- 1Laboratory for Critical Care Physiology, Feinstein Institutes for Medical Research, Northwell Health System, Manhasset, NY, United States
- 2Department of Emergency Medicine, North Shore University Hospital, Northwell Health System, Manhasset, NY, United States
- 3Department of Traumatology and Acute Critical Medicine, Osaka University Graduate School of Medicine, Osaka, Japan
- 4Department of Emergency Medicine, Donald and Barbara Zucker School of Medicine at Hofstra/Northwell, Manhasset, NY, United States
- 5Department of Surgery, Donald and Barbara Zucker School of Medicine at Hofstra/Northwell, Manhasset, NY, United States
- 6Elmezzi Graduate School of Molecular Medicine, Manhasset, NY, United States
- 7Department of Acute Medicine and Critical Care Medical Center, Osaka National Hospital, National Hospital Organization, Osaka, Japan
Despite three decades of advancements in cardiopulmonary resuscitation (CPR) methods and post-resuscitation care, neurological prognosis remains poor among survivors of out-of-hospital cardiac arrest, and there are no reliable methods for predicting neurological outcomes in patients with cardiac arrest (CA). Adopting more effective methods of neurological monitoring may aid in improving neurological outcomes and optimizing therapeutic interventions for each patient. In the present review, we summarize the development, evolution, and potential application of near-infrared spectroscopy (NIRS) in adults with CA, highlighting the clinical relevance of NIRS brain monitoring as a predictive tool in both pre-hospital and in-hospital settings. Several clinical studies have reported an association between various NIRS oximetry measurements and CA outcomes, suggesting that NIRS monitoring can be integrated into standardized CPR protocols, which may improve outcomes among patients with CA. However, no studies have established acceptable regional cerebral oxygen saturation cut-off values for differentiating patient groups based on return of spontaneous circulation status and neurological outcomes. Furthermore, the point at which resuscitation efforts can be considered futile remains to be determined. Further large-scale randomized controlled trials are required to evaluate the impact of NIRS monitoring on survival and neurological recovery following CA.
Background
Out-of-hospital cardiac arrest (OHCA) remains a major public health challenge worldwide. The global report on OHCA has described that the estimated incidence of OHCA treated via emergency medical services (EMS) was 47.3, 40.6, 45.9, and 51.1 per 100,000 person-years in North America, Europe, Asia, and Australia, respectively (i.e., ~4 million cases each year) (1). Despite advances in treatment, such as routine application of targeted temperature management (TTM), neurological prognosis remains poor among survivors of OHCA (2), and there are no reliable methods for predicting neurological outcomes in patients with cardiac arrest (CA) and post-cardiac arrest syndrome (PCAS). International guidelines recommend a multimodal approach for determining prognosis. However, these guidelines are neither universally accepted nor universally implemented, and prognostication may be delayed up to 72 h after restoration of normothermia (3–5).
Physiologically, a prolonged “no-flow” interval during CA followed by low cerebral perfusion during resuscitative management (i.e., “low-flow” status) leads to hypoxic ischemia–reperfusion brain injury—the primary cause of disability after successful resuscitation (6). Near-infrared spectroscopy (NIRS) can be used to obtain continuous, non-invasive measurements of regional cerebral oxygen saturation (rSO2) in real time, which may aid in monitoring oxygen metabolism in the brain during this ischemia–reperfusion process. Measurements of rSO2 are considered to reflect the balance between cerebral oxygen delivery and consumption in the area of the brain located beneath the device (7). NIRS has been applied in patients with circulatory shock (8), acute brain injury (9, 10), those undergoing perioperative cardiac surgery (11, 12) or carotid endarterectomy (13–15), and during veno-arterial extracorporeal membrane oxygenation in the intensive care unit (ICU) (16–18). Several recent studies have highlighted the feasibility of NIRS for brain monitoring during cardiopulmonary resuscitation (CPR) and after the return of spontaneous circulation (ROSC) in patients with CA (19–24). There are two theoretical uses for NIRS brain monitoring and it is important to understand both. Application of NIRS monitoring may aid in predicting patient outcomes, which may in turn aid clinicians in determining whether to continue or halt resuscitation efforts based on the patient's chance of survival. Alternatively, NIRS measurements may aid in determining the most appropriate resuscitation therapies. For example, patients with low initial NIRS values may benefit from more aggressive resuscitation efforts (e.g., improved CPR, pharmacological treatment, circulatory support). Unfortunately, no previous studies have validated the use of NIRS for either of these purposes. Furthermore, the cut-off rSO2 value for predicting good vs. poor clinical outcomes in patients with CA remains to be determined. Publicly available studies have also varied with regard to the timing of NIRS (during CPR or post-ROSC), the clinical setting (prehospital, emergency department [ED], or ICU), and the types of NIRS readings analyzed (initial, mean, highest, or changes in rSO2 values over the course of CPR or the ICU stay). Thus, further studies are required to determine the predictive value of NIRS monitoring and its potential for guiding treatment strategies in patients with OHCA (25). In the present review, we discuss the development and evolution of NIRS technology, as well as the potential usefulness of rSO2 during CA and post-resuscitation care.
A Brief Review of NIRS Technology
In 1977, Jöbsis provided the first evidence that NIRS can be used to monitor tissue metabolism in vivo (26). Notably, they intended to develop an optical technique for measuring in vivo redox changes in the mitochondrial enzyme cytochrome c oxidase (27). They discovered that near-infrared light penetrates deeper into tissues due to its higher tissue transparency, enabling real-time monitoring of changes in the concentrations of light-absorbing molecules within the tissue. Given that hemoglobin chromophores are present in higher concentrations than cytochrome c oxidase, numerous studies have focused on the use of NIRS to measure levels of oxygenated, deoxygenated (or redox/“reduced”), and total hemoglobin (28). In 1985, Ferrari et al. utilized NIRS for continuous, non-invasive monitoring of the human brain (29). In 1995, Müllner et al. provided the first preliminary report regarding the use of NIRS in patients with OHCA, demonstrating that higher median rSO2 values during continuous CPR in the ED were associated with better 1-week survival (19). In 2004, Newman et al. demonstrated the feasibility of continuous, non-invasive cerebral oximetry measurements obtained using NIRS and suggested a possible role for NIRS in evaluating the adequacy of CPR methods (20).
Given the physics of light in the near-infrared spectral region (600–900 nm) within the brain, tissue absorption is mainly determined based on levels of oxygenated and reduced hemoglobin, with smaller contributions from water, lipids, and cytochrome c oxidase. Cerebral saturation is measured using a light source fixed to the head, which transmits infrared and red spectrum light through the skin, skull, connective tissues, and brain. Quantification is then performed using a light detector. The separation between the source and detector is an important parameter of the NIRS system, as it determines the depth of penetration (i.e., ~2–2.5 cm with current systems) (30, 31). Values are thus measured from a “banana-shaped” volume of tissue (32, 33). Furthermore, the intence of near-infrared light is also important because the larger source-detector distance, the deeper the photon reaches inside the brain layer, but the intensity of the detected light decreases more strongly (34, 35).
rSO2 values can be affected by various factors, such as extracranial contamination, skin pigmentation (36, 37), and physiological conditions. Changes in physiological conditions may in turn lead to changes in cerebral blood flow or oxygen content. Among the factors known to influence these parameters are cardiac output, acid–base status, major hemorrhage, obstructions of arterial inflow/venous outflow, hemoglobin concentration, hemoglobin saturation, pulmonary function, inspired oxygen concentration, and drug use (e.g., phenylephrine) (38–41).
