- 1School of Pharmacy and Pharmaceutical Sciences, Cardiff University, Cardiff, United Kingdom
- 2School of Medicine, Institute of Infection and Immunity, Cardiff University, Cardiff, United Kingdom
- 3Wound Healing Research Unit, Welsh Wound Innovation Centre, School of Medicine, Cardiff University, Cardiff, United Kingdom
The coronavirus disease (COVID-19) outbreak has presented enormous challenges for healthcare, societal, and economic systems worldwide. There is an urgent global need for a universal vaccine to cover all SARS-CoV-2 mutant strains to stop the current COVID-19 pandemic and the threat of an inevitable second wave of coronavirus. Carbon dioxide is safe and superior antimicrobial, which suggests it should be effective against coronaviruses and mutants thereof. Depending on the therapeutic regime, CO2 could also ameliorate other COVID-19 symptoms as it has also been reported to have antioxidant, anti-inflammation, anti-cytokine effects, and to stimulate the human immune system. Moreover, CO2 has beneficial effects on respiratory physiology, cardiovascular health, and human nervous systems. This article reviews the rationale of early treatment by inhaling safe doses of warmed humidified CO2 gas, either alone or as a carrier gas to deliver other inhaled drugs may help save lives by suppressing SARS-CoV-2 infections and excessive inflammatory responses. We suggest testing this somewhat counter-intuitive, but low tech and safe intervention for its suitability as a preventive measure and treatment against COVID-19. Overall, development and evaluation of this therapy now may provide a safe and economical tool for use not only during the current pandemic but also for any future outbreaks of respiratory diseases and related conditions.
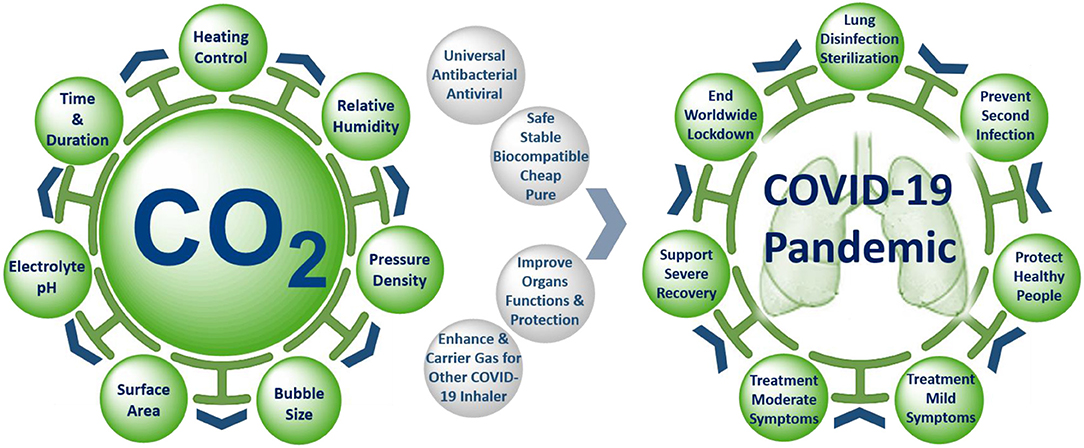
Graphical Abstract. Precise control of the unique properties and intervention parameters of warmed humidified CO2 gas make it a promising anti-COVID-19 therapy for mitigation and suppression of SARS-CoV-2 infection.
Background
The coronavirus disease (COVID-19) outbreak has presented enormous challenges for healthcare systems worldwide and caused terrible societal and economic impacts. There is also an urgent need to address health inequality in treating the current COVID-19 pandemic. Even now, scientists are racing to unravel sometimes conflicting information to understand the source, diagnose, and find effective treatments for SARS-CoV-2, and to conduct clinical trials of antiviral drugs and vaccines. Other COVID-19 mysteries include the appearance of new symptoms, the relation of silent hypoxia and sudden deaths, spikes insignificant vessel blockages, and increased risks of clotting (1). The virus is now known to be able to target a wide variety of cells throughout the human body through ACE2 and TMPRSS2 receptors (2) and is believed to have caused a spike in a rare syndrome: “multi-system inflammatory state requiring intensive care” in children. Furthermore, the mode of transmission and the extent of environmental contamination is yet unknown. While the virus may not technically be airborne, it is definitely borne in the air as aerosols (3).
One of the most critical unanswered questions is why some COVID-19 patients develop severe disease, while others do not? Does the answer hidden in the origin and continuing evolution of SARS-CoV-2 virus mutation into mild and wild different strains (4)? Alternatively, does the answer depend on the two phases of the individual human body immune responses; a protective phase and a damaging phase due to inflammation-cytokine storms (5)? Other questions include whether bacterial co-infections such as bacterial pneumonia and sepsis with antibiotic resistance lead to increased COVID-19 disease severity and mortality (6) and how long it will take to create an effective vaccine. Potential SARS-CoV-2 vaccines have a variety of approaches that depend on viral life cycles (7), and it is estimated that a vaccine will either arrive in 1 or 2 years or will never arrive at all. Even if the vaccine trials are successful, will the new vaccine cover all SARS-CoV-2 mutant strains, and give full immunity to everyone with no issues when translation to clinical practice? Can we produce enough, how much will it cost and who will pay (a particularly important issue in developing countries)? Can the new vaccine stop the threat of a second inevitable wave of coronavirus, or other pandemic viruses emerging to produce a similar situation in the future?
Gas therapy is a highly effective viral inactivation strategy. Carbon monoxide (CO) gas is very flammable and highly poisonous and referred to as the “Silent Killer,” because it binds to the parts of human blood that carry oxygen molecules, so it chemically blocks the body and organs from getting the needed oxygen. However, CO gas has also been shown to have antimicrobial and antiviral activities against infected cells (8), and two clinical trials (NCT02425579, NCT03799874) have demonstrated that the administration of low concentrations of CO is well-tolerated and safe in patients with sepsis-induced ARDS (9, 10). Similarly, while high concentrations of inhaled ozone (O3) can damage the lungs, cause chest pain, coughing, shortness of breath, throat irritation, and worsen chronic respiratory diseases such as asthma as well as compromise the ability of the body to fight respiratory infections (11), ozone gas therapy has been demonstrated to inactivate airborne viruses (12) and could inactivate the SARS-CoV-2 virus through oxidizing the sulfhydryl groups in cysteine of the virus-cell (13). There are also at least four ongoing clinical trials (NCT04290871 - NCT04306393 - NCT04305457 - NCT04290858) testing the use of inhaled nitric oxide (NO) gas for patients with COVID-19 (14), as increasing airway NO levels via gas inhalation or precursor molecules may improve oxygenation in COVID-19 subjects (15). As with the other gases, there is another side to NO, which can be harmful due to the formation of highly toxic and irritating nitrogen dioxide (NO2) gas and methemoglobinemia (16).
