-2701-5
109. Papaevangelou V, Quinlivan M, Lockwood J, Papaloukas O, Sideri G, Critselis E, et al. Subclinical VZV reactivation in immunocompetent children hospitalized in the ICU associated with prolonged fever duration. Clin Microbiol Infect. (2013) 19:E245–51. doi: 10.1111/1469-0691.12131
110. Libert N, Bigaillon C, Chargari C, Bensalah M, Muller V, Merat S, et al. Epstein-Barr virus reactivation in critically ill immunocompetent patients. Biomed J. (2015) 38:70–6. doi: 10.4103/2319-4170.132905
111. Tomblyn M, Chiller T, Einsele H, Gress R, Sepkowitz K, Storek J, et al. Guidelines for preventing infectious complications among hematopoietic cell transplantation recipients: a global perspective. Biol Blood Marrow Transplant. (2009) 15:1143–238. doi: 10.1016/j.bbmt.2009.06.019
112. U.S. Food Drug Administration (FDA). Testing Donors of Human Cells, Tissues, Cellular Tissue-Based Products (HCT/P): Specific Requirements | FDA. Available online at: https://www.fda.gov/vaccines-blood-biologics/safety-availability-biologics/testing-donors-human-cells-tissues-and-cellular-and-tissue-based-products-hctp-specific-requirements (accessed January 10, 2021).
113. Persson L, Dahl H, Linde A, Engervall P, Vikerfors T, Tidefelt U. Human cytomegalovirus, human herpesvirus-6 and human herpesvirus-7 in neutropenic patients with fever of unknown origin. Clin Microbiol Infect. (2003) 9:640–4. doi: 10.1046/j.1469-0691.2003.00578.x
114. Öhrmalm L, Wong M, Aust C, Ljungman P, Norbeck O, Broliden K, et al. Viral findings in adult hematological patients with neutropenia. PLoS ONE. (2012) 7:e36543. doi: 10.1371/journal.pone.0036543
115. Wood MJ. Viral infections in neutropenia–current problems and chemotherapeutic control. J Antimicrob Chemother. (1998) 41(Suppl. D):81–93. doi: 10.1093/jac/41.suppl_4.81.103
116. Fischer SA, Lu K. Screening of donor and recipient in solid organ transplantation. Am J Transplant. (2013) 13:9–21. doi: 10.1111/ajt.12094
117. George MJ, Snydman DR, Werner BG, Griffith J, Falagas ME, Dougherty NN, et al. The independent role of cytomegalovirus as a risk factor for invasive fungal disease in orthotopic liver transplant recipients. Am J Med. (1997) 103:106–13. doi: 10.1016/S0002-9343(97)80021-6
118. Fishman JA. Infection in solid-organ transplant recipients. N Engl J Med. (2007) 357:2601–14. doi: 10.1056/NEJMra064928
119. van den Berg AP, Klompmaker IJ, Haagsma EB, Peeters PM, Meerman L, Verwer R, et al. Evidence for an increased rate of bacterial infections in liver transplant patients with cytomegalovirus infection. Clin Transplant. (1996) 10:224–31.
120. Mendez JC, Dockrell DH, Espy MJ, Smith TF, Wilson JA, Harmsen WS, et al. Human β-herpesvirus interactions in solid organ transplant recipients. J Infect Dis. (2001) 183:179–84. doi: 10.1086/317929
121. Reinke P, Lippert J, Ewert R, Fietze E, Ode-Hakim S, Volk HD, et al. Late-acute renal allograft rejection and symptomless cytomegalovirus infection. Lancet. (1994) 344:1737–8. doi: 10.1016/S0140-6736(94)92887-8
122. Streblow DN, Orloff SL, Nelson JA. Acceleration of allograft failure by cytomegalovirus. Curr Opin Immunol. (2007) 19:577–82. doi: 10.1016/j.coi.2007.07.012
123. Borchers AT, Perez R, Kaysen G, Ansari AA, Gershwin ME. Role of cytomegalovirus infection in allograft rejection: a review of possible mechanisms. Transpl Immunol. (1999) 7:75–82. doi: 10.1016/S0966-3274(99)80023-9
124. Reinke P, Prösch S, Kern F, Volk HD. Mechanisms of human cytomegalovirus (HCMV) (re)activation and its impact on organ transplant patients. Transpl Infect Dis. (1999) 1:157–64. doi: 10.1034/j.1399-3062.1999.010304.x
125. Kotton CN, Kumar D, Caliendo AM, Huprikar S, Chou S, Danziger-Isakov L, et al. The third international consensus guidelines on the management of cytomegalovirus in solid-organ transplantation. Transplantation. (2018) 102:900–31. doi: 10.1097/TP.0000000000002191
126. Razonable RR, Humar A. Cytomegalovirus in solid organ transplantation. Am J Transplant. (2013) 13:93–106. doi: 10.1111/ajt.12103
127. Muheim C, Vogel G, Seydoux C, Gillet M, Mosimann F, Von Segesser L, et al. Determinants of protracted cytomegalovirus infection in solid-organ transplant patients. Transplantation. (2002) 74:226–36. doi: 10.1097/00007890-200207270-00014
128. Legendre C, Pascual M. Improving outcomes for solid-organ transplant recipients at risk from cytomegalovirus infection: late-onset disease and indirect consequences. Clin Infect Dis. (2008) 46:732–40. doi: 10.1086/527397