Currently, there are several commercially available NIRS devices (12, 16, 34, 36, 42–45). These devices differ with regard to the wavelengths and frequencies used, the timing of light transmission, the distance between the light source and detector, and the primary principle of measurement [e.g., Beer-Lambert law (46), spatial-resolved spectroscopy law (47), or time-resolved absorption spectroscopy law (46)]. Thus, the algorithms used to derive hemoglobin saturation from the inputs received also differ for each device. It has been reported that values for rSO2 typically range from 55 to 80%, and rSO2 <50% or a 20% reduction from the individual baseline is generally considered indicative of the need for intervention (48). However, it is noted that the threshold of the normal range is not clearly defined due to the characteristics of the equipment, and the range of normal values actually varies among the equipment (37). As most clinical NIRS devices assume a venous/arterial distribution in cerebral cortical tissue of ~70/30 or 75/25%, based in part on the results of positron emission tomography studies (39), rSO2 values are primarily influenced by cerebral venous oxygen saturation (49). However, previous studies have reported that the venous/arterial distribution of the cerebral cortex varies among individuals (37, 50, 51), suggesting that rSO2 values are also variable (50). Previous studies have reported that the absolute values, or different degrees of variability in rSO2 due to several factors, vary between NIRS devices under various conditions (36, 37, 45, 52). Given that rSO2 values also vary based on physiological conditions, some authors have suggested that relative changes in rSO2 from baseline are more appropriate for guiding resuscitative efforts than absolute values (53, 54).
Search Strategy
To review articles regarding NIRS brain monitoring in patients with CA, we searched PubMed, Web of Science, and Google Scholar for relevant studies. There was no language restriction. We developed a search strategy using the combination of keywords and Medical Subject Heading (MeSH) terms, which were “(Near-infrared spectroscopy [MeSH] OR (regional cerebral oxygen saturation) OR (brain oximetry)) AND ((Heart arrest [MeSH]) OR (cardiac surgery) OR prehospital)” for PubMed and Web of Science, and [“Near-infrared spectroscopy,” “cardiac arrest,” “regional saturation”] for Google Scholar. The main findings of the included studies are summarized in Table 1.
Use of NIRS for Earlier Detection Of Re-Arrest in Prehospital Settings
While ROSC is often successful in pre-hospital settings, many patients subsequently develop circulatory instability and experience re-arrest (i.e., a loss of pulse after sustained ROSC) (74). Since re-arrest before reaching the hospital is among the potential barriers to survival in patients with OHCA (74), early recognition of re-arrest is crucial for ensuring prompt re-activation of resuscitation protocols, including CPR and early defibrillation. Many EMS systems routinely use pulse oximetry measurements; however, pulse oximetry depends on the presence of a peripheral pulse, and the technique is unreliable when used during CA because pulsatile blood flow is inadequate in peripheral tissue beds under such conditions (75, 76). Using a finger pulse oximeter is problematic during CA because any resultant values likely reflect the pulsation of venous blood. Thus, although the presence of a plethysmograph waveform on pulse oximetry is potentially valuable in detecting ROSC, the main purpose of pulse oximetry is to ensure appropriate oxygenation after ROSC, and its use is limited during CPR (76).
In contrast to pulse oximetry, NIRS can measure tissue oxygenation in the absence of pulsatile flow, without the need to interrupt chest compressions (55, 57, 62). Since NIRS values are affected by ambient light (52, 77), some devices cannot be used outside. Nonetheless, rSO2 monitoring may aid in the early detection of ROSC and re-arrest in patients with CA (55–57, 62). Meex et al. observed that rSO2 values immediately increased after ROSC and that new episodes of ventricular fibrillation were immediately detected as sudden decreases in rSO2. These findings suggest that decline in rSO2 values can reflect life-threatening situations such as pulseless arrhythmia or severe cerebral hypoperfusion, both of which indicate an urgent need for CPR (55). Additional studies have reported that ROSC is associated with increases in NIRS values, while re-arrest is associated with decreases in NIRS values (56, 57). Notably, these studies showed the decrease in rSO2 at the re-arrest episode, which is difficult to find re-arrest without pulse check. It may be useful to be aware of re-arrest immediately without pulse check. Another study reported that NIRS monitoring can aid in assessing perfusion and guiding interventions during transport (78). Some authors have suggested that low NIRS readings highlight the need for additional lifesaving interventions such as fluid resuscitation and/or vasopressors (40, 41, 79). Therefore, NIRS monitoring may enable early recognition of re-arrest, especially in PEA, and poor cerebral circulation during EMS resuscitation protocol. Since vital signs and the results of physical assessments can be influenced by environmental factors (e.g., pre-hospital settings, ambulance transport), further clinical studies are required to determine the value of NIRS in various settings.
Feasibility of NIRS for the Assessment of CPR Quality
Well-performed CPR has been associated with higher rates of ROSC (80, 81), better cerebral perfusion (82), and improved cerebral oxygenation (83). Several large-scale studies have demonstrated that high-quality CPR improves survival and neurological outcomes among patients with CA (84–86). However, monitoring the adequacy of circulation and cerebral oxygenation during CPR remains challenging. To date, studies investigating the use of NIRS devices to assess the quality of CPR have yielded conflicting results (55, 58, 59).
To assess the quality of CPR, Kämäräinen et al. measured rSO2 using an INVOS 5100c device and simultaneously monitored indicators of CPR quality. Compression depth, the rate and release of compressions, and ventilation rate were monitored during CPR with automated real-time audiovisual feedback (59). Data related to the quality of CPR and rSO2 were measured at 30-s intervals until ROSC (59). The authors observed that cerebral oxygenation remained low throughout high-quality CPR (59), in contrast to the previous findings that cerebral rSO2 decreases due to circulatory arrest during cardiac surgery but increases during CPR (87) or cardiopulmonary bypass (88). However, the rSO2 data recorded in this study were unreliable in many cases, as 59% of the 30-s intervals exhibited artifacts that precluded quantification of rSO2 (59). In contrast, Meex et al. observed parallel increases in systolic arterial pressure and rSO2 during CPR (55), suggesting a positive effect of CPR on these two parameters. In addition, switching CPR providers resulted in a measurable increase in cerebral oxygen saturation. An rSO2 decreased to values between 30 and 35% after cessation of CPR. The authors further stated that rSO2 monitoring allows for both the continuous estimation of cerebral oxygenation without ROSC and the assessment of CPR efficacy (55). Previous research has indicated that mechanical chest compression, which is thought to provide adequate compression over long periods of time without fatigue or interruption, significantly increases rSO2 values in patients with OHCA, in contrast to manual chest compression (61). Although their sample sizes were small, other studies have also noted that mechanical CPR is associated with significantly higher rSO2 values than manual CPR (56, 60).