The Hypothesis and Evidence
Carbon dioxide (CO2) is a fundamental biological gas and has been used for medical purposes for over a century due to its unique properties (Figure 1). Carbon dioxide gas is natural, biocompatible, chemically stable, and safer than any other medical gases (NO, O3, or CO). It has been shown to possess antioxidant and anti-inflammatory properties, to improve blood oxygenation and enhance oxygen delivery to organs, to protect and improve lung function, to function as a carrier, or enhancer gas for drug delivery by rapid and direct open airway inhalation with easy administration in home, GP, emergency unit, and ICU settings. These unique biological, physical, and medical properties of CO2 make it a promising anti-COVID-19 therapy for mitigation and suppression of SARS-CoV-2 infection. Our hypothesis depends on inhaling precise doses of humidified and warmed CO2 medical gas, either alone or as a composite carrier gas with other COVID-19 inhaler medications (bronchodilators, antivirals, antibiotics, or anti-cytokine agents), to disinfect the SARS-CoV-2 virus inside the infected human lung, as a preventative measure to stop coronavirus infection spreading, and to improve the treatment of mild, moderate, and severe COVID-19 symptoms. The following benefits and evidence of using medical carbon dioxide gas support the hypothesis.
Universal Virucidal and Antimicrobial Activity
Direct inactivation technologies have several limitations against the current virus. Moist, warm CO2 gas could become a competitive disinfection technology. Carbon dioxide gas is an antiviral, antibacterial, and anti-infection agent effective not only on solid surfaces but also in aqueous solutions and water treatment settings (17). Heated, un-pressurized carbon dioxide bubbled through wastewater or aqueous media effectively destroys both waterborne bacteria and viruses (18). Moreover, supercritical CO2 can in-activate and eliminate coronaviruses from an animal, human tissues and solid surfaces (19–21). Supercritical CO2 offers a novel, user-friendly process to sterilize acellular tissue, such as lung matrices, for use in tissue and organ engineering (22). CO2 can also enhance the effect of some other antibacterial agents, further improving the protection imparted (17). When breathing is impaired, CO2-levels in the human body drop, which creates a favorable environment for bacterial growth and a higher risk of infection. Pure CO2 significantly decreased the growth rate of most viruses and bacteria at body temperature; this inhibitory effect of CO2 increased exponentially with time (23). This phenomenon could be attributed to unravels the secret of structure and function of the Endothelial Surface Layer (ESL) (24–27). As the venous ESL is probably comprised of nanobubbles of CO2, generated from tissue metabolism, that presumably kills the viruses and bacteria exiting to the blood flow on the way to leaving via the lungs (27). Even though the mechanism of inactivation of microorganisms by CO2 is not yet resolved, there are a number of hypotheses that have been proposed to explain the unique disinfection action of CO2 gas (28).
CO2 gas is far superior to other similar gases, with much higher viral inactivation rates at lower temperatures (18–100°C) without the need for pressurization (18, 29). CO2 interacts with water moisture to generate carbonic acid (pH 4.18), a reduced pH could affect virus and microbial cell inactivation, as lipid membrane stability is disrupted and permeability to carbon dioxide increases (30, 31). However, a reduction in the pH of the medium is not sufficient to account for the antimicrobial action of CO2, since it shows a specific inhibitory effect which is greater than that of the other acids used to lower the pH of media (hydrochloric acid, phosphoric acid, etc.) (32). These acids do not penetrate the microbial cells as easily as carbon dioxide (33). Cheng et al. believe that CO2 molecules could enter virus capsids much more easily than H+ and inactivate the virus (34). CO2-protein binding could also damage the capsid, inactivating the virus. Both mechanisms may be active during dense phase carbon dioxide treatment (DPCD) which has also been shown to effectively inactivate viruses (31). The warm atmospheric pressure CO2 gas during DPCD is suggested to have high viral inactivation effect by penetrating the virus capsid due to the high density of CO2 with a high interfacial area (α) produced by the continuous CO2-moist contact surface area (29). Following this; CO2 can bind inside the capsid proteins through acid/base interactions (35), producing the high virus inactivation rates (18). Also, when compared with other gases (Air, O2, N2, and Argon), CO2 gas has the highest inactivated viruses and bacteria rates in different NaCl solutions, even at ambient temperatures and normal atmospheric pressure (18). Recently, Edwards et al. demonstrate the effectiveness of aerosol administration of nasal saline comprising calcium and sodium salts diminishes exhaled particles and acts as a new natural defense against airborne pathogens in the human airways (36). Moreover, Zare and his teamwork report that spraying micron-sized water droplets can act as an effective disinfectant by causing inactivation of over 98% of the bacteria. They propose that the combined action of reactive oxygen species present in micron-size water droplets (but not in bulk water) along with the droplet surface charge is responsible for the observed bactericidal activity (37). The efficiency of CO2 technology will require adjustment and control of the mechanical and dynamic behavior of moist CO2 bubbles and properties such as temperature, flow and density rates, pressure, electrolyte pH, bubble size and thickness, surfaces area, and duration. All of these factors contribute to the observed fast microbial death (38).
Safe and Tolerance for Human Clinical Trials and Treatment
Carbon dioxide (CO2) gas is natural, inexpensive, non-toxic at low concentrations (5,000 ppm), non-flammable, and readily available in high purity from a variety of sources. When CO2 gas dissolves in water, it exists in chemical equilibrium with carbonic acid (pH = 4.18) which plays an essential role in the bicarbonate buffer system used to maintain acid-base homeostasis in the human body. The duration and concentration of carbon dioxide inhalation may be the key to the effective and protective role of CO2 gas therapy. A recent study investigated that pre-treatment by CO2 inhalation for 10 min, but not for 60 min, could improve lipopolysaccharide LPS-induced lung injury (39). A pre-clinical sheep model used perflubron combined with 12% CO2 to re-open constricted airways treatment for severe acute asthma (40). As a reference, OSHA has set a CO2 permissible exposure limit (PEL) of 5,000 ppm over 8 h and 30,000 ppm over 10 min. This compares favorably to CO gas at 50 ppm, NO gas at 25 ppm, and O3 gas at 0.10 ppm for 8 h. Humans can tolerate up to 10% CO2 before severe adverse effects are encountered (41) although CO2 tolerance decreases with age (p <0.0001) (42). Two clinical trials (NCT02616770 & NCT02334553) showed that perflubron carried in gas with ascending doses of carbon dioxide (4, 8, and 12% CO2) administered to healthy subjects was safe and effective in subjects with mild asthma (43, 44), while other ongoing clinical trials (NCT03903913) are testing the safety and tolerability the same formulation in subjects with cystic fibrosis. Moreover, CO2 concentrations of up to 35% have been applied in other clinical trial study used “CO2 inhalation challenge model” through a protected inhalation system to measure the anxiolytic and panicolytic effects of new test compounds (45, 46).
Suppressing Cytokine Storm
Evidence is accumulating inferring that a subcategory of patients with acute COVID-19 might experience cytokine storm syndrome (47). CO2 gas is one of the potential treatment strategies to dampen an overactive immune system and to quell a cytokine storm (48, 49). Many researchers have reported that the presence of CO2 reduces the production of proinflammatory cytokines such as tumor necrosis factor-alpha (TNF-α) and interleukins 1 and 6 (IL-1 and IL-6, respectively), suggesting that the gas temporarily inhibits macrophage activity via a mechanism that could be associated with the reduction of the local or systemic pH (50–54). Carbon dioxide gas can also affect the production of pro-and anti-inflammatory cytokines in endotoxin-stimulated human whole blood cultures under hypercapnic, normocapnic, and hypocapnic conditions (55). In another study, CO2 was shown to differentially affect the cytokine release of macrophage subpopulations exclusively via alteration of extracellular pH. Decreasing the extracellular pH to 6.5 mimicked the effects of CO2 and a decrease to 5.5 suppressed IL-6 release in cell lines (53).