129. Kumar D, Chernenko S, Moussa G, Cobos I, Manuel O, Preiksaitis J, et al. Cell-mediated immunity to predict cytomegalovirus disease in high-risk solid organ transplant recipients. Am J Transplant. (2009) 9:1214–22. doi: 10.1111/j.1600-6143.2009.02618.x
130. Vidrih JA, Walensky RP, Sax PE, Freedberg KA. Positive Epstein-Barr virus heterophile antibody tests in patients with primary human immunodeficiency virus infection. Am J Med. (2001) 111:192–4. doi: 10.1016/S0002-9343(01)00804-X
131. Goldberg DE, Smithen LM, Angelilli A, Freeman WR. HIV-associated retinopathy in the HAART era. Retina. (2005) 25:633–49. doi: 10.1097/00006982-200507000-00015
132. Morgello S, Cho ES, Nielsen S, Devinsky O, Petito CK. Cytomegalovirus encephalitis in patients with acquired immunodeficiency syndrome: an autopsy study of 30 cases and a review of the literature. Hum Pathol. (1987) 18:289–97. doi: 10.1016/S0046-8177(87)80012-6
133. Corey L, Wald A, Celum CL, Quinn TC. The effects of herpes simplex virus-2 on HIV-1 acquisition and transmission: a review of two overlapping epidemics. J Acquir Immune Defic Syndr. (2004) 35:435–45. doi: 10.1097/00126334-200404150-00001
134. DeSimone JA, Pomerantz RJ, Babinchak TJ. Inflammatory reactions in HIV-1-infected persons after initiation of highly active antiretroviral therapy. Ann Intern Med. (2000) 133:447–54. doi: 10.7326/0003-4819-133-6-200009190-00013
135. Lingappa JR, Celum C. Clinical and therapeutic issues for herpes simplex virus-2 and HIV co-infection. Drugs. (2007) 67:155–74. doi: 10.2165/00003495-200767020-00001
137. Pinto M, Dobson S. BK and JC virus: a review. J Infect. (2014) 68(Suppl. 1):S2–8. doi: 10.1016/j.jinf.2013.09.009
138. Gupta S, Govindarajan S, Fong TL, Redeker AG. Spontaneous reactivation in chronic hepatitis B: patterns and natural history. J Clin Gastroenterol. (1990) 12:562–8. doi: 10.1097/00004836-199010000-00015
139. Terrault NA, Lok ASF, McMahon BJ, Chang KM, Hwang JP, Jonas MM, et al. Update on prevention, diagnosis, and treatment of chronic hepatitis B: AASLD 2018 hepatitis B guidance. Hepatology. (2018) 67:1560–99. doi: 10.1002/hep.29800
140. Papatheodoridis G, Buti M, Cornberg M, Janssen H, Mutimer D, Pol S, et al. EASL clinical practice guidelines: management of chronic hepatitis B virus infection. J Hepatol. (2012) 57:167–85. doi: 10.1016/j.jhep.2012.02.010
141. Reddy KR, Beavers KL, Hammond SP, Lim JK, Falck-Ytter YT. American Gastroenterological Association Institute guideline on the prevention and treatment of hepatitis B virus reactivation during immunosuppressive drug therapy. Gastroenterology. (2015) 148:215–9. doi: 10.1053/j.gastro.2014.10.039
142. Kojaoghlanian T, Flomenberg P, Horwitz MS. The impact of adenovirus infection on the immunocompromised host. Rev Med Virol. (2003) 13:155–71. doi: 10.1002/rmv.386
143. Radke JR, Cook JL. Human adenovirus infections: update and consideration of mechanisms of viral persistence. Curr Opin Infect Dis. (2018) 31:251–6. doi: 10.1097/QCO.0000000000000451
144. Zheng Y, Stamminger T, Hearing P. E2F/Rb family proteins mediate interferon induced repression of adenovirus immediate early transcription to promote persistent viral infection. PLoS Pathog. (2016) 12:e1005415. doi: 10.1371/journal.ppat.1005415
145. Lion T. Adenovirus persistence, reactivation, and clinical management. FEBS Lett. (2019) 593:3571–82. doi: 10.1002/1873-3468.13576
146. Ison MG. Emerging infections: adenovirus infections in transplant recipients. Clin Infect Dis. (2006) 43:331–9. doi: 10.1086/505498
147. Florescu DF, Hoffman JA. Adenovirus in solid organ transplantation. Am J Transplant. (2013) 13:206–11. doi: 10.1111/ajt.12112
148. Nelson PP, Rath BA, Fragkou PC, Antalis E, Tsiodras S, Skevaki C. Current and future point-of-care tests for emerging and new respiratory viruses and future perspectives. Front Cell Infect Microbiol. (2020) 10:181. doi: 10.3389/fcimb.2020.00181
149. Zhang N, Wang L, Deng X, Liang R, Su M, He C, et al. Recent advances in the detection of respiratory virus infection in humans. J Med Virol. (2020) 92:408–17. doi: 10.1002/jmv.25674
150. Brendish NJ, Malachira AK, Clark TW. Molecular point-of-care testing for respiratory viruses versus routine clinical care in adults with acute respiratory illness presenting to secondary care: a pragmatic randomised controlled trial protocol (ResPOC). BMC Infect Dis. (2017) 17:128. doi: 10.1186/s12879-017-2219-x