The abovementioned findings indicate that dynamic rSO2 monitoring may be more useful than static assessments of rSO2 during CPR, as such monitoring can provide quantitative information regarding cardiac output and cerebral perfusion during chest compressions. Application of NIRS for the assessment of CPR quality and oxygen delivery to the brain may thus help to improve clinical outcomes following CA. Further studies are required to determine how NIRS monitoring can be integrated into standardized CPR protocols.
Prediction of ROSC and Favorable Neurological Outcomes
International guidelines recommend end-tidal CO2 (ETCO2) monitoring for the assessment of CPR quality, noting that a sudden increase in ETCO2 is likely to represent an early indicator of ROSC (89). The potential value of ETCO2 for optimizing resuscitation efforts is discussed elsewhere (89). However, ETCO2 readings are influenced by mechanical ventilation settings, the tidal volume of ventilation, many drugs administered during resuscitation, and by different lung pathologies. In addition, ETCO2 monitoring does not provide data related to cerebral circulation. Thus, ETCO2 monitoring is distinctly different from NIRS monitoring. In a recent prospective study by Engle et al., ETCO2 assessments and cerebral oximetry were performed simultaneously during CPR in the ED (90). The authors observed that both ETCO2 and rSO2 were good predictors of ROSC. However, logistic regression analysis of the simultaneously collected data revealed that rSO2 was superior to ETCO2 in predicting ROSC (90).
A 2015 systematic review and meta-analysis reported that both initial and average rSO2 values were significantly higher in the ROSC group than in the non-ROSC group (63). An extensive 2016 meta-analysis including 20 studies demonstrated that mean NIRS values were higher in patients experiencing ROSC, surviving to discharge, and surviving with good neurologic outcomes than in their respective counterparts (53). The authors further reported that combined initial and mean NIRS values were higher in patients who survived to discharge and in those who experienced good neurological outcomes than in their counterparts (53). In the most recent systematic review and meta-analysis of 26 studies, Schnaubelt et al. demonstrated that both mean rSO2 and ΔrSO2 (i.e., the difference between the initial value and the value at ROSC, or the difference between the initial value and the value at the end of CPR) were higher in the ROSC group than in the non-ROSC group (64). ROSC was not observed when mean rSO2 remained <26%. An rSO2 threshold of 36% predicted ROSC with a sensitivity of 67% and specificity of 69%, while a ΔrSO2 of 7% predicted ROSC with a sensitivity of 100% and a specificity of 86% [area under the curve (AUC) = 0.733 and 0.893, respectively] (64).
However, given that baseline values vary among patients (54), comparisons of static values obtained using different devices may be methodologically problematic (91, 92). Importantly, all studies in these meta-analyses focused on averages obtained from static values, rather than on changes in NIRS readings within the same patient. Thus, it is difficult to determine the absolute cut-off value for discontinuing CPR based on the currently available data, as some patients experienced ROSC even with rSO2 values lower than the suggested cut-off values. Furthermore, some authors have suggested that dynamic assessments of rSO2 obtained throughout resuscitation are more appropriate than static assessments for evaluating outcomes in patients with CA (22). In a single-center retrospective study, Takegawa et al. evaluated the association between the probability of ROSC and the degree of rSO2 increase during CPR among 90 patients with OHCA, 35 (38.9%) of whom achieved ROSC (65). Receiver operating characteristic curve (ROC) analysis revealed that the amount of maximum rise in rSO2 value (i.e., the difference between maximum and baseline values) over a 16-min measurement period yielded an AUC of 0.75 for differentiating between the ROSC and non-ROSC groups. In addition, the best AUC value was achieved by the combination of the amount of maximum rise and baseline rSO2, rather than by the amount of maximum rise alone (AUC = 0.91) (65). The authors suggested that discontinuation of CPR may be indicated in patients with low initial values who do not exhibit an appropriate increase in rSO2, resulting in a low mean value. Taken together, the available data suggest that average rSO2 and ΔrSO2 values during CPR may aid in determining the likelihood of achieving ROSC in patients with CA. Given that it is difficult to measure mean rSO2 during on-going CPR in real-world settings, it is reasonable to focus on the combination of baseline rSO2 and ΔrSO2 during CPR. Further large-scale, prospective, multicenter studies are required to assess the ability of ΔrSO2 to predict ROSC.
Previous studies have reported good neurologic outcomes following CA in patients with both high initial rSO2 values and high mean rSO2 values (53). In the most recent meta-analysis, the calculated averaged mean rSO2 values were higher in patients with favorable neurological outcomes (Glasgow–Pittsburgh Cerebral Performance Category [CPC]: 1 or 2) than in those with poor neurological outcomes (rSO2: 47 vs. 38%, P = 0.018) (64). CPC scores of 1 or 2 were not observed in patients with mean rSO2 values ≤ 30 ± 17%. Mean rSO2 values in patients with favorable neurological outcomes were significantly above 30%. However, ROC analysis for neurological outcomes could not confirm a significant discriminatory power for mean rSO2 values (AUC = 0.770, P = 0.098), likely due to the small sample size (64). The authors concluded that mean rSO2 and ΔrSO2 values have good predictive value for ROSC but not for favorable neurological outcomes (64). Moreover, in a post hoc analysis of a randomized clinical trial, Jakkula et al. observed no association between cerebral rSO2 (median rSO2 during the first 36 h) and concentrations of neuron-specific enolase (a marker of neurological injury) at 24, 48, and 72 h after OHCA or good neurological outcomes at 6 months (72). Despite the promising trends suggested by the available evidence, clear cut-off values of rSO2 for predicting favorable outcomes after CA are yet to be established.
Use of NIRS During TTM
Given that induction of hypothermia affects cerebral oxygen metabolism and changes the balance between oxygen supply and demand (93), several studies have examined the role of NIRS monitoring during TTM (66–71, 73). Although some small-scale studies have applied NIRS monitoring during and after TTM in patients with PCAS, meta-analyses or systematic reviews on NIRS monitoring during TTM have been extremely limited. Meex et al. evaluated serial changes in rSO2 during TTM in 28 patients with OHCA who underwent hypothermia at 33°C for 24 h after ROSC (66). Values for rSO2 decreased significantly within 3 h after the onset of TTM, indicating that the balance between oxygen supply and demand may have been adversely affected. After 3 h, rSO2 gradually increased again even during hypothermia, increasing further during the 12-h rewarming period. Although there was no significant difference in rSO2 between the survival and non-survival groups, the decrease in rSO2 observed during the early stages of hypothermia was significantly greater in the non-survival group than in the survival group (66). Other studies have also reported a general trend that rSO2 values decrease after the onset of hypothermia, increasing during and after rewarming (68, 70). These results were contrary to the expectation that rSO2 values should increase due to reductions in brain metabolism/oxygen consumption and the effects of hypothermic conditions on the affinity of hemoglobin for oxygen (66). Therefore, the contrary results were likely due to increases in cerebrovascular resistance and decreases in cerebral blood flow.