Inhaled Carrier Gas Delivery System
CO2 gas has unique safety, chemical stability, biocompatibility, and properties as well as a higher density than oxygen, high solubility in tissue and blood and high tolerance in vascular system (56). CO2 itself is a respiratory stimuli, enhances mucus clearance, and seems to be a bronchodilator by general induction of smooth muscle relaxation (57). Additionally, warmed and humidified CO2 insufflation leads to an improved body core temperature (BCT) maintenance, a reduction of the inflammatory and cytokine responses (58, 59) and improved quality of postoperative course, compared with standard insufflation (60, 61). Also, it can reduce intraoperative hypothermia, coagulation dysfunction, early postoperative cough pain, days to first flatus and solid food intake, and the length of hospital stays (62). In recent years, CO2-based technologies have accordingly gained considerable interest in the pharmaceutical industry. CO2 bubble-generating carrier systems can be used to locally accumulate a drug at diseased tissue, reducing side effects on the healthy tissue and improving their therapeutic effectiveness (63). CO2 may also be used as an enhancer and carrier gas for delivery of effective medical agents into a surgical wound (64) or respiratory diseases such as severe acute asthma and cystic fibrosis (40, 43, 44).
Clinical Usage and Medical Purposes
Medical carbon dioxide has been used as a pure gas or in specialized mixtures with other gases in anesthesia, as an insufflation gas for minimally invasive surgery (65), and in carboxytherapy (66). It can be used in the expansion of blood vessels to increase carbon dioxide level after rapid breathing, and to stimulate breathing after a period of non-breathing (67). Transdermal carbon dioxide gas therapy is widespread and uses carbon dioxide gas at high humidity, to increase tissue blood flow. Tissue oxygenation generates new blood vessels, and well-oxygenated tissues improve the effectiveness of antibiotic therapy. This is complemented by the antioxidant effect of CO2 itself, which reduces oxidative stress in open surgery (68), and improves wound healing (69).
Benefits of Hypercapnic Therapy
Hypercapnic therapy (elevated CO2 levels) has beneficial effects on the physiology of the respiratory, cardiovascular, and nervous system. In human critical care, hypercapnic acidosis (HCA) is frequently acceptable and improves innate immune function, resistance to infection, and protects and improves lung functions in patients with advanced lung disease. However, all these benefits require careful consideration of when and for how long hypercapnia will be applied. Hypercapnic acidosis, but not buffered hypercapnia, was reported to reduce the severity of sepsis-induced lung injury (70). Recent studies suggest that HCA is protective in the earlier phases of bacterial pneumonia-induced sepsis, just as HCA is protective in preclinical models of early and prolonged systemic sepsis (71). Also, CO2 gas in therapeutic hypercapnia and other forms of acidosis techniques is an excellent antioxidant and anti-inflammatory agent (72). Hypercapnic acidosis was associated with benefits on lung and distant organs in several disease models, apart from the reduction of ventilation parameters such as ventilator-induced lung injury (73), acute respiratory distress syndrome (ARDS) (74), ischemia-reperfusion injury (75) and sepsis (76), therapeutic hypercapnia through inspired carbon dioxide attenuated lung injury, as measured by gas exchange, reduced cytokine release, lung oedema formation, and histological lung injury. Hypercapnic acidosis improves ventilation-perfusion matching that also improves gas exchange (77), prevents oedema formation (78), clears the alveolar fluid in pulmonary oedema (79), maintains the integrity of the blood-brain-barrier and reduces neurologic deficits after trauma (80). HCA also reduces the oxidative stress that contributes to pathologic thick mucus gel formation in the lung (81, 82). It is hoped that hypercapnia therapy may offer real benefits, but well-planned and executed clinical studies will be required.
Recent COVID-19 Contradictory Studies
The partial pressure of CO2 in the atmosphere varies between 0.03 and 0.06% (83) but forms a high proportion (12.5–13.5%) with water vapor (1.3%) of the mainstream cigarette smoke (84). Recent studies have discovered the unusually low prevalence of current smoking was observed among hospitalized COVID-19 patients compared to the expected prevalence based on smoking prevalence in China. This preliminary analysis does not support the argument that current smoking is a risk factor for hospitalization for COVID-19, and might even suggest a protective role (85). Other cross-sectional studies in both COVID-19 out- and in-patients strongly suggests that daily smokers have a very much lower probability of developing symptomatic or severe SARS-CoV-2 infection as compared to the general population (86, 87). However, on the other hand, researchers at Baylor College of Medicine, the University of South Carolina and other institutions have identified tobacco smoking as a potential risk factor for infection of the COVID-19 virus, due to increasing the expression of ACE2, the receptor of SARS-CoV-2, in the lungs (88, 89). These two contradictory studies support our hypothesis of moist warm CO2 gas resulted from cigarettes smoking could kill the SARS-CoV-2 viruses inside the infected lungs of smoker patients, and that leads to decreasing the infected COVID-19 patient from the smoker, not the nicotinic or other outcomes of mainstream cigarette.
Testing the Hypothesis (a): Preclinical Study and Inactivation Mechanisms
Herein, we recommend preclinical studies to optimize the relation between disinfection efficacy and toxicity level of warm humidified CO2 gas while considering other related parameters to discover the possible mechanism of action of disinfection by CO2 gas. The temperature inside healthy lungs is around 37°C, the pH is between 7.38 and 7.42, and the relative humidity ranges from 30 to 70%. It is essential to keep humidity stable as too high humidity provides optimal conditions for microbial growth, and low humidity and dry air can dry mucous membranes and make them more susceptible to infection (90). The SARS-CoV-2 virus is highly stable at 4°C, but it is very sensitive to heat. It is remarkably stable in a wide range of pH values (pH 3–10) at room temperature (22°C) (91, 92). However, the stability of SARS-CoV-2 under different environmental conditions of temperature, pressure, relative humidity, and pH with biological tissue and barriers require further investigation.
Testing the Hypothesis (b): Clinical Evaluation and Implications
Whilst the properties and clinical applications of CO2 have been known for many decades; parameters must be systematically studied before it can be used in a new clinical setting.
(I) Healthy, Non-symptomatic, Mild, and Moderate Care Levels
Optimizing the balance between disinfection efficacy and toxicity of humidified warmed CO2 gas considering other parameters (temperature, relative humidity, pressure flow and density rates, electrolyte pH, bubble size and thickness, surfaces area, and duration) will be key. Different regimes will be needed to protect healthy and non-symptomatic patients and improve the condition of those suffering mild and moderate COVD-19 symptoms. Multiple-ascending dose studies in which subjects with mild to moderate COVID-19 will be enrolled [CO2 max 14%, tolerance decreases with age (p <0.0001)] (42). The suggested study could consist of a screening period, a run-in, dosing and evaluation periods, and a follow-up period. The dosing and evaluation period of the study could divide into three connected components. First, a dose-escalation study—This segment of the treatment period is designed to assess the safety and tolerability of escalating doses of medical CO2 gas (2–4%) in a healthy volunteer (Figure 2), and (4, 8, 12, and 14%) in those with mild-moderate COVID-19 symptoms (Figure 3). Second, a daily dosing study- This segment of the treatment period is designed to assess the short term (5 days) safety and tolerability of 1–2 times daily administrations of a fixed dose of medical CO2 gas in healthy volunteers, and 2–3 times daily administration of a fixed dose of medical CO2 gas in patients with mild-moderate COVID-19. Third, a drug delivery study- This segment of the treatment period is designed to assess the safety, efficacy, enhancing, and tolerability of humidified warmed CO2 gas (2–14%) composed with other inhaled medication such as an antiviral (Remdesivir or IFN-β SNG001), short-acting bronchodilator, antibiotic, anti-inflammation. The recommended clinical trial study may well-include placebo-control, humidified warmed CO2 gas (2–14%), and humidified warmed CO2 gas (2–14%) composed with other inhaled medication. Administration can be achieved through using simple comprised cartridge MDI puffer, portable nebulizer, or circularize II high-efficiency aerosol drug delivery system nebulizer in a negative pressure environment. Direct air/oxygen inhalation for a few minutes can be used to recover patients to baseline carbon dioxide levels. A safety monitoring committee must also review the results from each cohort before deciding continuation of the study at the next prescribed dose level, based on consideration of the clinical significance of safety and tolerability parameters.