151. Vos LM, Bruning AHL, Reitsma JB, Schuurman R, Riezebos-Brilman A, Hoepelman AIM, et al. Rapid molecular tests for influenza, respiratory syncytial virus, and other respiratory viruses: a systematic review of diagnostic accuracy and clinical impact studies. Clin Infect Dis. (2019) 69:1243–53. doi: 10.1093/cid/ciz056
152. Chan PKS, Lee N, Joynt GM, Choi KW, Cheung JLK, Yeung ACM, et al. Clinical and virological course of infection with haemagglutinin D222G mutant strain of 2009 pandemic influenza A (H1N1) virus. J Clin Virol. (2011) 50:320–4. doi: 10.1016/j.jcv.2011.01.013
153. Lee N, Hurt AC. Neuraminidase inhibitor resistance in influenza: a clinical perspective. Curr Opin Infect Dis. (2018) 31:520–6. doi: 10.1097/QCO.0000000000000498
154. Simon B, Pichon M, Valette M, Burfin G, Richard M, Lina B, et al. Whole genome sequencing of A(H3N2) influenza viruses reveals variants associated with severity during the 2016-2017 season. Viruses. (2019) 11:108. doi: 10.3390/v11020108
155. Li D, Wang D, Dong J, Wang N, Huang H, Xu H, et al. False-negative results of real-time reverse-transcriptase polymerase chain reaction for severe acute respiratory syndrome coronavirus 2: role of deep-learning-based ct diagnosis and insights from two cases. Korean J Radiol. (2020) 21:505–8. doi: 10.3348/kjr.2020.0146
156. Lippi G, Simundic AM, Plebani M. Potential preanalytical and analytical vulnerabilities in the laboratory diagnosis of coronavirus disease 2019 (COVID-19). Clin Chem Lab Med. (2020) 58:1070–6. doi: 10.1515/cclm-2020-0285
157. Póvoa P, Coelho L. Clinical significance of viral detection in critically ill patients more questions than answers. Am J Respir Crit Care Med. (2019) 199:411–3. doi: 10.1164/rccm.201809-1778ED
158. Centers for Disease Control and Prevention (CDC). Interim Guidance for Antigen Testing for SARS-CoV-2 | CDC. (2020). Available online at: https://www.cdc.gov/coronavirus/2019-ncov/lab/resources/antigen-tests-guidelines.html (accessed January 10, 2021).
159. Steiner I, Schmutzhard E, Sellner J, Chaudhuri A, Kennedy PGE. EFNS-ENS guidelines for the use of PCR technology for the diagnosis of infections of the nervous system. Eur J Neurol. (2012) 19:1278–91. doi: 10.1111/j.1468-1331.2012.03808.x
160. Halperin JJ. Diagnosis and management of acute encephalitis. In: Wijdicks EFM, Kramer AH, editors. Handbook of Clinical Neurology. Amsterdam: Elsevier B.V. (2017). p. 337–47. doi: 10.1016/B978-0-444-63600-3.00018-0
161. Koo HJ, Lim S, Choe J, Choi SH, Sung H, Do KH. Radiographic and CT features of viral pneumonia. Radiographics. (2018) 38:719–39. doi: 10.1148/rg.2018170048
162. Islam N, Salameh J-P, Leeflang MM, Hooft L, McGrath TA, van der Pol CB, et al. Thoracic imaging tests for the diagnosis of COVID-19. Cochrane Database Syst Rev. (2020) 11:CD013639. doi: 10.1002/14651858.cd013639.pub3
163. Venkatesan A, Michael BD, Probasco JC, Geocadin RG, Solomon T. Acute encephalitis in immunocompetent adults. Lancet. (2019) 393:702–16. doi: 10.1016/S0140-6736(18)32526-1
164. Lynch JP, Kajon AE. Adenovirus: epidemiology, global spread of novel serotypes, and advances in treatment and prevention. Semin Respir Crit Care Med. (2016) 37:586–602. doi: 10.1055/s-0036-1584923
165. Hakim FA, Tleyjeh IM. Severe adenovirus pneumonia in immunocompetent adults: a case report and review of the literature. Eur J Clin Microbiol Infect Dis. (2008) 27:153–8. doi: 10.1007/s10096-007-0416-z
166. Kim YJ, Boeckh M, Englund JA. Community respiratory virus infections in immunocompromised patients: hematopoietic stem cell and solid organ transplant recipients, and individuals with human immunodeficiency virus infection. Semin Respir Crit Care Med. (2007) 28:222–42. doi: 10.1055/s-2007-976494
167. Tylka JC, McCrory MC, Gertz SJ, Custer JW, Spaeder MC. Immunocompromised children with severe adenoviral respiratory infection. Crit Care Res Pract. (2016) 2016:9458230. doi: 10.1155/2016/9458230
168. Lion T. Adenovirus infections in immunocompetent and immunocompromised patients. Clin Microbiol Rev. (2014) 27:441–462. doi: 10.1128/CMR.00116-13
169. Barker JH, Luby JP, Dalley AS, Bartek WM, Burns DK, Erdman DD. Fatal type 3 adenoviral pneumonia in immunocompetent adult identical twins. Clin Infect Dis. (2003) 37:e142–6. doi: 10.1086/379127
170. Pfortmueller CA, Barbani MT, Schefold JC, Hage E, Heim A, Zimmerli S. Severe acute respiratory distress syndrome (ARDS) induced by human adenovirus B21: report on 2 cases and literature review. J Crit Care. (2019) 51:99–104. doi: 10.1016/j.jcrc.2019.02.019