There are several possible explanations for decreases in rSO2 during the early phase of TTM. Some investigators have suggested that cerebral blood flow and rSO2 are influenced by cardiac output, use of α-adrenergic vasoconstrictor agents (40, 41, 94), use of anesthetic agents, or other confounding factors. Some studies have also reported that rSO2 values during TTM are associated with neurological prognosis (67, 68, 71). Storm et al. evaluated the association between rSO2 values and neurological outcomes at hospital discharge and 6 months later in 60 patients with in-hospital cardiac arrest and OHCA. Continuous measurements of cerebral rSO2 were obtained for 40 h (i.e., from the onset of hypothermia to rewarming). Median rSO2 values at all time points (i.e., at the start of measurement; upon reaching 33°C; and at 4, 12, 24, and 40 h) were persistently higher in patients with CPC scores of 1–2 than in patients with CPC scores of 3–5 (median rSO2: 68 vs. 58%, P < 0.01) (67). However, rSO2 levels largely overlapped between outcome groups, suggesting that the potential of rSO2 to aid in predicting outcomes is limited (67). Genbrugge et al. reported that the mean rSO2 value during rewarming following hypothermia was significantly higher among patients with CPC scores of 1–2 than among those with CPC scores of 3–5 (70 ± 1 vs. 68 ± 1%, P = 0.046) (68). However, they also mentioned that significant differences in rSO2 in their study were unlikely to be clinically meaningful given that such data are not available at the bedside. Moreover, given the small sample size of the study, their data cannot be used to determine cutoff rSO2 values for predicting outcomes (68). In contrast, other studies have reported no significant differences in rSO2 values between prognosis groups, even when changes in rSO2 values over time were investigated (69, 70, 73).
Given the available evidence, further studies are required to validate the efficacy of rSO2 values during the early stages of TTM in predicting outcomes in patients with PCAS. Stratifying patients according to severity based on rSO2 values (71) may aid in distinguishing which patients would benefit from hypothermia. Further large-scale, prospective, multicenter studies are required to elucidate the potential of rSO2 during TTM for predicting neurological outcomes following CA.
Conclusion
In the present review, we summarized the development, evolution, and potential application of near-infrared spectroscopy (NIRS) in adults with CA, highlighting the clinical relevance of NIRS brain monitoring as a predictive tool in both pre-hospital and in-hospital settings (Figure 1). To date, no studies have established acceptable rSO2 cut-off values for differentiating patient groups based on ROSC status and neurological outcome. Furthermore, the extent of decrease in rSO2 from baseline that constitutes an abnormal finding in patients with CA remains to be determined. Additional studies are required to determine the point at which resuscitation efforts can be considered futile. Nonetheless, the available evidence indicates that rSO2 may aid not only in predicting outcomes among patients with CA, but also in optimizing CPR strategies and guiding neuroprotective interventions. Further large-scale randomized controlled trials are required to evaluate the impact of NIRS monitoring on survival and neurologic recovery. Moreover, additional studies should evaluate NIRS-guided resuscitative strategies, using improvements in NIRS values to optimize resuscitation efforts, post-resuscitation care, and patient outcomes.
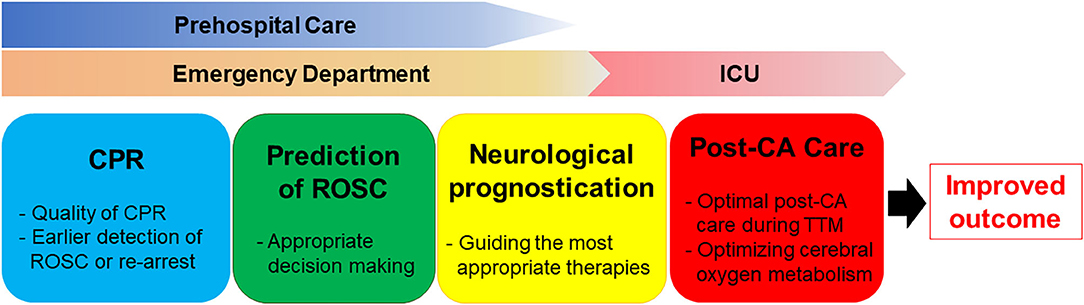
Figure 1. The clinical relevance of NIRS brain monitoring as a predictive tool in both pre-hospital and in-hospital settings.
Author Contributions
RT and KH: concept, design, and drafting manuscript. DR, TL, SM, MO, TS, and LB: critical revision of the manuscript for important intellectual content. All authors: have read and approved the manuscript.
Conflict of Interest
The authors declare that the research was conducted in the absence of any commercial or financial relationships that could be construed as a potential conflict of interest.
Supplementary Material
The Supplementary Material for this article can be found online at: https://www.frontiersin.org/articles/10.3389/fmed.2020.587930/full#supplementary-material
Abbreviations
OHCA, out-of-hospital cardiac arrest; EMS, emergency medical services; TTM, targeted temperature management; PCAS, post-cardiac arrest syndrome; rSO2, regional cerebral oxygen saturation; NIRS, near-infrared spectroscopy; ICU, intensive care unit; CPR, cardiopulmonary resuscitation; ROSC, return of spontaneous circulation; ED, emergency department; ETCO2, end-tidal CO2; AUC, area under the curve; ROC, receiver operating characteristic curve; CPC, Glasgow–Pittsburgh Cerebral Performance Category.