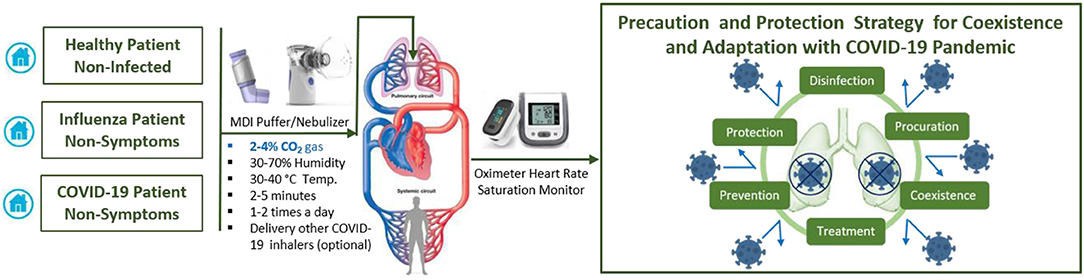
Figure 2. Suggested protocol for early and daily inhaling CO2 gas (2–4%) itself or composite with other COVID-19 inhaler medications could help in precaution and protection strategy to coexistence and adaptation with COVID-19 pandemic.
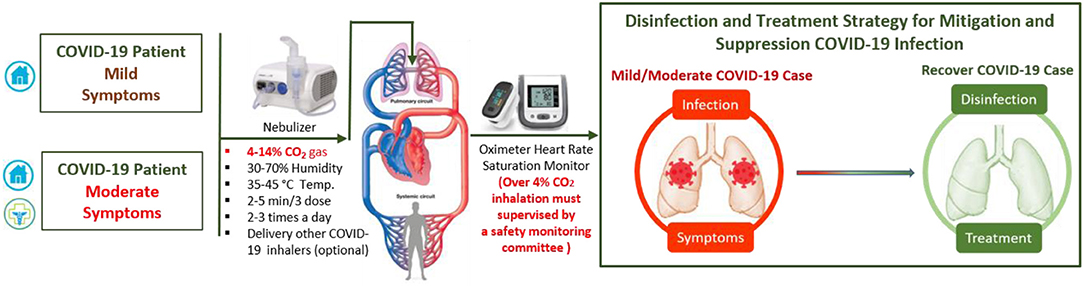
Figure 3. Suggested protocol for early inhaling CO2 gas (4–14%) itself or composite with other COVID-19 inhaler medications could save lives by disinfection and improve mild and moderate COVID-19 patient treatment.
(II) Severe Care Level
The damage mechanisms of SARS-CoV-2 are still unclear, with severe COVID-19 cases are complicated by high mortality rates due to compromised immune function and a high probability of antibiotic-resistant secondary infections. Most severe COVID-19 cases are associated with respiratory failure, with many already suffering from internal high hypercapnia acidosis (with humidity levels near 100%) that disrupt not only cardiac and neurological functions but also immune system function by suppressing both innate and adaptive immune responses to viral and bacterial proliferation and infection (54, 93–96). This dysfunction of the immune system with increasing SARS-CoV-2 infection can lead to an overreaction of the immune system (cytokine storm), during which white blood cells are misdirected to attack and inflame even healthy tissue, leading to failure of the lungs, heart, liver, intestines, kidneys, and genitals (Multiple Organ Dysfunction Syndrome, MODS). This may, in turn, lead to the lungs shutting down (Acute Respiratory Distress Syndrome, ARDS), which makes absorption of oxygen difficult. Most deaths due to COVID-19 are due to respiratory failure. To save the lives of severing COVID-19 patients, we must first stop the causes of SARS-CoV-2 infection and preventing secondary infections. However, due to the absence of a specific COVID-19 antiviral treatment, most severe COVID-19 patients be admitted to the intensive care unit to fight the symptoms, aiming to lower the mortality rate through intensive monitoring and supportive organ function treatments by anti-cytokine medications with artificial blood purification system machines (97). Herein, we cautiously suggest that external artificial hypercapnia acidosis (warmed humidified CO2 15–25%) could be applied to disinfect and stabilize the lungs of SARS-CoV-2 infected patients and prevent secondary infections (Figure 4). However, it should only be considered for severely affected patients if they are already is connected to life support and artificial blood purification through mechanical means, and a controlled gas mixture consisting of 25% CO2 and 75% O2 is delivered through a protected inhalation system while monitoring a wide range of physiological parameters, and administering supportive organ function treatments.
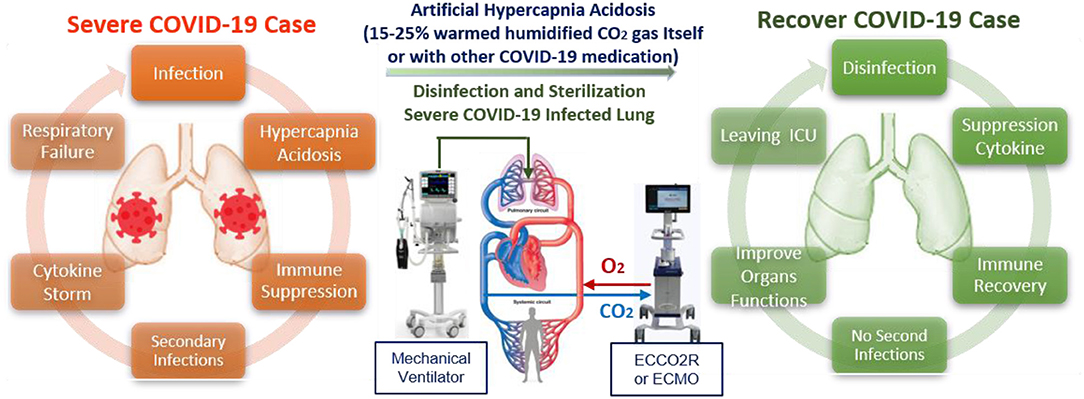
Figure 4. Suggested supporting treatment protocol depending on time and duration of limited and convenient artificial hypercapnia acidosis (15–25%) that could help in saving more lives of severe COVID-19 patients.