171. Savón C, Acosta B, Valdés O, Goyenechea A, Gonzalez G, Piñón A, et al. A myocarditis outbreak with fatal cases associated with adenovirus subgenera C among children from Havana City in 2005. J Clin Virol. (2008) 43:152–7. doi: 10.1016/j.jcv.2008.05.012
172. Pawełczyk M, Kowalski ML. The role of human parainfluenza virus infections in the immunopathology of the respiratory tract. Curr Allergy Asthma Rep. (2017) 17:16. doi: 10.1007/s11882-017-0685-2
173. Djamin RS, Uzun S, Snelders E, Kluytmans JJW, Hoogsteden HC, Aerts JGJV, et al. Occurrence of virus-induced COPD exacerbations during four seasons. Infect Dis. (2015) 47:96–100. doi: 10.3109/00365548.2014.968866
175. Song Z, Xu Y, Bao L, Zhang L, Yu P, Qu Y, et al. From SARS to MERS, thrusting coronaviruses into the spotlight. Viruses. (2019) 11:59. doi: 10.3390/v11010059
176. Assiri A, Al-Tawfiq JA, Al-Rabeeah AA, Al-Rabiah FA, Al-Hajjar S, Al-Barrak A, et al. Epidemiological, demographic, and clinical characteristics of 47 cases of Middle East respiratory syndrome coronavirus disease from Saudi Arabia: A descriptive study. Lancet Infect Dis. (2013) 13:752–61. doi: 10.1016/S1473-3099(13)70204-4
177. Eisenhut M. Extrapulmonary manifestations of severe respiratory syncytial virus infection–A systematic review. Crit Care. (2006) 10:R107. doi: 10.1186/cc4984
178. Nam HH, Ison MG. Respiratory syncytial virus infection in adults. BMJ. (2019) 366:l5021. doi: 10.1136/bmj.l5021
179. Falsey AR, Walsh EE. Respiratory syncytial virus infection in adults. Clin Microbiol Rev. (2000) 13:371–384. doi: 10.1128/CMR.13.3.371-384.2000
180. Smith DK, Seales S, Budzik C. Respiratory syncytial virus bronchiolitis in children. Am Fam Physician. (2017) 95:94–9.
181. Centers for Disease Control and Prevention (CDC). Preliminary clinical description of severe acute respiratory syndrome. MMWR Morb Mortal Wkly Rep. (2003) 52:255–256.
182. Huang C, Wang Y, Li X, Ren L, Zhao J, Hu Y, et al. Clinical features of patients infected with 2019 novel coronavirus in Wuhan, China. Lancet. (2020) 395:497–506. doi: 10.1016/S0140-6736(20)30183-5
183. Chan JFW, Yuan S, Kok KH, To KKW, Chu H, Yang J, et al. A familial cluster of pneumonia associated with the 2019 novel coronavirus indicating person-to-person transmission: a study of a family cluster. Lancet. (2020) 395:514–23. doi: 10.1016/S0140-6736(20)30154-9
184. Guan W, Ni Z, Hu Y, Liang W, Ou C, He J, et al. Clinical characteristics of coronavirus disease 2019 in China. N Engl J Med. (2020) 382:1708–20. doi: 10.1056/NEJMoa2002032
185. Tsivgoulis G, Palaiodimou L, Katsanos AH, Caso V, Köhrmann M, Molina C, et al. Neurological manifestations and implications of COVID-19 pandemic. Ther Adv Neurol Disord. (2020) 13:175628642093203. doi: 10.1177/1756286420932036
186. Tsivgoulis G, Fragkou PC, Lachanis S, Palaiodimou L, Lambadiari V, Papathanasiou M, et al. Olfactory bulb and mucosa abnormalities in persistent COVID-19-induced anosmia: a magnetic resonance imaging study. Eur J Neurol. (2020) 28:e6–8. doi: 10.1111/ene.14537
187. Bassetti M, Kollef MH, Timsit JF. Bacterial and fungal superinfections in critically ill patients with COVID-19. Intensive Care Med. (2020) 46:2071–4. doi: 10.1007/s00134-020-06219-8
188. World Health Organization (WHO). WHO Surveillance Case Definitions for ILI and SARI. (2018). Available online at: https://www.who.int/influenza/surveillance_monitoring/ili_sari_surveillance_case_definition/en/ (accessed January 11, 2021).
189. Mailles A, Stahl J. Infectious encephalitis in france in 2007: a National Prospective Study. Clin Infect Dis. (2009) 49:1838–47. doi: 10.1086/648419
190. Sonneville R, Klein I, de Broucker T, Wolff M. Post-infectious encephalitis in adults: diagnosis and management. J Infect. (2009) 58:321–8. doi: 10.1016/j.jinf.2009.02.011
191. Johnson RT. The pathogenesis of acute viral encephalitis and postinfectious encephalomyelitis. J Infect Dis. (1987) 155:359–64. doi: 10.1093/infdis/155.3.359
192. Basler CF. Molecular pathogenesis of viral hemorrhagic fever. Semin Immunopathol. (2017) 39:551–61. doi: 10.1007/s00281-017-0637-x
193. Paessler S, Walker DH. Pathogenesis of the viral hemorrhagic fevers. Annu Rev Pathol Mech Dis. (2013) 8:411–40. doi: 10.1146/annurev-pathol-020712-164041
194. Aleksandrowicz P, Wolf K, Falzarano D, Feldmann H, Seebach J, Schnittler H. Viral haemorrhagic fever and vascular alterations. Hamostaseologie. (2008) 28:77–84.
