- Division of Nephrology, Department of Medicine, Center for Immunity, Inflammation, and Regenerative Medicine (CIIR), University of Virginia, Charlottesville, VA, United States
Acute kidney injury (AKI) is a major clinical burden affecting 20 to 50% of hospitalized and intensive care patients. Irrespective of the initiating factors, the immune system plays a major role in amplifying the disease pathogenesis with certain immune cells contributing to renal damage, whereas others offer protection and facilitate recovery. Alarmins are small molecules and proteins that include granulysins, high-mobility group box 1 protein, interleukin (IL)-1α, IL-16, IL-33, heat shock proteins, the Ca++ binding S100 proteins, adenosine triphosphate, and uric acid. Alarmins are mostly intracellular molecules, and their release to the extracellular milieu signals cellular stress or damage, generally leading to the recruitment of the cells of the immune system. Early studies indicated a pro-inflammatory role for the alarmins by contributing to immune-system dysregulation and worsening of AKI. However, recent developments demonstrate anti-inflammatory mechanisms of certain alarmins or alarmin-sensing receptors, which may participate in the prevention, resolution, and repair of AKI. This dual function of alarmins is intriguing and has confounded the role of alarmins in AKI. In this study, we review the contribution of various alarmins to the pathogenesis of AKI in experimental and clinical studies. We also analyze the approaches for the therapeutic utilization of alarmins for AKI.
Introduction
Acute kidney injury (AKI) is a global problem associated with high mortality, morbidity, and clinical burden (1). AKI is defined as an abrupt deterioration of kidney function indicated by an increase in circulating levels of creatinine and blood urea nitrogen (BUN) and a decline in urine output and glomerular filtration rate (GFR) (1). Several factors can result in AKI including ischemia/reperfusion injury (IRI), sepsis, hemodynamic changes, systemic inflammation, muscle wasting, and nephrotoxicity (2, 3). The pathophysiology of AKI is multifaceted, exhibiting inflammation, tubular injury, and vascular damage (4), and can cause damages to the brain, heart, and lungs in the long run. There is no approved drug for treating AKI patients, and current clinical care involves renal replacement therapy (RRT) (1).
With the ever-changing definitions of damage-associated molecular patterns (DAMPs) and alarmins, newer criteria were established during the International DAMP & Alarmins meeting held in Japan in November 2019 (5). “Alarmins” are a class of endogenous immunomodulatory molecules released or expressed by living cells upon cell injury, death, stress, or infection that triggers activation of the immune system (5, 6). In February 2006 in an European Molecular Biology Organization workshop on innate danger signal held in Milano, Italy, Dr. Joost Oppenheim coined the term “alarmin” to designate endogenous molecules that signal tissue and cellular damage (7). Originally proposed by Dr. Polly Matzinger, DAMPs are endogenous molecules released upon non-programmed cell death that triggers inflammatory and immune responses (8), whereas pathogen-associated molecular patterns (PAMPs) are derived from invading microbes, for example, lipopolysaccharides (LPSs) that exhibit distinct biochemical property such that they alert intrusion of the pathogens (9). The PAMPs and DAMPs were shown to trigger specific pattern recognition receptors (PRRs), for example, Toll-like receptors (TLRs) for immune activation (10, 11). Although DAMPs may now be recognized as molecules that are released or secreted from dead cells, and alarmins constitute molecules that are secreted by living cells (5), there is still a lot of overlap and ambiguity in the literature. Nevertheless, to our understanding and for the purpose of this review, all DAMPs are alarmins, but not all alarmins are DAMPs. Several types of alarmins have now been recognized and are classified as nuclear, cytosolic, mitochondrial, extracellular matrix, and secreted (granule-derived) (Table 1). Recent evidences suggest that alarmins are pleiotropic factors that promote both inflammatory and regulatory responses (6). Both alarmins and their receptors are emerging as important biomarkers in a variety of disease conditions (6). Here, we review and discuss the inflammatory, regulatory, and regenerative capabilities of alarmin as it relates to AKI (Figure 1). Based on the available literary evidence, we classify the “yin” and “yang” of alarmins (Figure 2).
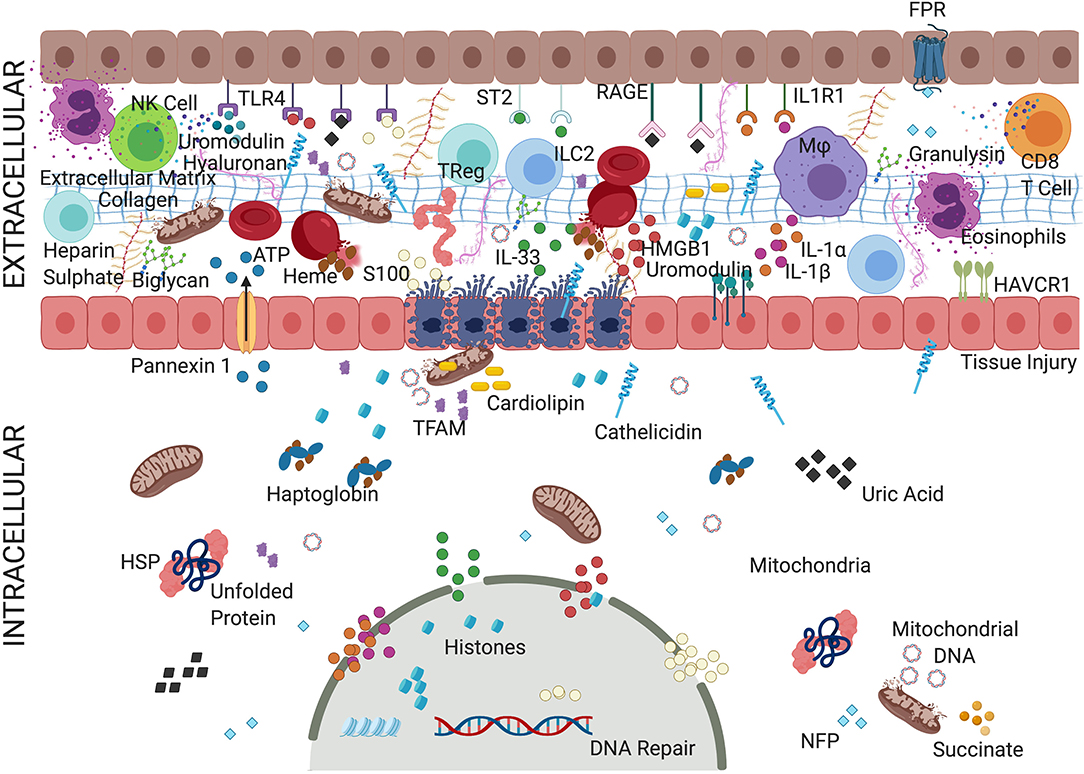
Figure 1. Convoluted mechanism of action of alarmins. An overview depicting complex but critical mechanisms of action of alarmins. During AKI, various biomolecules, termed alarmins, are triggered that include various proteins, non-protein small molecules, metabolites, and cellular organelles. Alarmins have been implicated in both pro-inflammatory activity, promoting inflammatory cells, and mounting anti-inflammatory effects and facilitating repair. The alarmins are classified as nuclear, cytosolic, cell membrane, extracellular matrix, and secreted (granule-derived). Most of the alarmins exert their pathological effects through cell surface receptors such as TLRs, IL-1Rs family, or RAGE triggering activation of various downstream targets such as NF-κB and interferon responsive factors (IRFs). Adenosine triphosphate (ATP), cathelicidins, defensins act through ionotropic, metabolic, and purinergic receptors, which facilitate the organization of NLRP3 inflammasome complex. Alarmins such as heparin sulfate (HS) and insulin-like growth factor–binding protein 7 (IGFBP7) binds to growth factor receptors activating immunomodulatory and prosurvival signals. Fragmented mitochondria released from the damaged cells could trigger inflammatory milieu. Thus, various alarmins activated during cellular injury not only induce inflammatory cells but act as a source of biomarkers and recruit regulatory cells to resolve the inflammation and initiate tissue repair. The specific role of alarmins in tissue injury, inflammation, and repair is underexplored but slowly evolving. For details refer to manuscript text. TLRs, Toll-like receptors; IL-1R, interleukin 1 receptor; IL-1RL1, interleukin-1 receptor-like 1 receptor; RAGE, receptor for advanced glycation end products; interleukin 33 (IL-33); interleukin 1α/β (IL-1α/β); HMGB1, high mobility group box 1; HAVcr-1, hepatitis A virus cellular receptor 1; HSPs, heat shock proteins; NFP, N-formyl peptides.
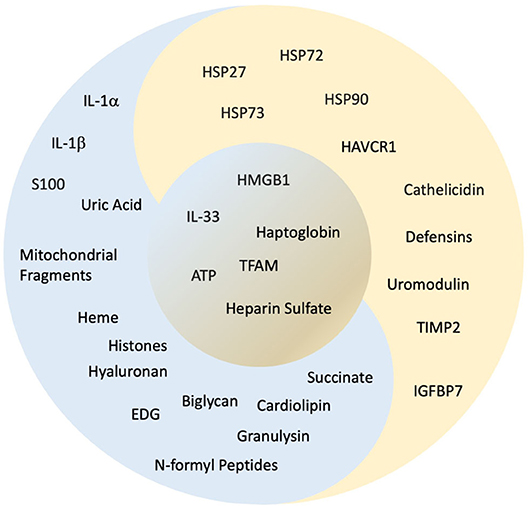
Figure 2. “Yin and yang” classification of alarmins. The concept of yin and yang is dualism. It shows how apparently opposing or contrary powers can really be similar, intertwined, and interdependent in the natural universe and how they can give rise to each other as they are engaged during AKI. Here, based on the available evidence, we have classified the alarmins, which have a negative influence as “yin” as represented in “blue,” alarmins with positive influence as “yang” represented in “gold” and alarmins with both “yin”/“yang” qualities are placed in the center represented in contrast between “blue” and “gold.” Refer to Figure 1 and Table 1 for abbreviations and the text for details.
Pro-Inflammatory Role of Alarmins in AKI
Nuclear Alarmins
IL-1 family cytokines consisting of IL-1α, IL-1β, IL-18, IL-33, IL-36α, IL-36β, IL-36γ, IL-36Rα, IL-37, IL-38, and IL1Ra are nuclear proteins that are produced as pro-proteins and are matured by proteases (82). Interleukin 1α and IL-1β promote pro-inflammatory cytokine production by multiple immune cells in toxin-induced AKI (83). Interleukin 1α-deficient mice were protected from cisplatin-induced AKI (15). However, there was no difference in inflammatory cell infiltration between wild-type and IL-1α−/− mice. The IL-1 family cytokine IL-33 has emerged as a critical factor in controlling the type 1 cytokine production. IL-33 is a nuclear protein that is typically released from the damaged cell and promotes inflammatory response (84). Increased expression of IL-33 was observed in kidneys of cisplatin and IRI-induced AKI models (17, 18). In the IRI model, IL-33 was postulated to amplify the recruitment of myeloid cells through secretion of chemokines monocyte chemoattractant protein 1 (MCP-1) and macrophage inflammatory protein 2 by the epithelial cells early after injury and promoted activation of invariant natural killer (NK) T cells in later stages (18). Following renal transplantation in patients, increased levels of IL-33 were observed in serum and urine and may contribute to renal IRI (19).