References
1. Berdowski J, Berg RA, Tijssen JG, Koster RW. Global incidences of out-of-hospital cardiac arrest and survival rates: systematic review of 67 prospective studies. Resuscitation. (2010) 81:1479–87. doi: 10.1016/j.resuscitation.2010.08.006
2. Soar J, Donnino MW, Maconochie I, Aickin R, Atkins DL, Andersen LW, et al. 2019 International consensus on cardiopulmonary resuscitation and emergency cardiovascular care science with treatment recommendations summary. Circulation. (2018) 138:e714–e30. doi: 10.1161/CIR.0000000000000734
3. Callaway CW, Donnino MW, Fink EL, Geocadin RG, Golan E, Kern KB, et al. Part 8: post-cardiac arrest care: 2015. American heart association guidelines update for cardiopulmonary resuscitation and emergency cardiovascular care. Circulation. (2015) 132(18 Suppl 2):S465–82. doi: 10.1161/CIR.0000000000000262
4. Nolan JP, Soar J, Cariou A, Cronberg T, Moulaert VR, Deakin CD, et al. European resuscitation council and European society of intensive care medicine guidelines for post-resuscitation care 2015: section 5 of the European resuscitation council guidelines for resuscitation 2015. Resuscitation. (2015) 95:202–22. doi: 10.1016/j.resuscitation.2015.07.018
5. Sandroni C, Cariou A, Cavallaro F, Cronberg T, Friberg H, Hoedemaekers C, et al. Prognostication in comatose survivors of cardiac arrest: an advisory statement from the European resuscitation council and the European society of intensive care medicine. Resuscitation. (2014) 85:1779–89. doi: 10.1016/j.resuscitation.2014.08.011
6. Sekhon MS, Ainslie PN, Griesdale DE. Clinical pathophysiology of hypoxic ischemic brain injury after cardiac arrest: a “two-hit” model. Crit Care. (2017) 21:90. doi: 10.1186/s13054-017-1670-9
7. Yao FS, Tseng CC, Ho CY, Levin SK, Illner P. Cerebral oxygen desaturation is associated with early postoperative neuropsychological dysfunction in patients undergoing cardiac surgery. J Cardiothorac Vasc Anesth. (2004) 18:552–8. doi: 10.1053/j.jvca.2004.07.007
8. Varis E, Pettila V, Wilkman E. Near-infrared spectroscopy in adult circulatory shock: a systematic review. J Intensive Care Med. (2020) 35:943–62. doi: 10.1177/0885066620907307
9. Rivera-Lara L, Geocadin R, Zorrilla-Vaca A, Healy R, Radzik BR, Palmisano C, et al. Validation of near-infrared spectroscopy for monitoring cerebral autoregulation in comatose patients. Neurocrit Care. (2017) 27:362–9. doi: 10.1007/s12028-017-0421-8
10. Rivera-Lara L, Geocadin R, Zorrilla-Vaca A, Healy R, Radzik BR, Palmisano C, et al. Near-infrared spectroscopy-derived cerebral autoregulation indices independently predict clinical outcome in acutely Ill comatose patients. J Neurosurg Anesthesiol. (2020) 32:234–41. doi: 10.1097/ANA.0000000000000589
11. Deschamps A, Hall R, Grocott H, Mazer CD, Choi PT, Turgeon AF, et al. Cerebral oximetry monitoring to maintain normal cerebral oxygen saturation during high-risk cardiac surgery: a randomized controlled feasibility trial. Anesthesiology. (2016) 124:826–36. doi: 10.1097/ALN.0000000000001029
12. Zheng F, Sheinberg R, Yee MS, Ono M, Zheng Y, Hogue CW. Cerebral near-infrared spectroscopy monitoring and neurologic outcomes in adult cardiac surgery patients: a systematic review. Anesth Analg. (2013) 116:663–76. doi: 10.1213/ANE.0b013e318277a255
13. Kondov S, Beyersdorf F, Schollhorn J, Benk C, Rylski B, Czerny M, et al. Outcome of near-infrared spectroscopy-guided selective shunting during carotid endarterectomy in general anesthesia. Ann Vasc Surg. (2019) 61:170–7. doi: 10.1016/j.avsg.2019.03.021
14. Greene NH, Minhaj MM, Zaky AF, Rozet I. Perioperative management of carotid endarterectomy: a survey of clinicians' backgrounds and practices. J Cardiothorac Vasc Anesth. (2014) 28:990–3. doi: 10.1053/j.jvca.2013.11.007
15. Cheng MA, Theard MA, Tempelhoff R. Anesthesia for carotid endarterectomy: a survey. J Neurosurg Anesthesiol. (1997) 9:211–16. doi: 10.1097/00008506-199707000-00002
16. Yagi T, Kawamorita T, Kuronuma K, Tachibana E, Watanabe K, Chiba N, et al. Usefulness of a new device to monitor cerebral blood oxygenation using NIRS during cardiopulmonary resuscitation in patients with cardiac arrest: a pilot study. Adv Exp Med Biol. (2020) 1232:323–9. doi: 10.1007/978-3-030-34461-0_41
17. Khan I, Rehan M, Parikh G, Zammit C, Badjatia N, Herr D, et al. Regional cerebral oximetry as an indicator of acute brain injury in adults undergoing veno-arterial extracorporeal membrane oxygenation-a prospective pilot study. Front Neurol. (2018) 9:993. doi: 10.3389/fneur.2018.00993
18. Maldonado Y, Singh S, Taylor MA. Cerebral near-infrared spectroscopy in perioperative management of left ventricular assist device and extracorporeal membrane oxygenation patients. Curr Opin Anaesthesiol. (2014) 27:81–88. doi: 10.1097/ACO.0000000000000035
19. Mullner M, Sterz F, Binder M, Hirschl MM, Janata K, Laggner AN. Near infrared spectroscopy during and after cardiac arrest–preliminary results. Clin Intensive Care. (1995) 6:107–11.
20. Newman DH, Callaway CW, Greenwald IB, Freed J. Cerebral oximetry in out-of-hospital cardiac arrest: standard CPR rarely provides detectable hemoglobin-oxygen saturation to the frontal cortex. Resuscitation. (2004) 63:189–94. doi: 10.1016/j.resuscitation.2004.05.003
21. Ito N, Nanto S, Nagao K, Hatanaka T, Kai T. Regional cerebral oxygen saturation predicts poor neurological outcome in patients with out-of-hospital cardiac arrest. Resuscitation. (2010) 81:1736–7. doi: 10.1016/j.resuscitation.2010.08.011
22. Parnia S, Nasir A, Shah C, Patel R, Mani A, Richman P. A feasibility study evaluating the role of cerebral oximetry in predicting return of spontaneous circulation in cardiac arrest. Resuscitation. (2012) 83:982–5. doi: 10.1016/j.resuscitation.2012.01.039
23. Ahn A, Yang J, Inigo-Santiago L, Parnia S. A feasibility study of cerebral oximetry monitoring during the post-resuscitation period in comatose patients following cardiac arrest. Resuscitation. (2014) 85:522–6. doi: 10.1016/j.resuscitation.2013.12.007