195. Messaoudi I, Basler CF. Immunological features underlying viral hemorrhagic fevers. Curr Opin Immunol. (2015) 36:38–46. doi: 10.1016/j.coi.2015.06.003
196. Hidalgo J, Richards GA, Jiménez JIS, Baker T, Amin P. Viral hemorrhagic fever in the tropics: report from the task force on tropical diseases by the World Federation of Societies of Intensive and Critical Care Medicine. J Crit Care. (2017) 42:366–72. doi: 10.1016/j.jcrc.2017.11.006
197. Schnittler H-J, Feldmann H. Viral hemorrhagic fever–a vascular disease? Thromb Haemost. (2003) 89:967–72.
198. European Association for the Study of the Liver, Electronic address: ZWFzbG9mZmljZSYjeDAwMDQwO2Vhc2xvZmZpY2UuZXU=, Clinical practice guidelines panel, Wendon J, Panel members, Cordoba J, et al. EASL Clinical Practical Guidelines on the management of acute (fulminant) liver failure. J Hepatol. (2017) 66:1047–81. doi: 10.1016/j.jhep.2016.12.003
199. Smith DB, Simmonds P. Hepatitis E virus and fulminant hepatitis-a virus or host-specific pathology? Liver Int. (2015) 35:1334–40. doi: 10.1111/liv.12629
200. Ajmera V, Xia G, Vaughan G, Forbi JC, Ganova-Raeva LM, Khudyakov Y, et al. What factors determine the severity of hepatitis A-related acute liver failure? J Viral Hepat. (2011) 18:e167–74. doi: 10.1111/j.1365-2893.2010.01410.x
201. Centers for Disease Control and Prevention (CDC). Principles of Epidemiology | Lesson 1 - Section 10. (2012). Available online at: https://www.cdc.gov/csels/dsepd/ss1978/lesson1/section10.html (accessed January 10, 2021).
202. Centers for Disease Control Prevention (CDC). Transmission-Based Precautions | Basics | Infection Control | CDC. Available online at: https://www.cdc.gov/infectioncontrol/basics/transmission-based-precautions.html (accessed January 10, 2021).
203. World Health Organization (WHO). How to guide-putting on PPE for Contact/Droplet Precautions Perform Hand Hygiene. Available online at: https://www.who.int/csr/resources/publications/ppe_en.pdf?ua=1 (accessed January 10, 2021).
204. Centers for Disease Control Preventoion (CDC). Infection Prevention Control Recommendations for Hospitalized Patients Under Investigation (PUIs) for Ebola Virus Disease (EVD) in U.S. Hospitals | Ebola Virus Disease | Clinicians | Ebola (Ebola Virus Disease) | CDC. (2018). Available online at: https://www.cdc.gov/vhf/ebola/clinicians/evd/infection-control.html (accessed January 10, 2021).
205. Centers for Disease Control and Prevention (CDC). Guidance on Personal Protective Equipment (PPE) | Personal Protective Equipment (PPE) | Public Health Planners | Ebola (Ebola Virus Disease) | CDC. (2018). Available online at: https://www.cdc.gov/vhf/ebola/healthcare-us/ppe/guidance.html (accessed January 11, 2021).
206. Simoons-Smit AM, Kraan EM, Beishuizen A, Strack van Schijndel RJ, Vandenbroucke-Grauls CM. Herpes simplex virus type 1 and respiratory disease in critically-ill patients: real pathogen or innocent bystander? Clin Microbiol Infect. (2006) 12:1050–59. doi: 10.1111/j.1469-0691.2006.01475.x
207. Tuxen D V., Wilson JW, Cade JF. Prevention of lower respiratory herpes simplex virus infection with acyclovir in patients with the adult respiratory distress syndrome. Am Rev Respir Dis. (1987) 136:402–5. doi: 10.1164/ajrccm/136.2.402
208. Forel JM, Martin-Loeches I, Luyt CE. Treating HSV and CMV reactivations in critically ill patients who are not immunocompromised: pro. Intensive Care Med. (2014) 40:1945–9. doi: 10.1007/s00134-014-3445-y
209. Schildermans J, De Vlieger G. Cytomegalovirus: a troll in the ICU? Overview of the literature and perspectives for the future. Front Med. (2020) 7:188. doi: 10.3389/fmed.2020.00188
210. Cowley NJ, Owen A, Shiels SC, Millar J, Woolley R, Ives N, et al. Safety and efficacy of antiviral therapy for prevention of cytomegalovirus reactivation in immunocompetent critically ill patients: a randomized clinical trial. JAMA Intern Med. (2017) 177:774–83. doi: 10.1001/jamainternmed.2017.0895
211. Limaye AP, Stapleton RD, Peng L, Gunn SR, Kimball LE, Hyzy R, et al. Effect of ganciclovir on IL-6 levels among cytomegalovirus-seropositive adults with critical illness: a randomized clinical trial. JAMA - J Am Med Assoc. (2017) 318:731–40. doi: 10.1001/jama.2017.10569
212. Chanques G, Jaber S. Treating HSV and CMV reactivations in critically ill patients who are not immunocompromised: con. Intensive Care Med. (2014) 40:1950–3. doi: 10.1007/s00134-014-3521-3
213. Torres A, Loeches IM, Sligl W, Lee N. Severe flu management: a point of view. Intensive Care Med. (2020) 46:153–62. doi: 10.1007/s00134-019-05868-8
214. Ng KE. Xofluza (Baloxavir marboxil) for the treatment of acute uncomplicated influenza. P T. (2019) 44:9–11.
215. World Health Organization (WHO). Influenza (Seasonal). (2018). Available online at: https://www.who.int/en/news-room/fact-sheets/detail/influenza-(seasonal) (accessed June 13, 2020).