High mobility group box 1 (HMGB1) is a nuclear protein that acts as a cotranscription factor and plays an important role in DNA repair, differentiation, and development (12). Upon release from the damaged cells, HMGB1 plays an active role in pro-inflammatory responses. HMGB1 exerts its pathogenic effects on kidneys through receptor for advanced glycation end products (RAGE) and TLRs including TLR2/TLR4/TLR5/TLR9 (12, 85). A cross-section clinical study demonstrated a rise in serum HMGB1 levels in patients with AKI (14). In experimental studies too, the administration of rHMGB1 after IRI exacerbated injury (13). Sepsis-induced AKI in mice with chronic kidney disease (CKD) increased the expression of vascular endothelial growth factor (VEGF) and HMGB1 levels; however, inhibition of HMGB1, but not VEGF, was found to be protective (86).
Mice with a deficiency in TLR4, one of the receptors for HMGB1, were protected against kidney IRI. Moreover, neither the anti-HMGB1 antibody nor rHMGB1 administration affected the renoprotection in TLR4−/− mice (13). The results indicate that HMGB1 might promote kidney injury through TLR4 signaling. Glycyrrhizic acid could also attenuate renal IRI by inhibiting the interactions of HMGB1 with tubular epithelial cells (TECs) (87). Treatment with mycophenolate mofetil (MMF), a commonly used immunosuppressant, resulted in the improvement of renal function in IRI along with reduced levels of plasma creatinine and cytokines, as well as lower TLR4 expression (88). However, there was no change in HMGB1 levels, thus implying that MMF reduces TLR4 expression directly. Interestingly, pretreatment with carbon monoxide-releasing molecule-2 prevented the nuclear histone acetyltransferase activity by inhibiting HMGB1 release (89). This resulted in a reduction in the pathological damage to the kidney and was accompanied by downregulation of TLR4, RAGE, tumor necrosis factor α (TNF-α), IL-1β, IL-6, and MCP-1 and protection from IRI, indicating HMGB1 as one of the mechanisms of MMF treatment. Elevated levels of circulating HMGB1 were found in patients with AKI (14) and were independently associated with leukocyte count and correlated negatively with proteinuria in AKI settings.
Histones are highly basic proteins, rich in arginine and lysine, and highly conserved across species. They provide structural stability to chromatin and regulate gene expression (90). Histones in extracellular space may appear either due to release from damaged cells, by pro-inflammatory cells through active secretion, or as a component of neutrophil extracellular traps from infiltrating neutrophils (91). Extracellular histones released from dying tubular cells were associated with AKI, and were found not only to exhibit direct toxicity to renal cells but to induce pro-inflammatory cytokine and activate the innate immune response in a TLR2/TLR4-dependent manner (20).
Cytosolic Alarmins
Heat shock proteins (HSPs) play an important role in a variety of cellular processes such as cryoprotection, intracellular assembly, protein folding, and translocation of oligomeric proteins (23). AKI increases the expression of HSP27, HSP72, and HSP73 in kidney tissues (21, 92–94). HSP27, HSP72, and HSP73 prevent apoptosis by decreasing intracellular reactive oxygen species (ROS) and by targeting mitochondrial caspase-dependent apoptotic pathways (92, 93, 95). They may also help with the stabilization and refolding of aggregated cellular proteins in an adenosine triphosphate (ATP)–dependent fashion (93). HSP90, on the other hand, participates in regeneration and differentiation of injured tubules (96). In a clinical study, the urinary level of HSP72 did not increase significantly in kidney transplant recipients with prerenal AKI, and a small increase in HSP70 level was noted at patients with other factors of AKI, namely, obstructive uropathy, calcineurin inhibitor drug toxicity, recurrence of primary glomerular disease, and non-steroidal anti-inflammatory drug use (97). Additionally, in the pediatric patient group, it was shown that HSP60 could be used as a diagnostic tool for AKI secondary to septic shock (98).
S100 proteins are a family of cytosolic calcium-binding proteins of ~25 known members that are involved in controlling apoptosis, proliferation, differentiation, migration, energy metabolism, calcium balance, protein phosphorylation, and inflammation (99). S100A8 and S100A9 are secretory proteins that can form both heterodimers and homodimers. S100A8/A9 derived from neutrophils and monocytes acts as an activator of the innate immune system through TLR4 (24). Based on the observations that levels of S100A8/A9 were proportionally elevated with increasing severity of experimental kidney injury (24), their serum levels were utilized as an early prognostic marker of AKI associated with cardiac surgery in a clinical study (25).
Uric acid crystallization has long been associated with gouty arthritis and kidney stones. However, a strong correlation of serum uric acid and AKI is emerging with multiple chronic conditions including hypertension, CKD, cardiovascular diseases, stroke, diabetic nephropathy, and metabolic syndrome (26). Uric acid acts as an antioxidant in the extracellular environment but exhibits pro-oxidant activity in the intracellular environment (100). Hyperuricemia in AKI results in dilatation of the collecting ducts leading to flattening of the epithelium, and multiple downstream consequences that include intraluminal crystal precipitation, increased intraluminal hydrostatic pressures, decrease in GFR and renal plasma flow, activation of inflammasome and necroptosis, crystal adhesion, granuloma formation, interstitial inflammation, and tubular cell injury (101, 102).
Haptoglobin is a protein produced exclusively in the liver that can bind to hemoglobulin and myoglobulin (103). Interestingly, it was observed that renal cells start expressing haptoglobin in AKI (31). Paradoxically, haptoglobin was reported to participate in both pro-inflammatory and anti-inflammatory responses. On the one hand, haptoglobin could prevent respiratory burst in stimulated neutrophils, blunt endotoxin-stimulated T-lymphocyte proliferation, and modulate macrophage and dendritic cell function; on the other hand, it could also activate TLR signaling and contribute to inflammation. Furthermore, haptoglobin abruptly released from kidneys could also exert adverse pathophysiological effects in acute transplant rejection, which is also caused by AKI (30). An increase in haptoglobin levels in cardiac surgery patients has been associated with postoperative AKI indicating a direct role in ischemic AKI (32).
Heme is an iron-containing, tetrapyrrole ring that is an essential prosthetic group in an array of proteins and influences cellular and metabolic functions (33). Free heme at higher than physiological levels can be cytotoxic because of its bioreactivity and pro-oxidative effects. Higher levels of heme were observed following ischemia-induced AKI (104). Mechanistically, heme contributed toward cellular toxicity by oxidizing lipids, denaturing proteins, cytoskeletal rearrangement, inhibiting enzyme activity, denaturing DNA, and affecting mitochondrial metabolism (105). It also induced pro-inflammatory response by inducing chemokines such as MCP-1 by the action of nuclear factor κB (NF-κB) signaling, increased leukocyte recruitment, and vascular permeability (34). Pigment nephropathy due to rhabdomyolysis and hemolysis accounts for ~10% of all cases of AKI (35).
Mitochondrial Alarmins
Considered to be the powerhouse of the cell and critical for maintaining the cellular functions, mitochondria are also a source of factors that can induce cell apoptosis (106). Fragmentation of mitochondria is an important early event in the manifestation of AKI of both chemical and ischemic etiology (36). The release of cytochrome C from mitochondria into the cytoplasm is an endogenous signal for the cell to undergo apoptosis. Swollen mitochondria were observed in renal tissues in mice treated with LPS, a model of sepsis-associated AKI (107). These mitochondria stained poorly for cytochrome c oxidase, an indication of underlying reduced electron transport chain activity. The mitochondria are fast becoming a critical target, and mitochondrial DAMPs that include mitochondrial DNA (mtDNA), ATP, N-formyl peptides, TFAM, succinate, and cardiolipin [reviewed in (108, 109)] are also being identified for their pathological roles in renal injury and dysfunction as discussed below.
Mitochondrial DNA (mtDNA), identified as a DAMP, has been suggested to also act as an alarmin that upon release into the cytoplasm triggers an inflammatory response and has been proposed to be used as a potential biomarker for kidney injury (109, 110). Cellular stress results in leakage of mtDNA leading to inflammation, likely through recognition by four innate receptors: cytosolic cyclic GMP-AMP synthase (cGAS), endosomal TLR9, and two inflammasomes: absent in melanoma 2 (AIM2), and NOD, LRR, and pyrin domain-containing protein 3 (NLRP3) (41). Levels of urinary mtDNA were elevated in mice after IRI-induced AKI (42). Clinical studies have indicated an association of urinary mtDNA with initiation and progression of AKI in the surgical intensive care unit (43), cardiac surgery (42), and sepsis (44) patients.
Adenosine triphosphate (ATP) is the vital source of energy for cellular processes, and its intracellular level is regulated by mitochondrial oxidative phosphorylation. However, extracellular ATP is an indication of mechanical stress and cellular damage (38). Binding of extracellular ATP activates the membrane-anchored ionotropic P2X (P2XRs) and metabolic P2Y (P2YRs) purinergic receptors. Activation of these purinergic signals by ATP triggers a variety of biological responses such as inflammation, tissue damage, and cell proliferation in renal diseases (38). Inhibition of purinergic receptors was protective in both ischemic and sepsis-induced AKI (111, 112). ATP and selective agonists of the P2X7 receptor were shown to activate peptidyl arginine deaminase 4 (PAD4) in proximal tubular cells (PTCs) and exacerbate IRI (113). Recently, the P2X4 receptor was shown to exacerbate ischemic AKI through NLRP3 inflammasome signaling in the renal proximal convoluted tubules (PCTs) (114). CD39 and CD73 are two ectonucleotidases that break down ATP to adenosine, which has anti-inflammatory properties (115). The absence of CD73 in mice exacerbated inflammation and worsened AKI outcomes (116), whereas mice transgenic for overexpression of human CD39 were protected against AKI (117). The release of ATP to the extracellular milieu and its intracellular levels is also regulated by pannexin receptors (39). Panx1 was recently shown to induce ferroptosis in renal IRI and its deletion protected from IRI (118, 119).
Mitochondrial N-formyl peptides (FMIT, mtFPs, NFP, or mitocryptides) are similar to bacterial DAMP peptides. The evidence of the role of FMIT leading to AKI is rather indirect through the progression of sepsis. It was reported that mitochondrial N-formyl peptides induce sepsis-like syndrome, which could further affect organs including kidneys, lungs, and brain (46). It is known that a significant proportion of trauma patients presents sepsis-like syndrome without bacterial infections, and this condition is termed systemic inflammatory response syndrome (SIRS). One of the most common complications of SIRS is AKI, which is triggered by FMIT through formyl peptide receptor activation leading to hypotension and vascular collapse (45).
Mitochondrial transcription factor A (TFAM) is a member of a high mobility group (HMG) box proteins (109). It is an important regulator of the transcription and replication of mtDNA, as well as a key regulator of mitochondrial dynamics and function (47). The development of TFAM-deficient mice has enhanced our understanding of the role of TFAM in renal injury. It was recently reported using this versatile mouse model that mitochondrial damage activates the widely investigated cGAS-STING pathway leading to renal inflammation and fibrosis (47). The role of mitochondrial damage and the cGAS-STING pathway was also recapitulated recently in the cisplatin-induced AKI mouse model (48).
Succinate is an intermediate of the tricarboxylic acid cycle, which reaches extracellular milieu upon injury or ischemic conditions in the tissue (109). Succinate receptor GPR91 expressed in immature DCs and macrophages binds to the extracellular succinate and gets activated, resulting in either initiation or exacerbation of immune response (49). Plasma succinate levels were shown to be upregulated in studies on the changes in the metabolic profiles in murine AKI (50).