Conclusion and Expecting Outcomes
There is an urgent global need for a universal vaccine to cover all SARS-CoV-2 mutant strains to stop the threat of an inevitable second wave of coronavirus. Currently, there are hundreds of clinical trials, but not yet any approved antiviral drugs specific for the treatment of COVID-19. The physical, biological, and medical properties of CO2 gas suggest that humified warmed CO2 gas possesses multiple bioactivities and offer a new concept to SARS-CoV-2 viral disinfection and COVID-19 treatment. This inexpensive and broadly applicable therapy could lead to a massive reduction in the global number of infected, especially when used as a carrier for delivery of other inhaled drugs and creates new possibilities for mitigation and suppression of any COVID-19 second wave, or indeed any new future respiratory viral pandemic. In the future, more bioactive properties of CO2 could be identified, and their mechanisms of action investigated. We believe well-designed clinical trials of CO2 and its various bioactive properties are warranted to examine its efficacy against these diseases in human beings. It is hoped that this hypothesis will serve as a stimulus for further investigation into this issue.
Data Availability Statement
The original contributions presented in the study are included in the article/supplementary material, further inquiries can be directed to the corresponding author/s.
Author Contributions
All authors contributed to the article and approved the submitted version. AE-B: conceptualization, methodology, writing—original draft, and writing—review and editing. EB: writing—review and editing. MG and KH: conceptualization and writing—review and editing.
Conflict of Interest
The authors declare that the research was conducted in the absence of any commercial or financial relationships that could be construed as a potential conflict of interest.
Acknowledgments
We thank our colleagues and the members of RIS at Cardiff University for comments on the manuscript, R. Mart, R. Weiser, A. Staphnill, R. Turner, M. Morgan, and C. Butler. Also, we thank The College of Biomedical and Life Sciences (BLS) at Cardiff University for financial support through the Wellcome Trust Institutional Strategic Support Fund (ISSF). This manuscript has been released as a pre-print at OSF Preprints (98).
References
1. Oxley TJ, Mocco J, Majidi S, Kellner CP, Shoirah H, Singh IP, et al. Large-vessel stroke as a presenting feature of covid-19 in the young. N Engl J Med. (2020) 382:e60. doi: 10.1056/NEJMc2009787
2. Sungnak W, Huang N, Bécavin C, Berg M, Queen R, Litvinukova M, et al. SARS-CoV-2 entry factors are highly expressed in nasal epithelial cells together with innate immune genes. Nat Med. (2020) 26:681–7. doi: 10.1038/s41591-020-0868-6
3. Liu Y, Ning Z, Chen Y, Guo M, Liu Y, Gali NK, et al. Aerodynamic analysis of SARS-CoV-2 in two Wuhan hospitals. Nature. (2020) 582:557–60. doi: 10.1038/s41586-020-2271-3
4. Tang X, Wu C, Li X, Song Y, Yao X, Wu X, et al. On the origin and continuing evolution of SARS-CoV-2. Natl Sci Rev. (2020) 3:nwaa036. doi: 10.1093/nsr/nwaa036
5. Shi Y, Wang Y, Shao C, Huang J, Gan J, Huang X, et al. COVID-19 infection: the perspectives on immune responses. Cell Death Differ. (2020) 27:1451–4. doi: 10.1038/s41418-020-0530-3
6. Chen N, Zhou M, Dong X, Qu J, Gong F, Han Y, et al. Epidemiological and clinical characteristics of 99 cases of 2019 novel coronavirus pneumonia in Wuhan, China: a descriptive study. Lancet. (2020) 395:507–13. doi: 10.1016/S0140-6736(20)30211-7
7. Callaway E. The race for coronavirus vaccines: a graphical guide. Nature. (2020) 580:576–7. doi: 10.1038/d41586-020-01221-y
8. Ma Z, Pu F, Zhang X, Yan Y, Zhao L, Zhang A, et al. Carbon monoxide and biliverdin suppress bovine viral diarrhoea virus replication. J Gen Virol. (2017) 98:2982–92. doi: 10.1099/jgv.0.000955
9. Fredenburgh LE, Kraft BD, Hess DR, Harris RS, Wolf MA, Suliman HB, et al. Effects of inhaled CO administration on acute lung injury in baboons with pneumococcal pneumonia. Am J Physiol Lung Cell Mol Physiol. (2015) 309:L834–46. doi: 10.1152/ajplung.00240.2015
10. Fredenburgh LE, Perrella MA, Barragan-Bradford D, Hess DR, Peters E, Welty-Wolf KE, et al. A phase I trial of low-dose inhaled carbon monoxide in sepsis-induced ARDS. JCI Insight. (2018) 3:e124039. doi: 10.1172/jci.insight.124039
11. Barrese E, Gioffrè A, Scarpelli M, Turbante D, Trovato R, Iavicoli S. Indoor pollution in work office: VOCs, formaldehyde and ozone by printer. Occupat Dis Environ Med. (2014) 3:49–55. doi: 10.4236/odem.2014.23006
12. Dubuis ME, Dumont-Leblond N, Laliberté C, Veillette M, Turgeon N, et al. Ozone efficacy for the control of airborne viruses: bacteriophage and norovirus models. PLoS ONE. (2020) 15:e0231164. doi: 10.1371/journal.pone.0231164
13. Rowen RJ, Robins H. A plausible “Penny” costing effective treatment for corona virus ozone therapy. J Infect Dis Epidemiol. (2020) 6:113. doi: 10.23937/2474-3658/1510113
14. Matthay MA, Aldrich JM, Gotts JE. Treatment for severe acute respiratory distress syndrome from COVID-19. Lancet Respir Med. (2020) 8:433–4. doi: 10.1016/S2213-2600(20)30127-2
15. Martel J, Ko YF, Young JD, Ojcius DM. Could nasal nitric oxide help to mitigate the severity of COVID-19? Microb Infect. (2020) 22:168–71. doi: 10.1016/j.micinf.2020.05.002
16. Ichinose F, Roberts JD Jr, Zapol WM. Inhaled nitric oxide: a selective pulmonary vasodilator: current uses and therapeutic potential. Circulation. (2004) 109:3106–11. doi: 10.1161/01.CIR.0000134595.80170.62
17. Martirosyan V, Hovnanyan K, Ayrapetyan S. Carbon dioxide as a microbial toxicity enhancer of some antibacterial agents: a new potential water purification tool. ISRN Biophys. (2012) 2012:906761. doi: 10.5402/2012/906761
18. Garrido Sanchis A, Pashley R, Ninham B. Virus and bacteria inactivation by CO2 bubbles in solution. NPJ Clean Water. (2019) 2:5. doi: 10.1038/s41545-018-0027-5
19. Fages Jacques Patrick Frayssinet and Gilbert Bonel. “Uses for a current of supercritical carbon dioxide as an antiviral agent.” U.S. Patent No. 5,723,012. 3 Mar. 1998. Washington, DC: U.S. Patent and Trademark Office.
20. Fages J, Frayssinet P, Bonel G. Antiviral treatment of collagenous material for use as prostheses and grafts-by treating with supercritical carbon di:oxide, hydrogen peroxide, sodium hydroxide and ethanol, preventing viral contamination from e.g. Hepatitis C. Patent EP748632-A1; FR2735372-A1; US5723012-A; EP748632-B1; DE69619893-E; ES2174035-T3. Bioland Sarl; Depuy Bioland (1997).
21. Qiu Y, Lin G, Zhang M, Chen Q. Method for removing the activity of coronavirus by using supercritical fluid. Patent CN1721526-A; CN1318581-C. Nanwei Ind Co Ltd. (2006).