24. Frisch A, Suffoletto BP, Frank R, Martin-Gill C, Menegazzi JJ. Potential utility of near-infrared spectroscopy in out-of-hospital cardiac arrest: an illustrative case series. Prehosp Emerg Care. (2012) 16:564–70. doi: 10.3109/10903127.2012.702191
25. Soar J, Callaway CW, Aibiki M, Bottiger BW, Brooks SC, Deakin CD, et al. Part 4: advanced life support: 2015 international consensus on cardiopulmonary resuscitation and emergency cardiovascular care science with treatment recommendations. Circulation. (2015) 132(16 Suppl 1):S84–145. doi: 10.1161/CIR.0000000000000273
26. Jobsis FF. Noninvasive, infrared monitoring of cerebral and myocardial oxygen sufficiency and circulatory parameters. Science. (1977) 198:1264–7. doi: 10.1126/science.929199
27. Jobsis FF, Keizer JH, LaManna JC, Rosenthal M. Reflectance spectrophotometry of cytochrome aa3 in vivo. J Appl Physiol Respir Environ Exerc Physiol. (1977) 43:858–72. doi: 10.1152/jappl.1977.43.5.858
28. Scholkmann F, Kleiser S, Metz AJ, Zimmermann R, Mata Pavia J, Wolf U, et al. A review on continuous wave functional near-infrared spectroscopy and imaging instrumentation and methodology. Neuroimage. (2014) 85:6–27. doi: 10.1016/j.neuroimage.2013.05.004
29. Ferrari M, Giannini I, Sideri G, Zanette E. Continuous non invasive monitoring of human brain by near infrared spectroscopy. Adv Exp Med Biol. (1985) 191:873–82. doi: 10.1007/978-1-4684-3291-6_88
30. Martelli F, Binzoni T, Pifferi A, Spinelli L, Farina A, Torricelli A. There's plenty of light at the bottom: statistics of photon penetration depth in random media. Sci Rep. (2016) 6:27057. doi: 10.1038/srep27057
31. Obrig H, Villringer A. Beyond the visible–imaging the human brain with light. J Cereb Blood Flow Metab. (2003) 23:1–18. doi: 10.1097/01.WCB.0000043472.45775.29
32. Feng S, Zeng FA, Chance B. Photon migration in the presence of a single defect: a perturbation analysis. Appl Opt. (1995) 34:3826–37. doi: 10.1364/AO.34.003826
33. Sassaroli A, Pifferi A, Contini D, Torricelli A, Spinelli L, Wabnitz H, et al. Forward solvers for photon migration in the presence of highly and totally absorbing objects embedded inside diffusive media. J Opt Soc Am A Opt Image Sci Vis. (2014) 31:460–9. doi: 10.1364/JOSAA.31.000460
34. Pisano A, Di Fraja D, Palmieri C. Monitoring cerebral oximetry by near-infrared spectroscopy (NIRS) in anesthesia and critical care: progress and perspectives. Gen Anesthesia Res Neuromethods. (2020) 150:75–96. doi: 10.1007/978-1-4939-9891-3_4
35. Mansouri C, L'Huillier J P, Kashou NH, Humeau A. Depth sensitivity analysis of functional near-infrared spectroscopy measurement using three-dimensional monte carlo modelling-based magnetic resonance imaging. Lasers Med Sci. (2010) 25:431–8. doi: 10.1007/s10103-010-0754-4
36. Davie SN, Grocott HP. Impact of extracranial contamination on regional cerebral oxygen saturation: a comparison of three cerebral oximetry technologies. Anesthesiology. (2012) 116:834–40. doi: 10.1097/ALN.0b013e31824c00d7
37. Bickler PE, Feiner JR, Rollins MD. Factors affecting the performance of 5 cerebral oximeters during hypoxia in healthy volunteers. Anesth Analg. (2013) 117:813–23. doi: 10.1213/ANE.0b013e318297d763
39. Ito H, Kanno I, Fukuda H. Human cerebral circulation: positron emission tomography studies. Ann Nucl Med. (2005) 19:65–74. doi: 10.1007/BF03027383
40. Nissen P, Brassard P, Jorgensen TB, Secher NH. Phenylephrine but not ephedrine reduces frontal lobe oxygenation following anesthesia-induced hypotension. Neurocrit Care. (2010) 12:17–23. doi: 10.1007/s12028-009-9313-x
41. Meng L, Cannesson M, Alexander BS, Yu Z, Kain ZN, Cerussi AE, et al. Effect of phenylephrine and ephedrine bolus treatment on cerebral oxygenation in anaesthetized patients. Br J Anaesth. (2011) 107:209–17. doi: 10.1093/bja/aer150
42. Schneider A, Minnich B, Hofstatter E, Weisser C, Hattinger-Jurgenssen E, Wald M. Comparison of four near-infrared spectroscopy devices shows that they are only suitable for monitoring cerebral oxygenation trends in preterm infants. Acta Paediatrica. (2014) 103:934–8. doi: 10.1111/apa.12698
43. Hou L, Liu Y, Qian L, Zheng Y, Gao J, Cao W, et al. Portable near-infrared technologies and devices for noninvasive assessment of tissue hemodynamics. J Healthc Eng. (2019) 2019:3750495. doi: 10.1155/2019/3750495
44. Tajima G, Shiozaki T, Izumino H, Yamano S, Hirao T, Inokuma T, et al. Portable system for monitoring of regional cerebral oxygen saturation during prehospital cardiopulmonary resuscitation: a pilot study. Acute Med Surg. (2015) 2:48–52. doi: 10.1002/ams2.71
45. Andresen B, Greisen G, Hyttel-Sorensen S. Comparison of INVOS 5100C and nonin SenSmart X-100 oximeter performance in preterm infants with spontaneous apnea. Pediatric Res. (2020) 87:1244–50. doi: 10.1038/s41390-020-0752-6
46. Pellicer A, Bravo Mdel C. Near-infrared spectroscopy: a methodology-focused review. Semin Fetal Neonatal Med. (2011) 16:42–49. doi: 10.1016/j.siny.2010.05.003
47. Liu H, Boas DA, Zhang Y, Yodh AG, Chance B. Determination of optical properties and blood oxygenation in tissue using continuous NIR light. Phys Med Biol. (1995) 40:1983–93. doi: 10.1088/0031-9155/40/11/015
48. Scheeren TW, Schober P, Schwarte LA. Monitoring tissue oxygenation by near infrared spectroscopy (NIRS): background and current applications. J Clin Monit Comput. (2012) 26:279–87. doi: 10.1007/s10877-012-9348-y
49. Murkin JM, Arango M. Near-infrared spectroscopy as an index of brain and tissue oxygenation. Br J Anaesth. (2009) 103(Suppl. 1):i3–13. doi: 10.1093/bja/aep299
50. Watzman HM, Kurth CD, Montenegro LM, Rome J, Steven JM, Nicolson SC. Arterial and venous contributions to near-infrared cerebral oximetry. Anesthesiology. (2000) 93:947–53. doi: 10.1097/00000542-200010000-00012
51. Pisano A. Can we claim accuracy from a regional near-infrared spectroscopy oximeter? Anesth Analg. (2016) 122:920. doi: 10.1213/ANE.0000000000001019
52. Zaouter C, Arbeid E. Influence of ambient light on cerebral oximeters. Br J Anaesth. (2010) 105:873–4. doi: 10.1093/bja/aeq330