216. Sedyaningsih ER, Malik MS, Setiawaty V, Trihono T, Burhan E, Aditama TY, et al. Effect of double dose oseltamivir on clinical and virological outcomes in children and adults admitted to hospital with severe influenza: double blind randomised controlled trial. BMJ. (2013) 346:f3039. doi: 10.1136/bmj.f3039
217. Welch SC, Lam SW, Neuner EA, Bauer SR, Bass SN. High-dose versus standard dose oseltamivir for treatment of severe influenza in adult intensive care unit patients. Intensive Care Med. (2015) 41:1365–6. doi: 10.1007/s00134-015-3816-z
218. Muthuri SG, Venkatesan S, Myles PR, Leonardi-Bee J, Al Khuwaitir TSA, Al Mamun A, et al. Effectiveness of neuraminidase inhibitors in reducing mortality in patients admitted to hospital with influenza A H1N1pdm09 virus infection: a meta-analysis of individual participant data. Lancet Respir Med. (2014) 2:395–404. doi: 10.1016/S2213-2600(14)70041-4
219. Sheahan TP, Sims AC, Leist SR, Schäfer A, Won J, Brown AJ, et al. Comparative therapeutic efficacy of remdesivir and combination lopinavir, ritonavir, and interferon beta against MERS-CoV. Nat Commun. (2020) 11:222. doi: 10.1038/s41467-019-13940-6
220. National Institutes of Health (NIH). Therapeutic Management | COVID-19 Treatment Guidelines. (2020). Available online at: https://www.covid19treatmentguidelines.nih.gov/therapeutic-management/ (accessed January 11, 2021).
221. Goldman JD, Lye DCB, Hui DS, Marks KM, Bruno R, Montejano R, et al. Remdesivir for 5 or 10 days in patients with severe covid-19. N Engl J Med. (2020) 383:1827–37. doi: 10.1056/nejmoa2015301
222. Spinner CD, Gottlieb RL, Criner GJ, Arribas López JR, Cattelan AM, Soriano Viladomiu A, et al. Effect of remdesivir vs standard care on clinical status at 11 days in patients with moderate COVID-19: a randomized clinical trial. J Am Med Assoc. (2020) 324:1048–57. doi: 10.1001/jama.2020.16349
223. Wang Y, Zhang D, Du G, Du R, Zhao J, Jin Y, et al. Remdesivir in adults with severe COVID-19: a randomised, double-blind, placebo-controlled, multicentre trial. Lancet. (2020) 395:1569–78. doi: 10.1016/S0140-6736(20)31022-9
224. Beigel JH, Tomashek KM, Dodd LE, Mehta AK, Zingman BS, Kalil AC, et al. Remdesivir for the treatment of Covid-19 — final report. N Engl J Med. (2020) 383:1813–26. doi: 10.1056/nejmoa2007764
225. Wold Health Organization (WHO). WHO Recommends Against the Use of Remdesivir in COVID-19 Patients. (2020). Available online at: https://www.who.int/news-room/feature-stories/detail/who-recommends-against-the-use-of-remdesivir-in-covid-19-patients (accessed January 11, 2021).