22. Balestrini JL, Liu A, Gard AL, Huie J, Blatt KMS, Schwan J, et al. Sterilization of lung matrices by supercritical carbon dioxide. Tissue Eng C Methods. (2016) 22:260–9. doi: 10.1089/ten.tec.2015.0449
23. Persson M, Svenarud P, Flock JI, Van Der Linden J. Carbon dioxide inhibits the growth rate of Staphylococcus aureus at body temperature. Surg Endosc Other Interv Tech. (2005) 19:91–4. doi: 10.1007/s00464-003-9334-z
24. Rizzo AN, Dudek SM. Endothelial glycocalyx repair: building a wall to protect the lung during sepsis. Am J Respir Cell Mol Biol. (2017) 56:687–88. doi: 10.1165/rcmb.2017-0065ED
25. Curry FE. The molecular structure of the endothelial glycocalyx layer (EGL) and surface layers (ESL) modulation of transvascular exchange. Adv Exp Med Biol. (2018) 1097:29–49. doi: 10.1007/978-3-319-96445-4_2
26. Sieve I, Münster-Kühnel AK, Hilfiker-Kleiner D. Regulation and function of endothelial glycocalyx layer in vascular diseases. Vascul Pharmacol. (2018) 100:26–33. doi: 10.1016/j.vph.2017.09.002
27. Reines BP, Ninham BW. Structure and function of the endothelial surface layer: unraveling the nanoarchitecture of biological surfaces. Q Rev Biophys. (2019) 52:e13. doi: 10.1017/S0033583519000118
28. Isenschmid A, Marison I, Von Stockar U. The influence of pressure and temperature of compressed CO2 on the survival of yeast cells. J Biotechnol. (1995) 39:229–37. doi: 10.1016/0168-1656(95)00018-L
29. Garrido A, Pashley R, Ninham B. Water sterilisation using different hot gases in a bubble column reactor. J Environ Chem Eng. (2018) 6:2651–9. doi: 10.1016/j.jece.2018.04.004
30. Lin HM, Yang Z, Chen LF. Inactivation of saccharomyces cerevisiae by supercritical and subcritical carbon dioxide. Biotechnol Progr. (1992) 8:458–61. doi: 10.1021/bp00017a013
31. Balaban MO, Ferrentino G. Dense Phase Carbon Dioxide: Food and Pharmaceutical Applications. John Wiley and Sons. Ames, IA (2012). doi: 10.1002/9781118243350
32. Becker Z. A comparison between the action of carbonic acid and other acids upon the living cell. Protoplasma. (1936) 25:161–75. doi: 10.1007/BF01839067
33. Debs-Louka E, Louka N, Abraham G, Chabot V, Allaf K. Effect of compressed carbon dioxide on microbial cell viability. Appl Environ Microbiol. (1999) 65:626–31. doi: 10.1128/AEM.65.2.626-631.1999
34. Cheng X, Imai T, Teeka J, Hirose M, Higuchi T, Sekine M. Inactivation of bacteriophages by high levels of dissolved CO2. Environ Technol. (2013) 34:539–44. doi: 10.1080/09593330.2012.704403
35. Cundari TR, Wilson AK, Drummond ML, Gonzalez HE, Jorgensen KR, Payne S, et al. CO2-formatics: how do proteins bind carbon dioxide? J Chem Inform Model. (2009) 49:2111–15. doi: 10.1021/ci9002377
36. Edwards D, Hickey A, Batycky R, Griel L, Lipp M, Dehaan W, et al. A new natural defense against airborne pathogens. QRB Discov. (2020) 1:e5. doi: 10.1017/qrd.2020.9
37. Dulay MT, Lee JK, Mody AC, Narasimhan R, Monack DM, Zare RN. Spraying small water droplets acts as a bacteriocide. QRB Discov. (2020) 1:e3. doi: 10.1017/qrd.2020.2
38. Spilimbergo S, Bertucco A. Non-thermal bacterial inactivation with dense CO2. Biotechnol Bioeng. (2003) 84:627–38. doi: 10.1002/bit.10783
39. Tang SE, Wu SY, Chu SJ, Tzeng YS, Peng CK, Lan CC, et al. Pre-treatment with ten-minute carbon dioxide inhalation prevents lipopolysaccharide-induced lung injury in mice via down-regulation of toll-like receptor 4 expression. Int J Mol Sci. (2019) 20:6293. doi: 10.3390/ijms20246293
40. E Mays T, Choudhury P, Leigh R, Koumoundouros E, Velden J, Shrestha G, et al. Nebulized perflubron and carbon dioxide rapidly dilate constricted airways in an ovine model of allergic asthma. Respir Res. (2014) 15:98. doi: 10.1186/s12931-014-0098-x
41. Zaba C, Marcinkowski JT, Wojtyła A, Tezyk A, Tobolski J, Zaba Z. Acute collective gas poisoning at work in a manure storage tank. Ann Agric Environ Med. (2011) 18:448–51.
42. Gill M, Natoli MJ, Vacchiano C, MacLeod DB, Ikeda K, Qin M, et al. Effects of elevated oxygen and carbon dioxide partial pressures on respiratory function and cognitive performance. J Appl Physiol. (2014) 117:406–12. doi: 10.1152/japplphysiol.00995.2013
43. Green FHY, Leigh R, Fadayomi M, Lalli G, Chiu A, Shrestha G, et al. A phase I, placebo-controlled, randomized, double-blind, single ascending dose-ranging study to evaluate the safety and tolerability of a novel biophysical bronchodilator (S-1226) administered by nebulization in healthy volunteers. Antimicrob Agents Chemother. (2016) 61:e00279–17. doi: 10.1186/s13063-016-1489-8
44. Swystun V, Green FHY, Dennis JH, Rampakakis E, Lalli G, Fadayomi M, et al. A phase IIa proof-of-concept, placebo-controlled, randomized, double-blind, crossover, single-dose clinical trial of a new class of bronchodilator for acute asthma. Trials. (2018) 19:321. doi: 10.1186/s13063-018-2720-6
45. Leibold NK, van den Hove DLA, Viechtbauer W, Buchanan GF, Goossens L, Lange I, et al. CO2 exposure as translational cross-species experimental model for panic. Transl Psychiatry. (2016) 6:e885. doi: 10.1038/tp.2016.162
46. Vickers K, Jafarpour S, Mofidi A, Rafat B, Woznica Linett A. The 35% carbon dioxide test in stress and panic research: overview of effects and integration of findings. Clin Psychol Rev. (2012) 32:153–64. doi: 10.1016/j.cpr.2011.12.004