Cardiolipin is a class of phospholipids that account for ~20% of lipids in the inner mitochondrial membrane (120). It is critical for many mitochondrial processes such as protein import, dynamics, respiratory chain functionally, and metabolism. Extracellular cardiolipin release due to mitochondrial stress or injury is sensed by T cells through the presentation on the major histocompatibility complex–like molecule CD1d (52). Cardiolipin can also bind to NLRP3 directly, eliciting, and inflammasome-mediated immune response (53). Peroxidation and loss of cardiolipin have been shown to contribute to pathogenesis in experimental AKI (54).
Extracellular Matrix Associated Alarmins
The epithelial injury and inflammation in AKI also lead to disruption of the glycocalyx, an endothelial surface layer consisting of lectin and proteoglycan (62).
Heparin sulfate (HS) is a major component of glycocalyx that helps in the organization of ~50% of the glycocalyx. Heparanase is an endoglycosidase enzyme that functions to cleave HS. Increased expression of heparanase has been observed in AKI, suggesting it could be used as an early biomarker (62). Shedding of glycocalyx is accompanied by reduction of endothelial nitric oxide synthase and an increase in inflammation (121). Activation of heparanase was also observed early in the sepsis-induced AKI in mice and correlated with higher pro-inflammatory cytokine levels (122). Detectability of heparanase in the urine also supported its potential as an important biomarker in sepsis–AKI (63). Further, inhibitors of heparanase activation attenuated the renal transcription of the pro-inflammatory mediators (122).
Hyaluronic acid (HA) is also an important component of the extracellular matrix. It is mainly composed of N-acetyl glucosamine and glucuronic acid (64). HA synthesis has been shown to increase during fibrosis and inflammatory conditions. Endothelial cells and TECs express abnormally high levels of CD44 and HA receptor during AKI (64). Further, the uptake of HA by these cells resulted in cellular dysfunction. In a pioneering study, urinary HA was correlated with AKI in patients, also suggesting that it could be used as a biomarker to differentiate AKI from CKD in patients. Additionally, an increase in HA has been attributed to T-cell and macrophage infiltration and formation fibrosis in AKI (65).
Biglycan is expressed as a component of ECM in all organs and belongs to the small leucine-rich proteoglycan (SLRP) family that is released from the extracellular matrix (68). Overexpression of biglycan is a common clinical feature in many renal pathologies. Overexpressing biglycan triggered activation of TLR2 and TLR4 to exacerbate pathophysiology of experimental AKI (67). More recently, it was reported that biglycan activates autophagy in macrophages through a novel CD44–TLR4 signaling axis in the setting of IRI (123). Both preclinical and human studies have identified soluble biglycan as biomarkers in inflammatory renal diseases [detailed specific review in (69)].
Cell Membrane–Bound Alarmins
Hepatitis A virus cellular receptor 1 (HAVCR1), initially identified as a receptor for several viruses, is also known as T-cell immunoglobulin and mucin domain 1 (TIM-1) or kidney injury molecule 1 (KIM-1). KIM-1, although expressed in multiple tissues, is not expressed in normal kidneys; however, it gets rapidly upregulated in PCT of the kidney in AKI (55). KIM-1 was the first non-myeloid phosphatidylserine receptor identified that could transform epithelial cells into “semiprofessional” phagocytes; thus, playing a role in the removal of apoptotic cells and necrotic tissue fragments (124). Recently, KIM-1 has also been attributed to the resolution of kidney inflammation, suggesting additional possible roles for this alarmin molecule or receptor (55). KIM-1 was shown to activate the ERK/MAPK signaling to promote the migration and proliferation of renal TECs (125). KIM-1 is detected in the urine of kidney injury patients and is being evaluated as a prominent biomarkers for AKI [extensively reviewed in (56–58)].
Uromodulin or Tamm–Horsfall protein (THP) is a glycoprotein expressed in the thick ascending limb of the kidney and is the highest excreted protein in the urine following proteolytic cleavage (60). Although the function of uromodulin is not completely understood, it is proposed as a biomarker of kidney injury (60), polycystic kidney disease (126), and acute transplant rejection (127). Uromodulin was shown to promote immune cell activation via activating TLR4 in experimental studies (128). Clinical studies suggested that uromodulin may also be involved in the progression of CKD with its serum levels positively correlating with serum levels of pro-inflammatory cytokines (129). Paradoxically, uromodulin also has a protective effect in AKI. Uromodulin was shown to exhibit anti-inflammatory effects through reducing TLR4 expression in the thick ascending limb as kidneys from THP-deficient mice exhibited more inflammation and injury in the outer medulla (59). In cardiac surgery–associated AKI, a lower uromodulin-to-creatinine ratio correlated with higher odds of AKI and higher peak serum creatinine levels (130). In another clinical study in acute pancreatitis related AKI, serum uromodulin concentration had a positive correlation with GFR, and patients with AKI had lower serum uromodulin (131). Lower serum uromodulin levels were thus predictors of AKI in pediatric cardiac surgery (132), patients with cirrhosis (61), or renal cancer patients with partial nephrectomy (133).
Secreted/Granule-Derived Alarmins
Many granule-derived alarmins were initially identified as antimicrobial products secreted by cells, but their role in sterile inflammation is now increasingly recognized (134).
Defensins are a class of antimicrobial peptides, present in the granules of many cell types, and have a broad range of antimicrobial activity in both Gram-negative and Gram-positive bacteria (135). Defensins can be categorized into two families, the α-defensins and β-defensins (136). Although Paneth cells in the intestine are the main source of α-defensins in mice, higher levels of defensins were observed in the kidneys in glomerulonephritis and CKD (137). Elevated levels of defensin were detected after AKI and were shown to induce inflammation, injury, and impaired barrier functions in the gut (70). As a result, the delivery of defensins and other pro-inflammatory molecules such as IL-17A from intestinal macrophages to the liver resulted in hepatic inflammation and apoptosis. In turn, overproduction of hepatic IL-6 and TNF-α led to systemic inflammation and enhancement of renal dysfunction in a feed-forward loop (70, 138). Urinary β-defensins were proposed to be a useful biomarker in early prediction of contrast-induced nephropathy, which accounts for ~10 to 15% of hospital-acquired AKI (71).
Cathelicidins are a family of antimicrobial and immunomodulatory peptides expressed in epithelial and immune cells under homeostasis and inflammation (139). A single cathelicidin is found in humans—hcAP18/LL-37 and rodents—cathelicidin-related antimicrobial peptide (CRAMP) (140). Cathelicidin expression was significantly downregulated in clinical AKI as well as in murine models (72). NLRP3 overactivation was discovered to be one of the major effects of this deficiency in cathelicidin that causes elevated inflammatory responses and apoptosis (141).
Tissue inhibitor of metalloproteinases 2 (TIMP-2) and insulin-like growth factor-binding protein 7 (IGFBP7) have gained recognition as clinical biomarkers of AKI, collectively known as Nephrocheck™ commercially (79). TIMP-2 is a natural inhibitor of matrix metalloproteinases involved in the degradation of the extracellular matrix (142). Under steady state, TIMP-2 is expressed in monocytes, B cells, and T cells (142). Increased levels of TIMP-2 were detected in urine immediately following AKI (78). In the normal kidneys, TIMP-2 is localized in PCT. However, there was an apparent reduction of TIMP-2 signals after AKI and directly correlated to the severity of AKI (78). IGFBP-7 binds to the IGF and regulates its bioavailability in body fluids and tissues. Following AKI, a massive increase in IGFBP7 in urine was observed (78). Similar to TIMP-2 strong cortical proximal tubular staining of IGFBP7 was observed in normal under normal conditions. However, upon AKI, there was a severe reduction of proximal tubular IGFBP7 (143). Insulin-like growth factor–binding protein has been hypothesized to be involved in cellular senescence (78) and immune cell function (80). More detailed mechanistic studies are required to uncover the molecular and cellular basis of IGFBP7 in the context of inflammation.
Thymic stromal lymphopoietin (TSLP) is mainly produced from stromal and epithelial cells, and its function to promote T helper type2 (TH2) cell response has linked it to allergic inflammation (144). The TSLP levels were elevated in sepsis-associated AKI in both humans and rodent models (81). TSLP was associated with NF-κB signaling to elicit the inflammatory response. Other granule-derived peptides such as those produced by eosinophils (73), and granulysins that are secreted by cytotoxic T lymphocytes and NK cells (145), were reported in renal allograft rejection (76, 77), and may also be linked with AKI and mortality (75).
Potential Therapeutic Application of Targeting Alarmin signaling
Alarmins were initially identified as acute-phase molecules that cause immune activation and were deemed pro-inflammatory. Consequently, several approaches to inhibit alarmins and their receptors have been explored for intervention in AKI. Interestingly, several alarmins also have dual functions and can promote protective pathways and thus are being explored for therapeutic use. We review these two opposing approaches below in the context of AKI.
Inhibiting Alarmin Signaling
Nuclear Alarmins
Administration of the soluble form of IL-33 receptor ST2 (sST2) was shown to prevent the onset of acute inflammation (84). It is believed that sST2 may act as a decoy receptor and neutralizes the IL-33 activity. Treatment with sST2 in the cisplatin-induced AKI model exhibited fewer CD4-infiltrating T cells, lower serum creatinine, and decreased acute tubular necrosis (ATN) and apoptosis as compared to the untreated controls (17). In contrast, treatment with recombinant IL-33 (rIL-33) exacerbated the AKI with an increase in CD4 T-cell infiltration, serum creatinine, ATN, and apoptosis (17). Interestingly, it was observed that the administration of rIL-33 did not exacerbate AKI in CD4-deficient mice, suggesting a direct effect of IL-33 activity on CD4 T cells (17). These data indicated that inhibiting the IL-33 signaling has therapeutic potential in treating or preventing AKI. Similarly, treatment with HMGB1 neutralizing antibody after IRI led to attenuation of TNF-α and MCP-1 levels and protected against kidney IRI, as evidenced by lower levels of serum creatinine, tubulointerstitial neutrophil infiltration, and tubular damage compared to the control mice (13). Various IL-1β/IL-1α/IL-1R1–specific inhibitory molecules are currently in different phases of clinical trials (16). Neutralization of histones using targeted neutralizing antibody also led to the attenuating pathogenic effect of histones, thus preventing AKI (20).
Cytosolic Alarmins
HSP90 transduces signals via binding to the transforming growth factor β type I (TGFβI) and type II (TGFβII) receptors (22). Blocking the interaction of HSP90 with TGFβII receptor by using 17-allyamino-17-demethoxygeldanamycin reduced fibrosis by promoting the ubiquitination of TGFβII. S100A8/A9–TLR4–NLRP3 inflammasome pathway was shown to trigger inflammation, apoptosis, and tissue injury during AKI. Inhibition of this pathway through siRNA to TLR4–NLRP3 ameliorated the kidney function in contrast-induced acute kidney injury model (24). Inhibition of TSLP, a TH2-inducing cytokine, with siRNA also resulted in lowering the sepsis-associated organ dysfunction and inflammatory cytokine levels (81).
In a rat model of cisplatin-induced AKI, moderate hyperuricemia was associated with the absence of intrarenal crystals but correlated with greater tubular injury, significant macrophage infiltration, and increased expression of MCP-1 (27). Treatment with rasburicase, a uric acid oxidase, reversed the inflammation and tubular injury (28). Many clinical approaches employed in AKI, including allopurinol, febuxostat, and Renal Replacement Therapy (RRT), may act by decreasing circulating urate to reduce its pro-inflammatory effects (29).