226. Fragkou PC, Belhadi D, Peiffer-Smadja N, Moschopoulos CD, Lescure F-X, Janocha H, et al. Review of trials currently testing treatment and prevention of COVID-19. Clin Microbiol Infect. (2020) 26:988–98. doi: 10.1016/j.cmi.2020.05.019
227. Seo S, Xie H, Campbell AP, Kuypers JM, Leisenring WM, Englund JA, et al. Parainfluenza virus lower respiratory tract disease after hematopoietic cell transplant: viral detection in the lung predicts outcome. Clin Infect Dis. (2014) 58:1357–68. doi: 10.1093/cid/ciu134
228. Foolad F, Aitken SL, Shigle TL, Prayag A, Ghantoji S, Ariza-Heredia E, et al. Oral versus aerosolized ribavirin for the treatment of respiratory syncytial virus infections in hematopoietic cell transplant recipients. Clin Infect Dis. (2019) 68:1641–9. doi: 10.1093/cid/ciy760
229. Wyde PR, Chetty SN, Jewell AM, Boivin G, Piedra PA. Comparison of the inhibition of human metapneumovirus and respiratory syncytial virus by ribavirin and immune serum globulin in vitro. Antiviral Res. (2003) 60:51–9. doi: 10.1016/S0166-3542(03)00153-0
230. Kitanovski L, Kopriva S, Pokorn M, Dolničar MB, Rajić V, Stefanović M, et al. Treatment of severe human metapneumovirus (hmpv) pneumonia in an immunocompromised child with oral ribavirin and ivig. J Pediatr Hematol Oncol. (2013) 35:e311–3. doi: 10.1097/MPH.0b013e3182915d2d
231. Hamelin ME, Gagnon C, Prince GA, Kiener P, Suzich JA, Ulbrandt N, et al. Prophylactic and therapeutic benefits of a monoclonal antibody against the fusion protein of human metapneumovirus in a mouse model. Antiviral Res. (2010) 88:31–7. doi: 10.1016/j.antiviral.2010.07.001
232. Ulbrandt ND, Ji H, Patel NK, Riggs JM, Brewah YA, Ready S, et al. Isolation and characterization of monoclonal antibodies which neutralize human metapneumovirus in vitro and in vivo. J Virol. (2006) 80:7799–806. doi: 10.1128/jvi.00318-06
233. Corti D, Bianchi S, Vanzetta F, Minola A, Perez L, Agatic G, et al. Cross-neutralization of four paramyxoviruses by a human monoclonal antibody. Nature. (2013) 501:439–43. doi: 10.1038/nature12442
234. Deffrasnes C, Hamelin MÈ, Prince GA, Boivin G. Identification and evaluation of a highly effective fusion inhibitor for human metapneumovirus. Antimicrob Agents Chemother. (2008) 52:279–87. doi: 10.1128/AAC.00793-07
235. Van Genechten T, van Heerden J, Bauters T, Dhooge C. Successful treatment of adenovirus infection with brincidofovir in an immunocompromised patient after hematological stem cell transplantation. Case Rep Infect Dis. (2020) 2020:5981289. doi: 10.1155/2020/5981289
236. Grimley MS, Chemaly RF, Englund JA, Kurtzberg J, Chittick G, Brundage TM, et al. Brincidofovir for asymptomatic adenovirus viremia in pediatric and adult allogeneic hematopoietic cell transplant recipients: a randomized placebo-controlled phase II trial. Biol Blood Marrow Transplant. (2017) 23:512–21. doi: 10.1016/j.bbmt.2016.12.621
237. Sudhindra P, Knoll B, Nog R, Singh N, Dhand A. Brincidofovir (CMX001) for the treatment of severe adenoviral pneumonia in kidney transplant recipient. Cureus. (2019) 11:e5296. doi: 10.7759/cureus.5296
238. Grahn A, Studahl M. Varicella-zoster virus infections of the central nervous system - prognosis, diagnostics and treatment. J Infect. (2015) 71:281–93. doi: 10.1016/j.jinf.2015.06.004
239. Stoeter DJ, Michael BD, Solomon T, Poole L. Managing acute central nervous system infections in the UK adult intensive care unit in the wake of UK encephalitis guidelines. J Intensive Care Soc. (2015) 16:330–8. doi: 10.1177/1751143715587927
240. Steiner I, Budka H, Chaudhuri A, Koskiniemi M, Sainio K, Salonen O, et al. Viral meningoencephalitis: a review of diagnostic methods and guidelines for management. Eur J Neurol. (2010) 17:999–e57. doi: 10.1111/j.1468-1331.2010.02970.x
241. Newcomb G, Mariuz P, Lachant D. CMV encephalitis/radiculitis: the difficulty in diagnosing in an intubated patient. Case Rep Crit Care. (2019) 2019:8067648. doi: 10.1155/2019/8067648
242. Dalton HR, Kamar N, Baylis SA, Moradpour D, Wedemeyer H, Negro F. EASL clinical practice guidelines on hepatitis E virus infection. J Hepatol. (2018) 68:1256–71. doi: 10.1016/j.jhep.2018.03.005
243. Lampertico P, Agarwal K, Berg T, Buti M, Janssen HLA, Papatheodoridis G, et al. EASL 2017 clinical practice guidelines on the management of hepatitis B virus infection. J Hepatol. (2017) 67:370–98. doi: 10.1016/j.jhep.2017.03.021
244. Zhou Y, Fu X, Liu X, Huang C, Tian G, Ding C, et al. Use of corticosteroids in influenza-associated acute respiratory distress syndrome and severe pneumonia: a systemic review and meta-analysis. Sci Rep. (2020) 10:3044. doi: 10.1038/s41598-020-59732-7
245. RECOVERY Collaborative Group, Horby P, Lim WS, Emberson JR, Mafham M, Bell JL, et al. Dexamethasone in hospitalized patients with Covid-19 - preliminary report. N Engl J Med. (2020). doi: 10.1056/nejmoa2021436. [Epub ahead of print].
246. Tomazini BM, Maia IS, Cavalcanti AB, Berwanger O, Rosa RG, Veiga VC, et al. Effect of dexamethasone on days alive and ventilator-free in patients with moderate or severe acute respiratory distress syndrome and COVID-19: the CoDEX randomized clinical trial. J Am Med Assoc. (2020) 324:1307–16. doi: 10.1001/jama.2020.17021
247. Angus DC, Derde L, Al-Beidh F, Annane D, Arabi Y, Beane A, et al. Effect of hydrocortisone on mortality and organ support in patients with severe COVID-19: the REMAP-CAP COVID-19 corticosteroid domain randomized clinical trial. J Am Med Assoc. (2020) 324:1317–29. doi: 10.1001/jama.2020.17022
248. Jeronimo CMP, Farias MEL, Val FFA, Sampaio VS, Alexandre MAA, Melo GC, et al. Methylprednisolone as adjunctive therapy for patients hospitalized with coronavirus disease 2019 (COVID-19; metcovid): a randomized, double-blind, phase IIb, placebo-controlled trial. Clin Infect Dis. (2020) ciaa1177. doi: 10.1093/cid/ciaa1177. [Epub ahead of print].