47. Yuen KS, Ye ZW, Fung SY, Chan CP, Jin DY. SARS-CoV-2 and COVID-19: the most important research questions. Cell Biosci. (2020) 10:40. doi: 10.1186/s13578-020-00404-4
48. Roberto Rodrigues Bicalho P, Magna Ribeiro F, Henrique Ferreira Marçal P, Gomes de Alvarenga D, de Sá Silva F. Does helium pneumoperitoneum reduce the hyperinflammatory response in septic animals during laparoscopy? Surg Res Pract. (2020) 2020:5738236. doi: 10.1155/2020/5738236
49. Lu Z, Casalino-Matsuda SM, Nair A, Buchbinder A, Budinger GRS, Sporn PHS, et al. A role for heat shock factor 1 in hypercapnia-induced inhibition of inflammatory cytokine expression. FASEB J. (2018) 32:3614–22. doi: 10.1096/fj.201701164R
50. West MA, Baker J, Bellingham J. Kinetics of decreased LPS-stimulated cytokine release by macrophages exposed to CO2. J Surg Res. (1996) 63:269–74. doi: 10.1006/jsre.1996.0259
51. Jacobi CA, Ordemann J, Halle E, Volk HD, Müller JM. Impact of laparoscopy with carbon dioxide versus helium on local and systemic inflammation in an animal model of peritonitis. J Laparoendosc Adv Surg Tech. (1999) 9:305–12. doi: 10.1089/lap.1999.9.305
52. Matsumoto T, Tsuboi S, Dolgor B, Bandoh T, Yoshida T, Kitano S. The effect of gases in the intraperitoneal space on cytokine response and bacterial translocation in a rat model. Surg Endosc. (2001) 15:80–84. doi: 10.1007/s004640000293
53. Kos M, Kuebler J, Jesch N, Vieten G, Bax N, van der Zee D, et al. Carbon dioxide differentially affects the cytokine release of macrophage subpopulations exclusively via alteration of extracellular Ph. Surg Endosc Other Int Tech. (2006) 20:570–6. doi: 10.1007/s00464-004-2175-6
54. Hanly EJ, Aurora AR, Fuentes JM, Shih SP, Marohn MR, De Maio A, et al. Hypercapnia and acidosis in sepsis – anesthesiology. J Gastrointest Surg. (2005) 9:1245–52. doi: 10.1097/ALN.0b013e3181ca361f
55. Kimura D, Totapally BR, Raszynski A, Ramachandran C, Torbati D. The effects of CO2 on cytokine concentrations in endotoxin-stimulated human whole blood. Crit Care Med. (2008) 36:2823–7. doi: 10.1097/CCM.0b013e318186f556
56. Martens S, Neumann K, Sodemann C, Deschka H, Wimmer-Greinecker G, Moritz A. Carbon dioxide field flooding reduces neurologic impairment after open heart surgery. Ann Thoracic Surg. (2008) 85:543–7. doi: 10.1016/j.athoracsur.2007.08.047
57. Van den Elshout F, Van Herwaarden C, Folgering H. Effects of hypercapnia and hypocapnia on respiratory resistance in normal and asthmatic subjects. Thorax. (1991) 46:28–32. doi: 10.1136/thx.46.1.28
58. Oderda M, Cerutti E, Gontero P, Manetta T, Mengozzi G, Meyer N, et al. Standard insufflation during RARP. Minerva Anestesiol. (2018) 84:1228. doi: 10.23736/S0375-9393.18.12695-2
59. Oderda M, Cerutti E, Gontero P, Manetta T, Mengozzi G, Meyer N, et al. The impact of warmed and humidified CO2 insufflation during robotic radical prostatectomy: results of a randomized controlled trial. Urol J. (2019) 86:130–40. doi: 10.1177/0391560319834837
60. Schlotterbeck H, Schaeffer R, Dow WA, Diemunsch P. Cold nebulization used to prevent heat loss during laparoscopic surgery: an experimental study in pigs. Surg Endosc. (2008) 22:2616–20. doi: 10.1007/s00464-008-9841-z
61. Noll E, Schaeffer R, Joshi G, Diemunsch S, Koessler S, Diemunsch P. Heat loss during carbon dioxide insufflation: comparison of a nebulization based humidification device with a humidification and heating system. Surg Endosc. (2012) 26:3622–5. doi: 10.1007/s00464-012-2385-2
62. Jiang R, Sun Y, Wang H, Liang M, Xie X. Effect of different carbon dioxide (CO2) insufflation for laparoscopic colorectal surgery in elderly patients: a randomized controlled trial. Medicine. (2019) 98:e17520. doi: 10.1097/MD.0000000000017520
63. Lin YJ, Huang CC, Wan WL, Chiang CH, Chang Y, Sung HW. Recent advances in CO2 bubble-generating carrier systems for localized controlled release. Biomaterials. (2017) 133:154–64. doi: 10.1016/j.biomaterials.2017.04.018
64. Persson M, van der Linden J. The potential use of carbon dioxide as a carrier gas for drug delivery into open wounds. Med Hypotheses. (2009) 72:121–4. doi: 10.1016/j.mehy.2008.08.026
65. Winkler JL, Jeronimo J, Singleton J, Janmohamed A, Santos C. Performance of cryotherapy devices using nitrous oxide and carbon dioxide. Int J Gynecol Obstetr. (2010) 111:73–77. doi: 10.1016/j.ijgo.2010.04.032
66. Verrier N, Fournier C, Fournel T. 3D tracking the brownian motion of colloidal particles using digital holographic microscopy and joint reconstruction. Appl Opt. (2015) 54:4996–5002. doi: 10.1364/AO.54.004996
67. Yu T, Cheng Y, Wang X, Tu B, Cheng N, Gong J, et al. Gases for establishing pneumoperitoneum during laparoscopic abdominal surgery. Cochrane Database Syst Rev. (2017) 6:CD009569. doi: 10.1002/14651858.CD009569.pub3
68. Tsuchiya M, Sato EF, Inoue M, Asada A. CO2 field flooding may also reduce oxidative stress in open surgery. Anesth Anal. (2009) 109:683–4. doi: 10.1213/ane.0b013e3181a909be
69. Brandi C, Grimaldi L, Nisi G, Brafa A, Campa A, Calabrò M, et al. The role of carbon dioxide therapy in the treatment of chronic wounds. In Vivo. (2010) 24:223–6.