Mitochondrial Alarmins
Mitochondrial fragmentation has been thought to be one of the possible mechanisms contributing to injury in AKI. Inhibition of mitochondrial fragments was observed by blocking fission protein Drp1 along with the reduction in cytochrome c release and apoptosis (36). Similar results were obtained by blocking Drp1 using a new pharmacological inhibitor mdivi-1 (36). Targeting mitochondria by promoting mitochondrial health for therapeutic effects on AKI includes promoting metabolism by augmenting fatty acid oxidation using peroxisome proliferator-activated receptor α (PPARα) overexpression (146) or augmenting ETC using CoQ10 (ubiquinone) (147). Mitochondrial fragmentation induces ROS, which was targeted using MitoQ and SS-31 to attenuate AKI (148). Cyclosporine that is used in transplantation may also counter AKI by regulating mitochondrial membrane permeability by inhibiting cyclophilin D (149). Agents such as temsirolimus (150) function by targeting mitophagy through activating mTOR signaling. Finally, improving mitochondrial biogenesis by enhancing nuclear transcription of mitochondrial proteins using PPARγ-coactivator-1α (PGC1α) (107) or by activating β-adrenergic receptors using formoterol (151) may also contribute to protection from AKI by reducing mitochondrial fragmentation. Compound SS-31, which reenergizes mitochondria by preventing matrix swelling and preserving cristae structure, thus restoring ATP, is being clinically tested. SS-31 selectively binds to cardiolipin, preventing its peroxidation and loss (37).
Depletion of extracellular ATP with apyrase, or blocking of P2XR with pyridoxal phosphate-6-azophenyl-2′,4′-disulfonic acid (PPADS), has been shown to prevent necrosis-related inflammation (152). In the same study, treatment with A438079, a selective P2X7 receptor inhibitor or knockdown of the P2X7 receptor with siRNA, reduced the apoptosis of PTCs. The use of recombinant alkaline phosphatase has been tested both experimentally (153) and clinically (40) in sepsis-associated AKI. It is believed that the mechanism of action may involve dephosphorylation of LPS for reduced TLR activation (154) and of ATP for conversion to the anti-inflammatory adenosine (155). Binding of adenosine or its synthetic analogs to adenosine receptors protected mice from IRI in an IL-11–dependent manner (156). Adenosine was also shown to induce immune tolerance through dendritic cells (157) and T-regulatory cells (Tregs) (158). Conversely, inhibition of adenosine kinase with a small molecule (ABT-702) to prevent the conversion of adenosine to ATP was protective in cisplatin nephrotoxicity (159). Paradoxically, extracellular nucleotides including ATP released from dying cells were also shown to promote wound repair in renal tubular injury (160).
Secreted and Extracellular Alarmins
Blocking of glycans with doxycycline, a broad-spectrum matrix metalloprotease inhibitor, was shown to restrict the secretion of pro-inflammatory cytokines in cisplatin and IRI-induced AKI (161, 162). Heparanase inhibitors such as PG545 was found protective in experimental ischemic IRI (63) and is currently in clinical testing. Mice receiving a diet containing 4-methylumbelliferone, a potent hyaluronic acid synthesis inhibitor, resulted in attenuation of AKI (66). Pharmacological treatment with a zinc chelator, dithizone, resulted in depletion of Paneth cell granules in adult mice (163) and rats (164). These mice exhibited less leukocyte infiltration, pro-inflammatory cytokine generation, and reduced epithelial necrosis and apoptosis. In contrast, studies have also indicated that a chronic loss of Paneth cell α-defensin expression could also skew toward a more pro-inflammatory phenotype (165). These opposing outcomes warrant additional mechanistic studies to fully understand the role of defensins in AKI.
Direct Application of Alarmins
Nuclear Alarmins
In contrast to the pro-inflammatory reports of IL-33, evidence also suggests that IL-33 is a potential mediator of type 2 immunity and a regulator of the protective immune response (166, 167). We identified that ST2, the receptor for IL-33, is regulated by IL-2 (168) and is expressed on a major subset of Tregs (169). Based on our data that IL-2 and IL-33 by themselves increased Tregs and partially protected from IRI and that these cytokines synergize to completely protect from AKI, we generated a novel hybrid cytokine (termed IL233) bearing activities of IL-2 and IL-33 in a single molecule (169). Treatment with IL233 robustly increased Tregs and the group 2 innate lymphoid cells (ILC2) and strongly protected kidneys from IRI, as well as cisplatin- and doxorubicin-induced nephrotoxic injuries (169, 170). A similar strategy of using exogenous IL-33 alone was demonstrated to increase ILC2 to protect from IRI in T cell–independent manner (171). Interestingly, reduction or depletion of ILC2 did not affect the severity of IRI in a mouse model, suggesting that ILC2 may be redundant for IRI (172), despite the finding that the adoptive transfer of ex vivo–expanded ILC2 was protective in murine IRI (169).
Cytoplasmic Alarmins
Preconditioning the mice with rHMGB1 prior to IRI protects the kidney against IRI was indicated by low serum creatinine, tubular damage, and tubulointerstitial neutrophil and macrophage infiltration (173). Pretreatment with rHMGB1 resulted in the upregulation of Siglec-G, which in turn negatively regulated HMGB1-mediated TLR4 pathway activation. This indicated significant protection from renal IRI from the activation of TLR4-dependent inflammatory response. It was also observed that lentivirus-mediated renal overexpression of HSP27 prevented the loss of renal function and decreased necrosis, inflammation, apoptosis, and F-actin cytoskeleton after IRI injury in mice (174). In a retrospective observational study, it was found that the intraoperative administration of haptoglobin administration was independently associated with a lower risk of AKI incidence after cardiovascular surgery (175).
Studies in 1989 identified heme oxygenase 1 (HO-1) as a protein induced in hypoxic cells. Protective responses of HO have been confirmed in various AKI studies (176). HO-1 participates in the dissipation of heme, thereby protecting the kidneys from inflammation and cellular damage. Induction of HO-1 and ferritin in the kidney protects against heme-induced kidney injury (177). HO-1 induction by granulocyte colony-stimulating factor has been shown to protect against AKI both in vivo and in vitro (178). Adiponectin, a cytokine produced from white fat, induces HO-1 in renal epithelial cells in vitro and prevents AKI following IRI (179). Along with heme, ferrous iron (Fe) that is found in heme also correlated with AKI (180). Administration of the iron-regulating hormone hepcidin reduced inflammation and decreased oxidative stress in mouse models of AKI (181). Further, the administration of a furin inhibitor to induce high levels of hepcidin also reduced AKI in mouse models (182).
Extracellular Matrix and Cell-Surface Alarmins
The use of extracellular matrix-associated alarmins for protection in AKI is largely understudied but is gaining attraction. In an interesting study (183), an HA-curcumin prodrug targeting the HA receptor-CD44 could assist in epithelial cell survival from oxidative stress during AKI. CRAMP-deficient (Cnlp−/−) mice exhibited exacerbated renal dysfunction accompanied by aggravated inflammatory response and apoptosis (72). Exogenous treatment with CRAMP markedly attenuated AKI accompanied by reduced NLRP3 orchestrated inflammatory response and apoptosis. In LPS-induced inflammatory settings, it was observed that overexpression of TIMP-2, a major diagnostic marker of AKI, significantly attenuated the production of nitric oxide, TNF-α, IL-1β, and ROS with increased production of anti-inflammatory cytokine (IL-10) (184). Future studies on the use of TIMP-2 are likely to produce interesting results.
Implications of Alarmins in Repair Post-AKI
The renoprotective role of alarmins also suggests their potential in repair after renal injury. Stem cells play an important role in tissue homeostasis, as well as tissue repair following injury (185). Researchers have used exogenous stem cells to improve tissue regeneration using a variety of approaches. However, still, there is a very limited clinical success than anticipated especially for solid organ injuries (185). Alternatively, harnessing the endogenous tissue-resident stem cells for mediating repair could be promising. In a breakthrough study in 1970, it was observed that priming injury at a distant site at the time of, or before the second trauma, resulted in accelerated repair (186, 187). In a recent study, Lee et al. (188) have used the alarmin, HMGB1, to accelerate repair using a bone fracture model. Exogenous treatment with HMGB1 accelerated facture healing through the formation of heterodimer complex between HMGB1 and chemokine, CXCL12 (stromal cell–derived factor1), which then signals through CXCR4 receptor (188). Because remote ischemic preconditioning was accompanied by an upregulation of HMGB1 (189), preconditioning with recombinant HMGB1 was tested and found to be protective in AKI (173). Such an approach may as well be investigated to promote repair in AKI.
Heat shock proteins, although identified as biomarkers for AKI, are now being investigated for their beneficial role in AKI. HSP73 and HSP90 were found to be induced in the injured PTC and loop of Henle early on after injury and then were upregulated again in the regenerating cells, suggesting these HSPs may participate in repair post-IRI, and may be exploited in future studies (94). HSP70 was shown to interact with cytoskeletal elements during the restoration of the cytoskeletal structure and polarity of proximal tubules after ischemic injury, indicating the role of HSP70 in renal repair (190). An interesting concept is that T-cell reactivity to HSP may induce tolerogenic responses, which may be beneficial for the resolution of inflammatory diseases (21, 191, 192). Indeed, a recent study showed that, in a murine model of IRI, heat preconditioning induced the release of HSP-70, which in turn promoted the expansion of Tregs that was renoprotective (193, 194).
A reparative role of Tregs in AKI was initially shown in murine IRI through depletion studies (195). Recently, we demonstrated that treatment with the fusion protein IL233 utilizes the synergy of IL-2 with the IL-33 alarmin in protection when administered after the onset of injury (169). IL233 treatment, initiated 2 weeks after renal injury, induced near-complete restoration of renal structure and function (170). IL233 treatment invoked the proliferation and renal recruitment of Tregs and ILC2s. Antibody-mediated depletion of these cells ameliorated the restoration of renal injury. Further, mobilization of these cells near the site of injury promoted the recruitment of progenitor cells in the kidneys. It remains to be evaluated whether this may be either a direct effect of these cells or through inducing an anti-inflammatory milieu, which may be conducive for progenitor cells to promote regenerative responses. Treatment with IL233 after the onset of lupus nephritis and diabetic nephropathy in animal models also induced persistent remission, suggestive of a reparative role of IL-33 alarmin in chronic renal injury (170, 196, 197). Current studies in our group are addressing the role of the IL-33/ST2 and IL233 in the repair of renal injury in both an immune-dependent and independent manner.
Conclusion
The immunoregulatory potential of alarmins, as well as their predictive value as a biomarker in a host of disease conditions, renders the study of alarmins beneficial for clinical applications. Despite all the advances in the understating of the pathophysiology of kidney diseases, the dearth of treatment strategies for AKI remains a major unmet clinical need. Novel therapeutic options or perhaps a combination of those in a concerted manner is required to solve this problem. Exploring the role of alarmins as diagnostic markers, immunomodulators, and harbingers of repair could be one of the strategies that may lead to therapy of AKI.
Author Contributions
RS conceived the idea and performed the final revision. VS performed the bulk of literature search in collaboration with RV and MD. RS, VS, RV, and MD co-wrote the manuscript. All authors contributed to the article and approved the submitted version.
Funding
The authors of this manuscript were supported by LaunchPad Diabetes Fund (RS); and National Institute of Diabetes and Kidney Diseases and National Institute of Allergy and Infectious Diseases of the NIH, under awards R01DK104963 (RS), R21DK112105 (RS, M. Rosner and K. Lynch) 1R01DK105833 (RS and S.M. Fu), 2R01AI116725 (Subcontract to RS from Dr. F. Perrino, Wake Forest University).