53. Cournoyer A, Iseppon M, Chauny JM, Denault A, Cossette S, Notebaert E. Near-infrared spectroscopy monitoring during cardiac arrest: a systematic review and meta-analysis. Acad Emerg Med. (2016) 23:851–62. doi: 10.1111/acem.12980
54. Tobias JD. Cerebral oxygenation monitoring: near-infrared spectroscopy. Expert Rev Med Devices. (2006) 3:235–43. doi: 10.1586/17434440.3.2.235
55. Meex I, De Deyne C, Dens J, Scheyltjens S, Lathouwers K, Boer W, et al. Feasibility of absolute cerebral tissue oxygen saturation during cardiopulmonary resuscitation. Crit Care. (2013) 17:R36. doi: 10.1186/cc12546
56. Schewe JC, Thudium MO, Kappler J, Steinhagen F, Eichhorn L, Erdfelder F, et al. Monitoring of cerebral oxygen saturation during resuscitation in out-of-hospital cardiac arrest: a feasibility study in a physician staffed emergency medical system. Scand J Trauma Resusc Emerg Med. (2014) 22:58. doi: 10.1186/s13049-014-0058-y
57. Nomura J, Takegawa R, Shiozaki T, Kakino H, Murai M, Katsura K, et al. A case report: an out-of-hospital cardiac arrest (OHCA) patient with pulseless electrical activity monitored cerebral rSO2 from the prehospital scene. J Jpn Soc Emerg Med. (2016) 19:745–7. doi: 10.11240/jsem.19.745
58. Paarmann H, Heringlake M, Sier H, Schon J. The association of non-invasive cerebral and mixed venous oxygen saturation during cardiopulmonary resuscitation. Interact Cardiovasc Thorac Surg. (2010) 11:371–3. doi: 10.1510/icvts.2010.240929
59. Kämäräinen A, Sainio M, Olkkola KT, Huhtala H, Tenhunen J, Hoppu S. Quality controlled manual chest compressions and cerebral oxygenation during in-hospital cardiac arrest. Resuscitation. (2012) 83:138–42. doi: 10.1016/j.resuscitation.2011.09.011
60. Parnia S, Nasir A, Ahn A, Malik H, Yang J, Zhu J, et al. A feasibility study of cerebral oximetry during in-hospital mechanical and manual cardiopulmonary resuscitation*. Crit Care Med. (2014) 42:930–33. doi: 10.1097/CCM.0000000000000047
61. Ogawa Y, Shiozaki T, Hirose T, Ohnishi M, Nakamori Y, Ogura H, et al. Load-distributing-band cardiopulmonary resuscitation for out-of-hospital cardiac arrest increases regional cerebral oxygenation: a single-center prospective pilot study. Scand J Trauma Resusc Emerg Med. (2015) 23:99. doi: 10.1186/s13049-015-0182-3
62. Asim K, Gokhan E, Ozlem B, Ozcan Y, Deniz O, Kamil K, et al. Near infrared spectrophotometry (cerebral oximetry) in predicting the return of spontaneous circulation in out-of-hospital cardiac arrest. Am J Emerg Med. (2014) 32:14–17. doi: 10.1016/j.ajem.2013.09.010
63. Sanfilippo F, Serena G, Corredor C, Benedetto U, Maybauer MO, Al-Subaie N, et al. Cerebral oximetry and return of spontaneous circulation after cardiac arrest: a systematic review and meta-analysis. Resuscitation. (2015) 94:67–72. doi: 10.1016/j.resuscitation.2015.06.023
64. Schnaubelt S, Sulzgruber P, Menger J, Skhirtladze-Dworschak K, Sterz F, Dworschak M. Regional cerebral oxygen saturation during cardiopulmonary resuscitation as a predictor of return of spontaneous circulation and favourable neurological outcome - a review of the current literature. Resuscitation. (2018) 125:39–47. doi: 10.1016/j.resuscitation.2018.01.028
65. Takegawa R, Shiozaki T, Ogawa Y, Hirose T, Mori N, Ohnishi M, et al. Usefulness of cerebral rSO2 monitoring during CPR to predict the probability of return of spontaneous circulation. Resuscitation. (2019) 139:201–7. doi: 10.1016/j.resuscitation.2019.04.015
66. Meex I, Dens J, Jans F, Boer W, Vanhengel K, Vundelinckx G, et al. Cerebral tissue oxygen saturation during therapeutic hypothermia in post-cardiac arrest patients. Resuscitation. (2013) 84:788–93. doi: 10.1016/j.resuscitation.2013.01.003
67. Storm C, Leithner C, Krannich A, Wutzler A, Ploner CJ, Trenkmann L, et al. Regional cerebral oxygen saturation after cardiac arrest in 60 patients–a prospective outcome study. Resuscitation. (2014) 85:1037–41. doi: 10.1016/j.resuscitation.2014.04.021
68. Genbrugge C, Eertmans W, Meex I, Van Kerrebroeck M, Daems N, Creemers A, et al. What is the value of regional cerebral saturation in post-cardiac arrest patients? A prospective observational study. Crit Care. (2016) 20:327. doi: 10.1186/s13054-016-1509-9
69. Bougle A, Daviaud F, Bougouin W, Rodrigues A, Geri G, Morichau-Beauchant T, et al. Determinants and significance of cerebral oximetry after cardiac arrest: a prospective cohort study. Resuscitation. (2016) 99:1–6. doi: 10.1016/j.resuscitation.2015.11.011
70. Saritas A, Cinleti BA, Zincircioglu C, Uzun U, Kose I, Senoglu N. Effect of regional cerebral oximetry to estimate neurologic prognostic outcomes in patients administered targeted temperature management. Am J Emerg Med. (2018) 36:2236–41. doi: 10.1016/j.ajem.2018.04.016
71. Nakatani Y, Nakayama T, Nishiyama K, Takahashi Y. Effect of target temperature management at 32-34 degrees C in cardiac arrest patients considering assessment by regional cerebral oxygen saturation: A multicenter retrospective cohort study. Resuscitation. (2018) 126:185–90. doi: 10.1016/j.resuscitation.2018.02.007
72. Jakkula P, Hastbacka J, Reinikainen M, Pettila V, Loisa P, Tiainen M, et al. Near-infrared spectroscopy after out-of-hospital cardiac arrest. Crit Care. (2019) 23:171. doi: 10.1186/s13054-019-2428-3
73. Sakurai A, Ihara S, Tagami R, Yamaguchi J, Sugita A, Kuwana T, et al. Parameters influencing brain oxygen measurement by regional oxygen saturation in postcardiac arrest patients with targeted temperature management. Ther Hypothermia Temp Manag. (2020) 10:71–75. doi: 10.1089/ther.2019.0032
74. Salcido DD, Stephenson AM, Condle JP, Callaway CW, Menegazzi JJ. Incidence of rearrest after return of spontaneous circulation in out-of-hospital cardiac arrest. Prehosp Emerg Care. (2010) 14:413–18. doi: 10.3109/10903127.2010.497902
75. European Resuscitation Council. Part 6: advanced cardiovascular life support. Section 4: devices to assist circulation. European resuscitation council. Resuscitation. (2000) 46:127–34. doi: 10.1016/S0300-9572(00)00277-X