70. Higgins BD, Costello J, Contreras M, Hassett P, O' Toole D, Laffey JG. Differential effects of buffered hypercapnia versus hypercapnic acidosis on shock and lung injury induced by systemic sepsis. Anesthesiology. (2009) 111:1317–26. doi: 10.1097/ALN.0b013e3181ba3c11
71. Curley G, Contreras M, Nichol A, Higgins B, Laffey J. Hypercapnia and acidosis in sepsis. Anesthesiology. (2010) 112:462–72. doi: 10.1097/ALN.0b013e3181ca361f
72. Gates KL, Howell HA, Nair A, Vohwinkel CU, Welch LC, Beitel GJ, et al. Hypercapnia impairs lung neutrophil function and increases mortality in murine pseudomonas pneumonia. Am J Respir Cell Mol Biol. (2013) 49:821–8. doi: 10.1165/rcmb.2012-0487OC
73. Peltekova V, Engelberts D, Otulakowski G, Uematsu S, Post M, Kavanagh BP. Hypercapnic acidosis in ventilator-induced lung injury. Intensive Care Med. (2010) 36:869–78. doi: 10.1007/s00134-010-1787-7
74. Hummler HD, Banke K, Wolfson MR, Buonocore G, Ebsen M, Bernhard W, et al. The effects of lung protective ventilation or hypercapnic acidosis on gas exchange and lung injury in surfactant deficient rabbits. PLoS ONE. (2016) 11:e0147807. doi: 10.1371/journal.pone.0147807
75. Laffey JG, Jankov RP, Engelberts D, Tanswell AK, Post M, Lindsay T, et al. Effects of therapeutic hypercapnia on mesenteric ischemia–reperfusion injury. Am J Respir Crit Care Med. (2003) 168:1383–90. doi: 10.1164/rccm.2108078
76. O'Toole D, Hassett P, Contreras M, Higgins BD, McKeown STW, McAuley DF, et al. Hypercapnic acidosis attenuates pulmonary epithelial wound repair by an NF-κB dependent mechanism. Thorax. (2009) 64:976–82. doi: 10.1136/thx.2008.110304
77. Sinclair SE, Kregenow DA, Starr I, Schimmel C, Lamm WJE, Hlastala MP, et al. Therapeutic hypercapnia and ventilation-perfusion matching in acute lung injury: low minute ventilation vs inspired CO2. Chest. (2006) 130:85–92. doi: 10.1378/chest.130.1.85
78. Ketabchi F, Egemnazarov B, Schermuly RT, Ghofrani HA, Seeger W, Grimminger F, et al. Effects of hypercapnia with and without acidosis on hypoxic pulmonary vasoconstriction. Am J Physiol Lung Cell Mol Physiol. (2009) 297:L977–83. doi: 10.1152/ajplung.00074.2009
79. Briva A, Lecuona E, Sznajder JI. Permissive and non-permissive hypercapnia: mechanisms of action and consequences of high carbon dioxide levels. Arch Bronconeumol. (2010) 46:378–82. doi: 10.1016/S1579-2129(10)70088-4
80. Yang WC, Wang Q, Chi LT, Wang YZ, Cao HL, Li WZ. Therapeutic hypercapnia reduces blood–brain barrier damage possibly via protein kinase Cε in rats with lateral fluid percussion injury. J Neuroinflammation. (2019) 16:36. doi: 10.1186/s12974-019-1427-2
81. Yuan S, Hollinger M, Lachowicz-Scroggins ME, Kerr SC, Dunican EM, Daniel BM, et al. Oxidation increases mucin polymer cross-links to stiffen airway mucus gels. Sci Transl Med. (2015) 7:276ra27. doi: 10.1126/scitranslmed.3010525
82. Fahy JV, Dickey BF. Airway mucus function and dysfunction. N Engl J Med. (2010) 363:2233–47. doi: 10.1056/NEJMra0910061
83. Keeling CD, Whorf TP. Atmospheric CO2 Records from Sites in the Scripps Institution of Oceanography (SIO) Air Sampling Network (1985–2007). (2004). doi: 10.3334/CDIAC/ATG.NDP001
84. Guais A, Brand G, Jacquot L, Karrer M, Dukan S, Grévillot G, et al. Toxicity of carbon dioxide: a review. Chem Res Toxicol. (2011) 24:2061–70. doi: 10.1021/tx200220r
85. Farsalinos K, Barbouni A, Niaura R. Smoking, vaping and hospitalization for COVID-19. Qeios. [Preprint] (2020) doi: 10.32388/Z69O8A.5
86. Miyara M, Tubach F, Amoura Z. Low incidence of daily active tobacco smoking in patients with symptomatic COVID-19. Qeios [Preprint]. (2020) doi: 10.32388/WPP19W
87. Changeux JP, Amoura Z, Rey FA, Miyara M. A nicotinic hypothesis for Covid-19 with preventive and therapeutic implications. Qeios. (2020) 343:33–39. doi: 10.32388/FXGQSB.2
88. Cai G, Bossé Y, Xiao F, Kheradmand F, Amos CI. Tobacco smoking increases the lung gene expression of ACE2, the receptor of SARS-CoV-2. Am J Respir Crit Care Med. (2020) 201:1557–9. doi: 10.1164/rccm.202003-0693LE
89. Patanavanich R, Glantz SA. Smoking is associated with COVID-19 progression: a meta-analysis. Nicotine Tob Res. (2020) 22:1653–6. doi: 10.1093/ntr/ntaa082
90. Lepeule J, Litonjua AA, Gasparrini A, Koutrakis P, Sparrow D, Vokonas PS, et al. Lung function association with outdoor temperature and relative humidity and its interaction with air pollution in the elderly. Environ Res. (2018) 165:110–17. doi: 10.1016/j.envres.2018.03.039
91. Chin AWH, Chu JTS, Perera MRA, Hui KPY, Yen HL, Chan MCW, et al. Stability of SARS-CoV-2 in different environmental conditions. Lancet Microbe. (2020) 1:e10. doi: 10.1016/S2666-5247(20)30003-3
92. van Doremalen N, Bushmaker T, Morris DH, Holbrook MG, Gamble A, Williamson BN, et al. Aerosol and surface stability of SARS-CoV-2 as compared with SARS-CoV-1. N Engl J Med. (2020) 382:1564–7. doi: 10.1056/NEJMc2004973
93. Coakley R, Taggart C, Greene C, McElvaney N, O'Neill S. Ambient pCO2 modulates intracellular pH, intracellular oxidant generation, and interleukin-8 secretion in human neutrophils. J Leukocyte Biol. (2002) 71:603–10. doi: 10.1189/jlb.71.4.603
94. Vadász I, Hubmayr RD, Nin N, Sporn PH, Sznajder JI. Hypercapnia: a nonpermissive environment for the lung. Am J Respir Cell Mol Biol. (2012) 46:417–21. doi: 10.1165/rcmb.2011-0395PS
95. Pugin J, Dunn-Siegrist I, Dufour J, Tissieres P, Charles PE, Comte R. Cyclic stretch of human lung cells induces an acidification and promotes bacterial growth. Am J Respir Cell Mol Biol. (2008) 38:362–70. doi: 10.1165/rcmb.2007-0114OC
96. Helenius IT, Krupinski T, Turnbull DW, Gruenbaum Y, Silverman N, Johnson EA. Elevated CO2 suppresses specific drosophila innate immune responses and resistance to bacterial infection. Proc Natl Acad Sci USA. (2009) 106:18710–15. doi: 10.1073/pnas.0905925106
97. Xie P, Ma W, Tang H, Liu D. Severe COVID-19: a review of recent progress with a look toward the future. Front Public Health. (2020) 8:189. doi: 10.3389/fpubh.2020.00189
Keywords: anti-COVID-19, antiviral, anti-cytokine storm, improve COVID-19 symptoms, carrier gas composition, enhancer antiviral, protect and improve organs function, suppression COVID-19 pandemic
Citation: El-Betany AMM, Behiry EM, Gumbleton M and Harding KG (2020) Humidified Warmed CO2 Treatment Therapy Strategies Can Save Lives With Mitigation and Suppression of SARS-CoV-2 Infection: An Evidence Review. Front. Med. 7:594295. doi: 10.3389/fmed.2020.594295
Received: 13 August 2020; Accepted: 25 November 2020;
Published: 11 December 2020.
Edited by:
Marco Iosa, Santa Lucia Foundation (IRCCS), ItalyReviewed by:
Barry Ninham, Australian National University, AustraliaRichard Pashley, University of New South Wales Canberra, Australia
Copyright © 2020 El-Betany, Behiry, Gumbleton and Harding. This is an open-access article distributed under the terms of the Creative Commons Attribution License (CC BY). The use, distribution or reproduction in other forums is permitted, provided the original author(s) and the copyright owner(s) are credited and that the original publication in this journal is cited, in accordance with accepted academic practice. No use, distribution or reproduction is permitted which does not comply with these terms.
*Correspondence: Alaa M. M. El-Betany, ZWxiZXRhbnlhMUBjYXJkaWZmLmFjLnVr; Mark Gumbleton, Z3VtYmxldG9uQGNhcmRpZmYuYWMudWs=; Keith G. Harding, aGFyZGluZ2tnQGNhcmRpZmYuYWMudWs=