Conflict of Interest
The authors declare that the research was conducted in the absence of any commercial or financial relationships that could be construed as a potential conflict of interest.
References
1. Chawla LS, Bellomo R, Bihorac A, Goldstein SL, Siew ED, Bagshaw SM, et al. Acute kidney disease and renal recovery: consensus report of the Acute Disease Quality Initiative (ADQI) 16 Workgroup. Nat Rev Nephrol. (2017) 13:241–57. doi: 10.1038/nrneph.2017.2
2. Ronco C, Bellomo R, Kellum JA. Acute kidney injury. Lancet. (2019) 380:756–66. doi: 10.1016/S0140-6736(19)32563-2
3. Glodowski SD, Wagener G. New insights into the mechanisms of acute kidney injury in the intensive care unit. J Clin Anesth. (2015) 27:175–80. doi: 10.1016/j.jclinane.2014.09.011
4. Tögel F, Westenfelder C. Recent advances in the understanding of acute kidney injury. F1000Prime Rep. (2014) 6:285–307. doi: 10.12703/P6-83
5. Mezzapelle R, Venereau E, Bianchi ME. Stress and alarmins. Report from the 9th iD&EAs meeting. Cell Death Dis. (2019) 10:937. doi: 10.1038/s41419-019-2165-1
6. Matta BM, Reichenbach DK, Blazar BR, Turnquist HR. Alarmins and their receptors as modulators and indicators of alloimmune responses. Am J Transplant. (2017) 17:320–7. doi: 10.1111/ajt.13887
7. Bianchi ME. DAMPs, PAMPs and alarmins: all we need to know about danger. J Leukoc Biol. (2007) 81:1–5. doi: 10.1189/jlb.0306164
8. Matzinger P. Friendly and dangerous signals: is the tissue in control? Nat Immunol. (2007) 8:11–13. doi: 10.1038/ni0107-11
9. Janeway CA, Medzhitov R. Innate immune recognition. Annu Rev Immunol. (2002) 20:197–216. doi: 10.1146/annurev.immunol.20.083001.084359
10. Amarante-Mendes GP, Adjemian S, Branco LM, Zanetti LC, Weinlich R, Bortoluci KR. Pattern recognition receptors and the host cell death molecular machinery. Front Immunol. (2018) 9:2379. doi: 10.3389/fimmu.2018.02379
11. Gong T, Liu L, Jiang W, Zhou R. DAMP-sensing receptors in sterile inflammation and inflammatory diseases. Nat Rev Immunol. (2020) 20:95–112. doi: 10.1038/s41577-019-0215-7
12. Chen Q, Guan X, Zuo X, Wang J, Yin W. The role of high mobility group box 1 (HMGB1) in the pathogenesis of kidney diseases. Acta Pharm Sin B. (2016) 6:183–8. doi: 10.1016/j.apsb.2016.02.004
13. Wu H, Ma J, Wang P, Corpuz TM, Panchapakesan U, Wyburn KR, et al. HMGB1 contributes to kidney ischemia reperfusion injury. J Am Soc Nephrol. (2010) 21:1878–90. doi: 10.1681/ASN.2009101048
14. Zakiyanov O, Kriha V, Vachek J, Zima T, Tesar V, Kalousova M. Placental growth factor, pregnancy-associated plasma protein-A, soluble receptor for advanced glycation end products, extracellular newly identified receptor for receptor for advanced glycation end products binding protein and high mobility group box 1 levels in patients with acute kidney injury: a cross sectional study. BMC Nephrol. (2013) 14:245. doi: 10.1186/1471-2369-14-245
15. Lee JW, Nam WJ, Han MJ, Shin JH, Kim JG, Kim SH, et al. Role of IL-1α in cisplatin-induced acute renal failure in mice. Korean J Intern Med. (2011) 26:187–94. doi: 10.3904/kjim.2011.26.2.187
16. Anders H-J. Brief review of inflammasomes and alarmins: IL-1b and IL-1a in kidney disease. J Am Soc Nephrol. (2016) 27:2564–75. doi: 10.1681/ASN.2016020177
17. Akcay A, Nguyen Q, He Z, Turkmen K, Won Lee D, Hernando AA, et al. IL-33 exacerbates acute kidney injury. J Am Soc Nephrol. (2011) 22:2057–67. doi: 10.1681/ASN.2010091011
18. Ferhat M, Robin A, Giraud S, Sena S, Goujon JM, Touchard G, et al. Endogenous IL-33 contributes to kidney ischemia-reperfusion injury as an alarmin. J Am Soc Nephrol. (2018) 29:1272–88. doi: 10.1681/ASN.2017060650
19. Thierry A, Giraud S, Robin A, Barra A, Bridoux F, Ameteau V, et al. The alarmin concept applied to human renal transplantation: evidence for a differential implication of HMGB1 and IL-33. PLoS ONE. (2014) 9:e88742. doi: 10.1371/journal.pone.0088742
20. Allam R, Scherbaum CR, Darisipudi MN, Mulay SR, Hägele H, Lichtnekert J, et al. Histones from dying renal cells aggravate kidney injury via TLR2 and TLR4. J Am Soc Nephrol. (2012) 23:1375–88. doi: 10.1681/ASN.2011111077
21. Guo Q, Du X, Zhao Y, Zhang D, Yue L, Wang Z. Ischemic postconditioning prevents renal ischemia reperfusion injury through the induction of heat shock proteins in rats. Mol Med Rep. (2014) 10:2875–81. doi: 10.3892/mmr.2014.2641
22. Noh H, Kim HJ, Yu MR, Kim WY, Kim J, Ryu JH, et al. Heat shock protein 90 inhibitor attenuates renal fibrosis through degradation of transforming growth factor-β type II receptor. Lab Investig. (2012) 92:1583–96. doi: 10.1038/labinvest.2012.127
23. Chebotareva N, Bobkova I, Shilov E. Heat shock proteins and kidney disease: perspectives of HSP therapy. Cell Stress Chaperones. (2017) 22:319–43. doi: 10.1007/s12192-017-0790-0
24. Tan X, Zheng X, Huang Z, Lin J, Xie C, Lin Y. Involvement of S100A8/A9-TLR4-NLRP3 inflammasome pathway in contrast-induced acute kidney injury. Cell Physiol Biochem. (2017) 43:209–22. doi: 10.1159/000480340
25. Nikolakopoulou Z, Hector LR, Creagh-Brown BC, Evans TW, Quinlan GJ, Burke-Gaffney A. Plasma S100A8/A9 heterodimer is an early prognostic marker of acute kidney injury associated with cardiac surgery. Biomark Med. (2019) 13:205–18. doi: 10.2217/bmm-2018-0238
26. Johnson RJ, Bakris GL, Borghi C, Chonchol MB, Feldman D, Lanaspa MA, et al. Hyperuricemia, acute and chronic kidney disease, hypertension, and cardiovascular disease: report of a scientific workshop organized by the national kidney foundation. Am J Kidney Dis. (2018) 71:851–65. doi: 10.1053/j.ajkd.2017.12.009
27. Hahn K, Kanbay M, Lanaspa MA, Johnson RJ, Ejaz AA. Serum uric acid and acute kidney injury: a mini review. J Adv Res. (2017) 8:529–36. doi: 10.1016/j.jare.2016.09.006
28. Galardy PJ, Hochberg J, Perkins SL, Harrison L, Goldman S, Cairo MS. Rasburicase in the prevention of laboratory/clinical tumour lysis syndrome in children with advanced mature B-NHL: a Children's Oncology Group report. Br J Haematol. (2013) 163:365–72. doi: 10.1111/bjh.12542
29. Benn CL, Dua P, Gurrell R, Loudon P, Pike A, Ian Storer R, et al. Physiology of hyperuricemia and urate-lowering treatments. Front Med. (2018) 5:160. doi: 10.3389/fmed.2018.00160
30. Shen H, Song Y, Colangelo CM, Wu T, Bruce C, Scabia G, et al. Haptoglobin activates innate immunity to enhance acute transplant rejection in mice. J Clin Invest. (2012) 122:383–7. doi: 10.1172/JCI58344
31. Zager RA, Vijayan A, Johnson ACM. Proximal tubule haptoglobin gene activation is an integral component of the acute kidney injury “stress response.” Am J Physiol Renal Physiol. (2012) 303:F139–48. doi: 10.1152/ajprenal.00168.2012
32. Feng C, Naik BI, Xin W, Ma JZ, Scalzo DC, Thammishetti S, et al. Haptoglobin 2-2 phenotype is associated with increased acute kidney injury after elective cardiac surgery in patients with diabetes mellitus. J Am Heart Assoc. (2017) 6:e006565. doi: 10.1161/JAHA.117.006565
33. Tracz MJ, Alam J, Nath KA. Physiology and pathophysiology of heme: implications for kidney disease. J Am Soc Nephrol. (2007) 18:414–20. doi: 10.1681/ASN.2006080894
34. Wagener FADTG, Eggert A, Boerman OC, Oyen WJG, Verhofstad A, Abraham NG, et al. Heme is a potent inducer of inflammation in mice and is counteracted by heme oxygenase. Blood. (2001) 98:1802–11. doi: 10.1182/blood.V98.6.1802
35. Sakthirajan R, Dhanapriya J, Varghese A, Saravanakumar K, Dineshkumar T, Balasubramaniyan T, et al. Clinical profile and outcome of pigment-induced nephropathy. Clin Kidney J. (2018) 11:348–52. doi: 10.1093/ckj/sfx121
36. Brooks C, Wei Q, Cho SG, Dong Z. Regulation of mitochondrial dynamics in acute kidney injury in cell culture and rodent models. J Clin Invest. (2009) 119:1275–85. doi: 10.1172/JCI37829
37. Birk AV, Liu S, Soong Y, Mills W, Singh P, Warren JD, et al. The mitochondrial-targeted compound SS-31 re-energizes ischemic mitochondria by interacting with cardiolipin. J Am Soc Nephrol. (2013) 24:1250–61. doi: 10.1681/ASN.2012121216
38. Solini A, Usuelli V, Fiorina P. The dark side of extracellular ATP in kidney diseases. J Am Soc Nephrol. (2015) 26:1007–16. doi: 10.1681/ASN.2014070721
39. Penuela S, Gehi R, Laird DW. The biochemistry and function of pannexin channels. Biochim Biophys Acta Biomembr. (2013) 1828:15–22. doi: 10.1016/j.bbamem.2012.01.017
40. Pickkers P, Mehta RL, Murray PT, Joannidis M, Molitoris BA, Kellum JA, et al. Effect of human recombinant alkaline phosphatase on 7-day creatinine clearance in patients with sepsis-associated acute kidney injury a randomized clinical trial. J Am Med Assoc. (2018) 320:1998–2009. doi: 10.1001/jama.2018.14283
41. West AP, Shadel GS. Mitochondrial DNA in innate immune responses and inflammatory pathology. Nat Rev Immunol. (2017) 17:363–75. doi: 10.1038/nri.2017.21
42. Whitaker RM, Stallons LJ, Kneff JE, Alge JL, Harmon JL, Rahn JJ, et al. Urinary mitochondrial DNA is a biomarker of mitochondrial disruption and renal dysfunction in acute kidney injury. Kidney Int. (2015) 88:1336–44. doi: 10.1038/ki.2015.240