249. World Health Organization (WHO). WHO Updates Clinical Care Guidance with Corticosteroid Recommendations. (2020). Available online at: https://www.who.int/news-room/feature-stories/detail/who-updates-clinical-care-guidance-with-corticosteroid-recommendations (accessed January 11, 2021).
250. da Costa BK, Sato DK. Viral encephalitis: a practical review on diagnostic approach and treatment. J Pediatr. (2020) 96:12–9. doi: 10.1016/j.jped.2019.07.006
251. Hung IFN, To KKW, Lee CK, Lee KL, Yan WW, Chan K, et al. Hyperimmune IV immunoglobulin treatment: a multicenter double-blind randomized controlled trial for patients with severe 2009 influenza A(H1N1) infection. Chest. (2013) 144:464–73. doi: 10.1378/chest.12-2907
252. National Institutes of Health (NIH). Blood-Derived Products | COVID-19 Treatment Guidelines. (2020). Available online at: https://www.covid19treatmentguidelines.nih.gov/immune-based-therapy/blood-derived-products/ (accessed January 11, 2021).
253. National Institutes for Health (NIH). Statement on Baricitinib EUA | COVID-19 Treatment Guidelines. (2020). Available online at: https://www.covid19treatmentguidelines.nih.gov/statement-on-baricitinib-eua/ (accessed January 11, 2021).
254. Tang PK. Palivizumab prophylaxis in preterm infants. Lancet Respir Med. (2017) 5:171. doi: 10.1016/S2213-2600(17)30050-4
255. Mochizuki H, Kusuda S, Okada K, Yoshihara S, Furuya H, Simões EAF, et al. Palivizumab prophylaxis in preterm infants and subsequent recurrent wheezing: six-year follow-up study. Am J Respir Crit Care Med. (2017) 196:29–38. doi: 10.1164/rccm.201609-1812OC
256. Salazar G, Zhang N, Fu TM, An Z. Antibody therapies for the prevention and treatment of viral infections. npj Vaccines. (2017) 2:1–12. doi: 10.1038/s41541-017-0019-3
257. Centers for Disease Control and Prevention (CDC). Seasonal Flu Shot | CDC. (2020). Available online at: https://www.cdc.gov/flu/prevent/flushot.htm (accessed January 11, 2021).
258. World Health Organization (WHO). Middle East respiratory syndrome coronavirus (MERS-CoV). (2019). Available online at: https://www.who.int/news-room/q-a-detail/middle-east-respiratory-syndrome-coronavirus-(mers-cov) (accessed January 11, 2021).
259. Milken Institute. COVID-19 Vaccine Tracker. (2021). Available online at: https://www.covid-19vaccinetracker.org/ (accessed January 11, 2021).
260. van Riel D, de Wit E. Next-generation vaccine platforms for COVID-19. Nat Mater. (2020) 19:810–2. doi: 10.1038/s41563-020-0746-0
261. Centers for Diseae Control and Prevention (CDC). Vaccine Information Statement | Adenovirus | VIS | CDC. (2020). Available online at: https://www.cdc.gov/vaccines/hcp/vis/vis-statements/adenovirus.html (accessed June 22, 2020).
262. Dooling KL, Guo A, Patel M, Lee GM, Moore K, Belongia EA, et al. Recommendations of the advisory committee on immunization practices for use of herpes zoster vaccines. Morb Mortal Wkly Rep. (2018) 67:103–8. doi: 10.15585/mmwr.mm6703a5
263. Yin WW, Wang CL, Chen QL, Dong GM, Li YH, Zhu WY, et al. [Expert consensus on rabies exposure prophylaxis]. Zhonghua Yu Fang Yi Xue Za Zhi. (2019) 53:668–79. doi: 10.3760/cma.j.issn.0253-9624.2019.07.004
Keywords: viral infections, reactivation, respiratory tract infection, intensive care unit, critically ill, critical care, neurologic syndrome, shock
Citation: Fragkou PC, Moschopoulos CD, Karofylakis E, Kelesidis T and Tsiodras S (2021) Update in Viral Infections in the Intensive Care Unit. Front. Med. 8:575580. doi: 10.3389/fmed.2021.575580
Received: 23 June 2020; Accepted: 02 February 2021;
Published: 23 February 2021.
Edited by:
Alberto Enrico Maraolo, University of Naples Federico II, ItalyReviewed by:
Thiago DeSouza-Vieira, National Institutes of Health (NIH), United StatesNaoshi Takeyama, Aichi Medical University, Japan
Copyright © 2021 Fragkou, Moschopoulos, Karofylakis, Kelesidis and Tsiodras. This is an open-access article distributed under the terms of the Creative Commons Attribution License (CC BY). The use, distribution or reproduction in other forums is permitted, provided the original author(s) and the copyright owner(s) are credited and that the original publication in this journal is cited, in accordance with accepted academic practice. No use, distribution or reproduction is permitted which does not comply with these terms.
*Correspondence: Paraskevi C. Fragkou, ZXZpdGEuZnJhZ291JiN4MDAwNDA7Z21haWwuY29t
†These authors have contributed equally to this work