76. Neumar RW, Otto CW, Link MS, Kronick SL, Shuster M, Callaway CW, et al. Part 8: adult advanced cardiovascular life support: 2010 American heart association guidelines for cardiopulmonary resuscitation and emergency cardiovascular care. Circulation. (2010) 122(18 Suppl 3):S729–67. doi: 10.1161/CIRCULATIONAHA.110.970988
77. Wik L. Near-infrared spectroscopy during cardiopulmonary resuscitation and after restoration of spontaneous circulation: a valid technology? Curr Opin Crit Care. (2016) 22:191–8. doi: 10.1097/MCC.0000000000000301
78. Parker J, Walenta T, Turner-Nelson K. Near-infrared spectroscopy in transport with a patient in multi-factorial shock. Air Med J. (2019) 38:235–8. doi: 10.1016/j.amj.2019.03.005
79. Balakrishnan B, Dasgupta M, Gajewski K, Hoffmann RG, Simpson PM, Havens PL, et al. Low near infrared spectroscopic somatic oxygen saturation at admission is associated with need for lifesaving interventions among unplanned admissions to the pediatric intensive care unit. J Clin Monit Comput. (2018) 32:89–96. doi: 10.1007/s10877-017-0007-1
80. Abella BS, Sandbo N, Vassilatos P, Alvarado JP, O'Hearn N, Wigder HN, et al. Chest compression rates during cardiopulmonary resuscitation are suboptimal: a prospective study during in-hospital cardiac arrest. Circulation. (2005) 111:428–34. doi: 10.1161/01.CIR.0000153811.84257.59
81. Eftestol T, Sunde K, Steen PA. Effects of interrupting precordial compressions on the calculated probability of defibrillation success during out-of-hospital cardiac arrest. Circulation. (2002) 105:2270–3. doi: 10.1161/01.CIR.0000016362.42586.FE
82. Yannopoulos D, McKnite S, Aufderheide TP, Sigurdsson G, Pirrallo RG, Benditt D, et al. Effects of incomplete chest wall decompression during cardiopulmonary resuscitation on coronary and cerebral perfusion pressures in a porcine model of cardiac arrest. Resuscitation. (2005) 64:363–72. doi: 10.1016/j.resuscitation.2004.10.009
83. Imberti R, Bellinzona G, Riccardi F, Pagani M, Langer M. Cerebral perfusion pressure and cerebral tissue oxygen tension in a patient during cardiopulmonary resuscitation. Intensive Care Med. (2003) 29:1016–19. doi: 10.1007/s00134-003-1719-x
84. Stiell I, Nichol G, Wells G, De Maio V, Nesbitt L, Blackburn J, et al. Health-related quality of life is better for cardiac arrest survivors who received citizen cardiopulmonary resuscitation. Circulation. (2003) 108:1939–44. doi: 10.1161/01.CIR.0000095028.95929.B0
85. Ewy GA, Bobrow BJ. Cardiocerebral resuscitation: an approach to improving survival of patients with primary cardiac arrest. J Intensive Care Med. (2016) 31:24–33. doi: 10.1177/0885066614544450
86. Bobrow BJ, Vadeboncoeur TF, Stolz U, Silver AE, Tobin JM, Crawford SA, et al. The influence of scenario-based training and real-time audiovisual feedback on out-of-hospital cardiopulmonary resuscitation quality and survival from out-of-hospital cardiac arrest. Ann Emerg Med. (2013) 62:47–56.e41. doi: 10.1016/j.annemergmed.2012.12.020
87. Nagdyman N, Fleck TP, Ewert P, Abdul-Khaliq H, Redlin M, Lange PE. Cerebral oxygenation measured by near-infrared spectroscopy during circulatory arrest and cardiopulmonary resuscitation. Br J Anaesth. (2003) 91:438–42. doi: 10.1093/bja/aeg181
88. Pilkington SN, Hett DA, Pierce JM, Smith DC. Auditory evoked responses and near infrared spectroscopy during cardiac arrest. Br J Anaesth. (1995) 74:717–19. doi: 10.1093/bja/74.6.717
89. Link MS, Berkow LC, Kudenchuk PJ, Halperin HR, Hess EP, Moitra VK, et al. Part 7: adult advanced cardiovascular life support: 2015 American heart association guidelines update for cardiopulmonary resuscitation and emergency cardiovascular care. Circulation. (2015) 132(18 Suppl 2):S444–64. doi: 10.1161/CIR.0000000000000261
90. Engel TW, II, Thomas C, Medado P, Bastani A, Reed B, Millis S, et al. End tidal CO2 and cerebral oximetry for the prediction of return of spontaneous circulation during cardiopulmonary resuscitation. Resuscitation. (2019) 139:174–81. doi: 10.1016/j.resuscitation.2019.04.006
91. Genbrugge C, Dens J, Meex I, Boer W, Jans F, De Deyne C. Cerebral saturation monitoring during cardiopulmonary resuscitation should be used as dynamic, rather than static, information. Resuscitation. (2013) 84:e111–12. doi: 10.1016/j.resuscitation.2012.10.028
92. Thavasothy M, Broadhead M, Elwell C, Peters M, Smith M. A comparison of cerebral oxygenation as measured by the NIRO 300 and the INVOS 5100 near-infrared spectrophotometers. Anaesthesia. (2002) 57:999–1006. doi: 10.1046/j.1365-2044.2002.02826.x
93. Busija DW, Leffler CW. Hypothermia reduces cerebral metabolic rate and cerebral blood flow in newborn pigs. Am J Physiol. (1987) 253:H869–73. doi: 10.1152/ajpheart.1987.253.4.H869
Keywords: cardiac arrest, cardiopulmonary resuscitation, near-infrared spectroscopy, cerebral oxygen saturation, brain oximetry, ROSC, neurological outcome, prognostication
Citation: Takegawa R, Hayashida K, Rolston DM, Li T, Miyara SJ, Ohnishi M, Shiozaki T and Becker LB (2020) Near-Infrared Spectroscopy Assessments of Regional Cerebral Oxygen Saturation for the Prediction of Clinical Outcomes in Patients With Cardiac Arrest: A Review of Clinical Impact, Evolution, and Future Directions. Front. Med. 7:587930. doi: 10.3389/fmed.2020.587930
Received: 27 July 2020; Accepted: 02 October 2020;
Published: 29 October 2020.
Edited by:
Chih-Hsien Wang, National Taiwan University Hospital, TaiwanReviewed by:
Gyaninder Pal Singh, All India Institute of Medical Sciences, IndiaTaka-aki Nakada, Chiba University, Japan
Copyright © 2020 Takegawa, Hayashida, Rolston, Li, Miyara, Ohnishi, Shiozaki and Becker. This is an open-access article distributed under the terms of the Creative Commons Attribution License (CC BY). The use, distribution or reproduction in other forums is permitted, provided the original author(s) and the copyright owner(s) are credited and that the original publication in this journal is cited, in accordance with accepted academic practice. No use, distribution or reproduction is permitted which does not comply with these terms.
*Correspondence: Kei Hayashida, a2hheWFzaGlkYSYjeDAwMDQwO25vcnRod2VsbC5lZHU=