43. Hu Q, Ren J, Wu J, Li G, Wu X, Liu S, et al. Urinary mitochondrial DNA levels identify acute kidney injury in surgical critical illness patients. Shock. (2017) 48:11–17. doi: 10.1097/SHK.0000000000000830
44. Hu Q, Ren J, Ren H, Wu J, Liu S, Wang G, et al. Urinary mitochondrial DNA identifies renal dysfunction and mitochondrial damage in sepsis-induced acute kidney injury. Oxid Med Cell Longev. (2018) 2018:8074936. doi: 10.1155/2018/8074936
45. Wenceslau CF, McCarthy CG, Goulopoulou S, Szasz T, NeSmith EG, Webb RC. Mitochondrial-derived N-formyl peptides: novel links between trauma, vascular collapse and sepsis. Med Hypotheses. (2013) 81:532–5. doi: 10.1016/j.mehy.2013.06.026
46. Wenceslau CF, McCarthy CG, Szasz T, Goulopoulou S, Clinton Webb R. Mitochondrial N-formyl peptides induce cardiovascular collapse and sepsis-like syndrome. Am J Physiol Hear Circ Physiol. (2015) 308:768–77. doi: 10.1152/ajpheart.00779.2014
47. Chung KW, Dhillon P, Huang S, Sheng X, Shrestha R, Qiu C, et al. Mitochondrial damage and activation of the STING pathway lead to renal inflammation and fibrosis. Cell Metab. (2019) 30:784–99.e5. doi: 10.1016/j.cmet.2019.08.003
48. Maekawa H, Inoue T, Ouchi H, Jao TM, Inoue R, Nishi H, et al. Mitochondrial damage causes inflammation via Cgas-sting signaling in acute kidney injury. Cell Rep. (2019) 29:1261–73.e6. doi: 10.1016/j.celrep.2019.09.050
49. Littlewood-Evans A, Sarret S, Apfel V, Loesle P, Dawson J, Zhang J, et al. GPR91 senses extracellular succinate released from inflammatory macrophages and exacerbates rheumatoid arthritis. J Exp Med. (2016) 213:1655–62. doi: 10.1084/jem.20160061
50. Wei Q, Xiao X, Fogle P, Dong Z. Changes in metabolic profiles during acute kidney injury and recovery following ischemia/reperfusion. PLoS ONE. (2014) 9:e106647. doi: 10.1371/journal.pone.0106647
51. Wijermars LGM, Schaapherder AF, Kostidis S, Wüst RCI, Lindeman JH. Succinate accumulation and ischemia–reperfusion injury: of mice but not men, a study in renal ischemia–reperfusion. Am J Transplant. (2016) 16:2741–6. doi: 10.1111/ajt.13793
52. Dieudé M, Striegl H, Tyznik AJ, Wang J, Behar SM, Piccirillo CA, et al. Cardiolipin binds to CD1D and stimulates CD1D-restricted γδ T cells in the normal murine repertoire. J Immunol. (2011) 186:4771–781. doi: 10.4049/jimmunol.1000921
53. Iyer SS, He Q, Janczy JR, Elliott EI, Zhong Z, Olivier AK, et al. Mitochondrial cardiolipin is required for Nlrp3 inflammasome activation. Immunity. (2013) 39:311–23. doi: 10.1016/j.immuni.2013.08.001
54. Eirin A, Lerman A, Lerman LO. The emerging role of mitochondrial targeting in kidney disease. In: Barrett JE, Singh H, Sheu S-S, editors. Handbook of Experimental Pharmacology. New York, NY: Springer LLC (2019). p. 229–50.
55. Bonventre JV. Kidney injury molecule-1 (KIM-1): a urinary biomarker and much more. Nephrol Dial Transplant. (2009) 24:3265–8. doi: 10.1093/ndt/gfp010
56. Parikh CR, Thiessen-Philbrook H, Garg AX, Kadiyala D, Shlipak MG, Koyner JL, et al. Performance of kidney injury molecule-1 and liver fatty acid-binding protein and combined biomarkers of AKI after cardiac surgery. Clin J Am Soc Nephrol. (2013) 8:1079–88. doi: 10.2215/CJN.10971012
57. Ko GJ, Grigoryev DN, Linfert D, Jang HR, Watkins T, Cheadle C, et al. Transcriptional analysis of kidneys during repair from AKI reveals possible roles for NGAL and KIM-1 as biomarkers of AKI-to-CKD transition. Am J Physiol Ren Physiol. (2010) 298:F1472–83. doi: 10.1152/ajprenal.00619.2009
58. Yin C, Wang N. Kidney injury molecule-1 in kidney disease. Ren Fail. (2016) 38:1567–73. doi: 10.1080/0886022X.2016.1193816
59. El-Achkar TM, Wu XR, Rauchman M, McCracken R, Kiefer S, Dagher PC. Tamm-Horsfall protein protects the kidney from ischemic injury by decreasing inflammation and altering TLR4 expression. Am J Physiol Ren Physiol. (2008) 295:F534–44. doi: 10.1152/ajprenal.00083.2008
60. Rosin DL, Okusa MD. Dangers within: DAMP responses to damage and cell death in kidney disease. J Am Soc Nephrol. (2011) 22:416–25. doi: 10.1681/ASN.2010040430
61. Patidar KR, Garimella PS, Macedo E, Slaven JE, Ghabril MS, Weber RE, et al. Admission plasma uromodulin and the risk of acute kidney injury in hospitalized patients with cirrhosis: a pilot study. Am J Physiol Gastrointest Liver Physiol. (2019) 317:G447–52. doi: 10.1152/ajpgi.00158.2019
62. Arfian N, Ratna Sari DC, Romi MM, Wibisono DP, Emoto N. Heparanase expression in renal interstitial may contribute to epithelial and endothelial cells injuries after kidney ischemic/ reperfusion episode in mice. KnE Life Sci. (2015) 2:70. doi: 10.18502/kls.v2i1.119
63. Abassi Z, Hamoud S, Hassan A, Khamaysi I, Nativ O, Heyman SN, et al. Involvement of heparanase in the pathogenesis of acute kidney injury: nephroprotective effect of PG545. Oncotarget. (2017) 8:34191–204. doi: 10.18632/oncotarget.16573
64. Akin D, Ozmen S, Yilmaz ME. Hyaluronic acid as a new biomarker to differentiate acute kidney injury from chronic kidney disease. Iran J Kidney Dis. (2017) 11:409–13.
65. Steen EH, Wang X, Balaji S, Butte MJ, Bollyky PL, Keswani SG. The role of the anti-inflammatory cytokine interleukin-10 in tissue fibrosis. Adv Wound Care. (2020) 9:184–98. doi: 10.1089/wound.2019.1032
66. Colombaro V, Declèves A-E, Jadot I, Voisin V, Giordano L, Habsch I, et al. Inhibition of hyaluronan is protective against renal ischaemia-reperfusion injury. Nephrol Dial Transpl. (2013) 28:2484–93. doi: 10.1093/ndt/gft314
67. Moreth K, Frey H, Hubo M, Zeng-Brouwers J, Nastase MV, Hsieh LTH, Schaefer L. Biglycan-triggered TLR-2- and TLR-4-signaling exacerbates the pathophysiology of ischemic acute kidney injury. Matrix Biol. (2014) 35:143–51. doi: 10.1016/j.matbio.2014.01.010
68. Stokes MB, Holler S, Cui Y, Hudkins KL, Eitner F, Fogo A, et al. Expression of decorin, biglycan, and collagen type I in human renal fibrosing disease. Kidney Int. (2000) 57:487–98. doi: 10.1046/j.1523-1755.2000.00868.x
69. Hsieh LTH, Nastase MV, Zeng-Brouwers J, Iozzo R V, Schaefer L. Soluble biglycan as a biomarker of inflammatory renal diseases. Int J Biochem Cell Biol. (2014) 54:223–35. doi: 10.1016/j.biocel.2014.07.020
70. Park SW, Kim M, Kim JY, Ham A, Brown KM, Mori-Akiyama Y, et al. Paneth cell–mediated multiorgan dysfunction after acute kidney injury. J Immunol. (2012) 189:5421–33. doi: 10.4049/jimmunol.1200581
71. Bennett MR, Ravipati N, Ross G, Nguyen MT, Hirsch R, Beekman RH, et al. Using proteomics to identify preprocedural risk factors for contrast induced nephropathy. Proteomics Clin Appl. (2008) 2:1058–64. doi: 10.1002/prca.200780141
72. Pan LL, Liang W, Ren Z, Li C, Chen Y, Niu W, et al. Cathelicidin-related antimicrobial peptide protects against ischaemia reperfusion-induced acute kidney injury in mice. Br J Pharmacol. (2020) 177:2726–42. doi: 10.1111/bph.14998
73. Gauckler P, Shin J, Mayer G, Kronbichler A. Eosinophilia and kidney disease: more than just an incidental finding? J Clin Med. (2018) 7:529. doi: 10.3390/jcm7120529
74. Lee HT, Park SW, Kim M, D'Agati VD. Acute kidney injury after hepatic ischemia and reperfusion injury in mice. Lab Investig. (2009) 89:196–208. doi: 10.1038/labinvest.2008.124
75. Chung WH, Chang WC, Stocker SL, Juo CG, Graham GG, Lee MHH, et al. Insights into the poor prognosis of allopurinol-induced severe cutaneous adverse reactions: the impact of renal insufficiency, high plasma levels of oxypurinol and granulysin. Ann Rheum Dis. (2015) 74:2157–64. doi: 10.1136/annrheumdis-2014-205577
76. Kotsch K, Mashreghi MF, Bold G, Tretow P, Beyer J, Matz M, et al. Enhanced granulysin mRNA expression in urinary sediment in early and delayed acute renal allograft rejection. Transplantation. (2004) 77:1866–75. doi: 10.1097/01.TP.0000131157.19937.3F
77. Sarwal MM, Jani A, Chang S, Huie P, Wang Z, Salvatierra O, et al. Granulysin expression is a marker for acute rejection and steroid resistance in human renal transplantation. Hum Immunol. (2001) 62:21–31. doi: 10.1016/S0198-8859(00)00228-7
78. Johnson ACM, Zager RA. Mechanisms underlying increased TIMP2 and IGFBP7 urinary excretion in experimental AKI. J Am Soc Nephrol. (2018) 29:2157–67. doi: 10.1681/ASN.2018030265
79. Xie Y, Ankawi G, Yang B, Garzotto F, Passannante A, Breglia A, et al. Tissue inhibitor metalloproteinase-2 (TIMP-2)∙ IGF-binding protein-7 (IGFBP7)levels are associated with adverse outcomes in patients in the intensive care unit with acute kidney injury. Kidney Int. (2019) 95:1486–93. doi: 10.1016/j.kint.2019.01.020
80. Ding H, Wu T. Insulin-like growth factor binding proteins in autoimmune diseases. Front Endocrinol. (2018) 9:499. doi: 10.3389/fendo.2018.00499
81. Han N-R, Moon P-D, Kim H-M, Jeong H-J. TSLP exacerbates septic inflammation via Murine Double Minute 2 (MDM2) signaling pathway. J Clin Med. (2019) 8:1350. doi: 10.3390/jcm8091350
82. Dinarello CA. Overview of the IL-1 family in innate inflammation and acquired immunity. Immunol Rev. (2018) 281:8–27. doi: 10.1111/imr.12621
83. Privratsky JR, Zhang J, Lu X, Rudemiller N, Wei Q, Yu YR, et al. Interleukin 1 receptor (IL-1R1) activation exacerbates toxin-induced acute kidney injury. Am J Physiol Ren Physiol. (2018) 315:F682–91. doi: 10.1152/ajprenal.00104.2018
84. Reichenbach DK, Schwarze V, Matta BM, Tkachev V, Lieberknecht E, Liu Q, et al. The IL-33/ST2 axis augments effector T-cell responses during acute GVHD. Blood. (2015) 125:3183–92. doi: 10.1182/blood-2014-10-606830
85. Das N, Dewan V, Grace PM, Gunn RJ, Tamura R, Tzarum N, et al. HMGB1 activates proinflammatory signaling via TLR5 leading to allodynia. Cell Rep. (2016) 17:1128–40. doi: 10.1016/j.celrep.2016.09.076
86. Leelahavanichkul A, Huang Y, Hu X, Zhou H, Tsuji T, Chen R, et al. Chronic kidney disease worsens sepsis and sepsis-induced acute kidney injury by releasing high mobility group box protein-1. Kidney Int. (2011) 80:1198–211. doi: 10.1038/ki.2011.261
87. Lau A, Wang S, Liu W, Haig A, Zhang ZX, Jevnikar AM. Glycyrrhizic acid ameliorates HMGB1-mediated cell death and inflammation after renal ischemia reperfusion injury. Am J Nephrol. (2014) 40:84–95. doi: 10.1159/000364908
88. Zhang Y-X, Zhang J-R, Wang Z-G. Mycophenolate mofetil affects monocyte toll-like receptor 4 signaling during mouse renal ischemia/reperfusion injury. Chin Med J. (2013) 126:1224–9. doi: 10.1016/j.trim.2010.04.002
89. Ruan Y, Wang L, Zhao Y, Yao Y, Chen S, Li J, et al. Carbon monoxide potently prevents ischemia-induced high-mobility group box 1 translocation and release and protects against lethal renal ischemia-reperfusion injury. Kidney Int. (2014) 86:525–37. doi: 10.1038/ki.2014.80
90. Silk E, Zhao H, Weng H, Ma D. The role of extracellular histone in organ injury. Cell Death Dis. (2017) 8:e2812. doi: 10.1038/cddis.2017.52
91. Rosin DL, Okusa MD. Dying cells and extracellular histones in AKI: beyond a NET effect? J Am Soc Nephrol. (2012) 23:1275–77. doi: 10.1681/ASN.2012060615
92. Tian X, Zhao L, Song X, Yan Y, Liu N, Li T, et al. HSP27 inhibits homocysteine-induced endothelial apoptosis by modulation of ROS production and mitochondrial caspase-dependent apoptotic pathway. Biomed Res Int. (2016) 2016:4847874. doi: 10.1155/2016/4847874
93. Aufricht C, Lu E, Thulin G, Kashgarian M, Siegel NJ, Van Why SK. ATP releases HSP-72 from protein aggregates after renal ischemia. Am J Physiol. (1998) 274:F268–74. doi: 10.1152/ajprenal.1998.274.2.F268
94. Morita K, Wakui H, Komatsuda A, Ohtani H, Miura AB, Itoh H, et al. Induction of heat-shock proteins HSP73 and HSP90 in rat kidneys after ischemia. Ren Fail. (1995) 17:405–19. doi: 10.3109/08860229509037605
95. Yokoo T, Kitamura M. IL-1beta depresses expression of the 70-kilodalton heat shock protein and sensitizes glomerular cells to oxidant-initiated apoptosis. J Immunol. (1997) 159:2886–92.
96. Komatsuda A, Wakui H, Imai H, Miura AB, Itoh H, Tashima Y. Expression of 90-kDa heat-shock protein within cellular crescents in human diseased kidneys. Nephrology. (1996) 2:87–91. doi: 10.1111/j.1440-1797.1996.tb00070.x
97. Ramirez-Sandoval JC, Barrera-Chimal J, Simancas PE, Correa-Rotter R, Bobadilla NA, Morales-Buenrostro LE. Tubular urinary biomarkers do not identify aetiology of acute kidney injury in kidney transplant recipients. Nephrology. (2014) 19:352–8. doi: 10.1111/nep.12240
98. El-Gamasy MA, El-Sadek AE, Fakhreldin AR, Kamel A, Elbehery EG. Heat shock protein 60 as a biomarker for acute kidney injury secondary to septic shock in pediatric patients, Egyptian multicenter experience. Saudi J Kidney Dis Transpl. (2018) 29:852–62. doi: 10.4103/1319-2442.239651
99. Xia C, Braunstein Z, Toomey AC, Zhong J, Rao X. S100 proteins as an important regulator of macrophage inflammation. Front Immunol. (2018) 8:1908. doi: 10.3389/fimmu.2017.01908
100. Sautin YY, Johnson RJ. Uric acid: the oxidant-antioxidant paradox. Nucleosides Nucleotides Nucleic Acids. (2008) 27:608–19. doi: 10.1080/15257770802138558
101. Mulay SR, Shi C, Ma X, Anders HJ. Novel insights into crystal-induced kidney injury. Kidney Dis. (2018) 4:49–57. doi: 10.1159/000487671
102. Ejaz AA, Johnson RJ, Shimada M, Mohandas R, Alquadan KF, Beaver TM, et al. The role of uric acid in acute kidney injury. Nephron. (2019) 142:275–83. doi: 10.1159/000499939
103. Quaye IK. Haptoglobin, inflammation and disease. Trans R Soc Trop Med Hyg. (2008) 102:735–42. doi: 10.1016/j.trstmh.2008.04.010
104. Maines MD, Mayer RD, Ewing JF, McCoubrey WK. Induction of kidney heme oxygenase-1 (HSP32) mRNA and protein by ischemia/reperfusion: possible role of heme as both promotor of tissue damage and regulator of HSP32. J Pharmacol Exp Ther. (1993) 264:457–62.
105. Nath KA, Grande JP, Croatt AJ, Likely S, Hebbel RP, Enright H. Intracellular targets in heme protein-induced renal injury. Kidney Int. (1998) 53:100–11. doi: 10.1046/j.1523-1755.1998.00731.x
106. Ralto KM, Parikh SM. Mitochondria in acute kidney injury. Semin Nephrol. (2016) 36:8–16. doi: 10.1016/j.semnephrol.2016.01.005
107. Tran M, Tam D, Bardia A, Bhasin M, Rowe GC, Kher A, et al. PGC-1α promotes recovery after acute kidney injury during systemic inflammation in mice. J Clin Invest. (2011) 121:4003–14. doi: 10.1172/JCI58662
108. Nakahira K, Hisata S, Choi AMK. The roles of mitochondrial damage-associated molecular patterns in diseases. Antioxid Redox Signal. (2015) 23:1329–50. doi: 10.1089/ars.2015.6407
109. Rodríguez-Nuevo A, Zorzano A. The sensing of mitochondrial DAMPs by non-immune cells. Cell Stress. (2019) 3:195–207. doi: 10.15698/cst2019.06.190
110. Martin JL, Gruszczyk AV, Beach TE, Murphy MP, Saeb-Parsy K. Mitochondrial mechanisms and therapeutics in ischaemia reperfusion injury. Pediatr Nephrol. (2019) 34:1167–74. doi: 10.1007/s00467-018-3984-5
111. Yan Y, Bai J, Zhou X, Tang J, Jiang C, Tolbert E, et al. P2X7 receptor inhibition protects against ischemic acute kidney injury in mice. Am J Physiol Cell Physiol. (2015) 308:C463–72. doi: 10.1152/ajpcell.00245.2014
112. Arulkumaran N, Sixma ML, Pollen S, Ceravola E, Jentho E, Prendecki M, et al. P2x7 receptor and sepsis-induced acute tubular injury. Intensive Care Med. (2012) 6:e13622. doi: 10.14814/phy2.13622
113. Rabadi M, Kim M, Li H, Han SJ, Choi Y, D'Agati V, et al. ATP induces PAD4 in renal proximal tubule cells via P2X7 receptor activation to exacerbate ischemic AKI. Am J Physiol Ren Physiol. (2018) 314:F293–305. doi: 10.1152/ajprenal.00364.2017
114. Han SJ, Lovaszi M, Kim M, D'Agati V, Haskó G, Lee HT. P2X4 receptor exacerbates ischemic AKI and induces renal proximal tubular NLRP3 inflammasome signaling. FASEB J. (2020) 34:5465–82. doi: 10.1096/fj.201903287R
115. Antonioli L, Pacher P, Vizi ES, Haskó G. CD39 and CD73 in immunity and inflammation. Trends Mol Med. (2013) 19:355–67. doi: 10.1016/j.molmed.2013.03.005
116. Blume C, Felix A, Shushakova N, Gueler F, Falk CS, Haller H, et al. Autoimmunity in CD73/Ecto-5′-nucleotidase deficient mice induces renal injury. PLoS ONE. (2012) 7:e37100. doi: 10.1371/journal.pone.0037100
117. Crikis S, Lu B, Murray-Segal LM, Selan C, Robson SC, D'Apice AJF, et al. Transgenic overexpression of CD39 protects against renal ischemia-reperfusion and transplant vascular injury. Am J Transplant. (2010) 10:2586–95. doi: 10.1111/j.1600-6143.2010.03257.x
118. Su L, Jiang X, Yang C, Zhang J, Chen B, Li Y, et al. Pannexin 1 mediates ferroptosis that contributes to renal ischemia/reperfusion injury. J Biol Chem. (2019) 294:19395–404. doi: 10.1074/jbc.RA119.010949
119. Jankowski J, Perry HM, Medina CB, Huang L, Yao J, Bajwa A, et al. Epithelial and endothelial pannexin1 channels mediate AKI. J Am Soc Nephrol. (2018) 29:1887–99. doi: 10.1681/ASN.2017121306
120. Schlame M, Greenberg ML. Biosynthesis, remodeling and turnover of mitochondrial cardiolipin. Biochim Biophys Acta Mol Cell Biol Lipids. (2017) 1862:3–7. doi: 10.1016/j.bbalip.2016.08.010
121. Lipowsky HH, Lescanic A. The effect of doxycycline on shedding of the glycocalyx due to reactive oxygen species. Microvasc Res. (2013) 90:80–85. doi: 10.1016/j.mvr.2013.07.004
122. Lygizos MI, Yang Y, Altmann CJ, Okamura K, Hernando AA, Perez MJ, et al. Heparanase mediates renal dysfunction during early sepsis in mice. Physiol Rep. (2013) 1:1–12. doi: 10.1002/phy2.153
123. Poluzzi C, Nastase MV, Zeng-Brouwers J, Roedig H, Hsieh LTH, Michaelis JB, et al. Biglycan evokes autophagy in macrophages via a novel CD44/Toll-like receptor 4 signaling axis in ischemia/reperfusion injury. Kidney Int. (2019) 95:540–62. doi: 10.1016/j.kint.2018.10.037
124. Ichimura T, Asseldonk EJPV, Humphreys BD, Gunaratnam L, Duffield JS, Bonventre J V. Kidney injury molecule-1 is a phosphatidylserine receptor that confers a phagocytic phenotype on epithelial cells. J Clin Invest. (2008) 118:1657–68. doi: 10.1172/JCI34487
125. Zhang Z, Cai CX. Kidney injury molecule-1 (KIM-1) mediates renal epithelial cell repair via ERK MAPK signaling pathway. Mol Cell Biochem. (2016) 416:109–16. doi: 10.1007/s11010-016-2700-7
126. Kistler AD, Mischak H, Poster D, Dakna M, Wüthrich RP, Serra AL. Identification of a unique urinary biomarker profile in patients with autosomal dominant polycystic kidney disease. Kidney Int. (2009) 76:89–96. doi: 10.1038/ki.2009.93
127. Ling XB, Sigdel TK, Lau K, Ying L, Lau I, Schilling J, et al. Integrative urinary peptidomics in renal transplantation identifies biomarkers for acute rejection. J Am Soc Nephrol. (2010) 21:646–53. doi: 10.1681/ASN.2009080876
128. Säemann MD, Weichhart T, Zeyda M, Staffler G, Schunn M, Stuhlmeier KM, et al. Tamm-Horsfall glycoprotein links innate immune cell activation with adaptive immunity via a Toll-like receptor-4–dependent mechanism. J Clin Invest. (2005) 115:468–75. doi: 10.1172/JCI200522720
129. Sinikka Prajczer, Ursula Heidenreich, Walter Pfaller, Peter Kotanko, Karl Lhotta PJ. Evidence for a role of uromodulin in chronic kidney disease progression | request PDF. Nephrol Dial Transplant. (2010) 25:1896–903. doi: 10.1093/ndt/gfp748
130. Garimella PS, Jaber BL, Tighiouart H, Liangos O, Bennett MR, Devarajan P, et al. Association of preoperative urinary uromodulin with aki after cardiac surgery. Clin J Am Soc Nephrol. (2017) 12:10–8. doi: 10.2215/CJN.02520316
131. Kú snierz-Cabala B, Gala-Bładzí nska A, Mazur-Laskowska M, Dumnicka P, Sporek M, Matuszyk A, et al. Serum uromodulin levels in prediction of acute kidney injury in the early phase of acute pancreatitis. Molecules. (2017) 22:988. doi: 10.3390/molecules22060988
132. Bennett MR, Pyles O, Ma Q, Devarajan P. Preoperative levels of urinary uromodulin predict acute kidney injury after pediatric cardiopulmonary bypass surgery. Pediatr Nephrol. (2018) 33:521–6. doi: 10.1007/s00467-017-3823-0
133. Lane BR, Babitz SK, Vlasakova K, Wong A, Noyes SL, Boshoven W, et al. Evaluation of urinary renal biomarkers for early prediction of acute kidney injury following partial nephrectomy: a feasibility study. Eur Urol Focus. (2018) S2405-4569:30318–3. doi: 10.1016/j.euf.2018.10.017
134. Yang D, Han Z, Oppenheim JJ. Alarmins and immunity. Immunol Rev. (2017) 280:41–56. doi: 10.1111/imr.12577
135. Schwaderer AL, Wang H, Kim SH, Kline JM, Liang D, Brophy PD, et al. Polymorphisms in α-defensin-encoding DEFA1A3 associate with urinary tract infection risk in children with vesicoureteral reflux. J Am Soc Nephrol. (2016) 27:3175–86. doi: 10.1681/ASN.2015060700
136. McDermott AM. Defensins and other antimicrobial peptides at the ocular surface. Ocul Surf. (2004) 2:229–47. doi: 10.1016/S1542-0124(12)70111-8
137. Donovan KL, Topley N. What are renal defensins defending? Nephron Exp Nephrol. (2003) 93:e125–8. doi: 10.1159/000070235
138. Park SW, Chen SW, Kim M, Brown KM, Kolls JK, D'Agati VD, et al. Cytokines induce small intestine and liver injury after renal ischemia or nephrectomy. Lab Investig. (2011) 91:63–84. doi: 10.1038/labinvest.2010.151
139. Hilchie AL, Wuerth K, Hancock REW. Immune modulation by multifaceted cationic host defense (antimicrobial) peptides. Nat Chem Biol. (2013) 9:761–8. doi: 10.1038/nchembio.1393
140. Hancock REW, Haney EF, Gill EE. The immunology of host defence peptides: beyond antimicrobial activity. Nat Rev Immunol. (2016) 16:321–34. doi: 10.1038/nri.2016.29
141. McHugh BJ, Wang R, Li HN, Beaumont PE, Kells R, Stevens H, et al. Cathelicidin is a “fire alarm”, generating protective NLRP3-dependent airway epithelial cell inflammatory responses during infection with Pseudomonas aeruginosa. PLoS Pathog. (2019) 15:e1007694. doi: 10.1371/journal.ppat.1007694
142. Khokha R, Murthy A, Weiss A. Metalloproteinases and their natural inhibitors in inflammation and immunity. Nat Rev Immunol. (2013) 13:649–65. doi: 10.1038/nri3499
143. Ferry RJ, Katz LEL, Grimberg A, Cohen P, Weinzimer SA. Cellular actions of insulin-like growth factor binding proteins. Horm Metab Res. (1999) 31:192–202. doi: 10.1055/s-2007-978719
144. Zhang Y, Zhou B. Functions of thymic stromal lymphopoietin in immunity and disease. Immunol Res. (2012) 52:211–23. doi: 10.1007/s12026-012-8264-z
145. Krensky AM, Clayberger C. Biology and clinical relevance of granulysin: review article. Tissue Antigens. (2009) 73:193–8. doi: 10.1111/j.1399-0039.2008.01218.x
146. Li S, Nagothu KK, Desai V, Lee T, Branham W, Moland C, et al. Transgenic expression of proximal tubule peroxisome proliferator-activated receptor-α in mice confers protection during acute kidney injury. Kidney Int. (2009) 76:1049–62. doi: 10.1038/ki.2009.330
147. Fontecha-barriuso M, Martin-sanchez D, Martinez-moreno JM, Monsalve M, Ramos AM, Sanchez-niño MD, et al. The role of PGC-1α and mitochondrial biogenesis in kidney diseases. Biomolecules. (2020) 10:347. doi: 10.3390/biom10020347
148. Mitchell T, Rotaru D, Saba H, Smith RAJ, Murphy MP, MacMillan-Crow LA. The mitochondria-targeted antioxidant mitoquinone protects against cold storage injury of renal tubular cells and rat kidneys. J Pharmacol Exp Ther. (2011) 336:682–92. doi: 10.1124/jpet.110.176743
149. Piot C, Croisille P, Staat P, Thibault H, Rioufol G, Mewton N, et al. Effect of cyclosporine on reperfusion injury in acute myocardial infarction. N Engl J Med. (2008) 359:473–81. doi: 10.1056/NEJMoa071142
150. Howell GM, Gomez H, Collage RD, Loughran P, Zhang X, Escobar DA, et al. Augmenting autophagy to treat acute kidney injury during endotoxemia in mice. PLoS ONE. (2013) 8:e69520. doi: 10.1371/journal.pone.0069520
151. Jesinkey SR, Funk JA, Stallons LJ, Wills LP, Megyesi JK, Beeson CC, et al. Formoterol restores mitochondrial and renal function after ischemia-reperfusion injury. J Am Soc Nephrol. (2014) 25:1157–62. doi: 10.1681/ASN.2013090952
152. Ponnusamy M, Ma L, Gong R, Pang M, Chin YE, Zhuang S. P2X 7 receptors mediate deleterious renal epithelial-fibroblast cross talk. Am J Physiol Physiol. (2011) 300:F62–70. doi: 10.1152/ajprenal.00473.2010
153. Beumer C, Wulferink M, Raaben W, Fiechter D, Brands R, Seinen W. Calf intestinal alkaline phosphatase, a novel therapeutic drug for Lipopolysaccharide (LPS)-mediated diseases, attenuates LPS toxicity in mice and piglets. J Pharmacol Exp Ther. (2003) 307:737–44. doi: 10.1124/jpet.103.056606
154. Pettengill M, Matute JD, Tresenriter M, Hibbert J, Burgner D, Richmond P, et al. Human alkaline phosphatase dephosphorylates microbial products and is elevated in preterm neonates with a history of late-onset sepsis. (2017) 12:e0175936. doi: 10.1371/journal.pone.0175936
155. Peters E, Masereeuw R, Pickkers P. The potential of alkaline phosphatase as a treatment for sepsis-associated acute kidney injury. Nephron Clin Pract. (2014) 127:144–8. doi: 10.1159/000363256
156. Kim JY, Kim M, Ham A, Brown KM, Greene RW, D'Agati VD, et al. IL-11 is required for A1 adenosine receptor-mediated protection against ischemic AKI. J Am Soc Nephrol. (2013) 24:1558–70. doi: 10.1681/ASN.2013010114
157. Li L, Huang L, Ye H, Song SP, Bajwa A, Lee SJ, et al. Dendritic cells tolerized with adenosine A2AR agonist attenuate acute kidney injury. J Clin Invest. (2012) 122:3931–42. doi: 10.1172/JCI63170
158. Kinsey GR, Huang L, Jaworska K, Khutsishvili K, Becker DA, Ye H, et al. Autocrine adenosine signaling promotes regulatory T cell-mediated renal protection. J Am Soc Nephrol. (2012) 23:1528–37. doi: 10.1681/ASN.2012010070
159. Cao W, Yuan Y, Liu X, Li Q, An X, Huang Z, et al. Adenosine kinase inhibition protects against cisplatin-induced nephrotoxicity. Am J Physiol Ren Physiol. (2019) 317:F107–15. doi: 10.1152/ajprenal.00385.2018
160. Nakagawa S, Omura T, Yonezawa A, Yano I, Nakagawa T, Matsubara K. Extracellular nucleotides from dying cells act as molecular signals to promote wound repair in renal tubular injury. Am J Physiol Physiol. (2014) 307:F1404–11. doi: 10.1152/ajprenal.00196.2014
161. Nakagawa T, Kakizoe Y, Iwata Y, Miyasato Y, Mizumoto T, Adachi M, et al. Doxycycline attenuates cisplatin-induced acute kidney injury through pleiotropic effects. Am J Physiol Ren Physiol. (2018) 315:F1347–57. doi: 10.1152/ajprenal.00648.2017
162. Cortes AL, Gonsalez SR, Rioja LS, Oliveira SSC, Santos ALS, Prieto MC, et al. Protective outcomes of low-dose doxycycline on renal function of Wistar rats subjected to acute ischemia/reperfusion injury. Biochim Biophys Acta Mol Basis Dis. (2018) 1864:102–14. doi: 10.1016/j.bbadis.2017.10.005
163. Seno H, Sawada M, Fukuzawa H, Morita-Fujisawa Y, Takaishi S, Hiai H, et al. Involvement of tumor necrosis factor alpha in intestinal epithelial cell proliferation following Paneth cell destruction. Scand J Gastroenterol. (2002) 37:154–60. doi: 10.1080/003655202753416803
164. Sawada M, Takahashi K, Sawada S, Midorikawa O. Selective killing of Paneth cells by intravenous administration of dithizone in rats. Int J Exp Pathol. (1991) 72:407–21.
165. Salzman NH, Hung K, Haribhai D, Chu H, Karlsson-Sjöberg J, Amir E, et al. Enteric defensins are essential regulators of intestinal microbial ecology. Nat Immunol. (2010) 11:76–83. doi: 10.1038/ni.1825
166. Turnquist HR, Zhao Z, Rosborough BR, Liu Q, Castellaneta A, Isse K, et al. IL-33 expands suppressive CD11b + Gr-1 int and regulatory T cells, including ST2L + Foxp3 + cells, and mediates regulatory T cell-dependent promotion of cardiac allograft survival. J Immunol. (2011) 187:4598–610. doi: 10.4049/jimmunol.1100519
167. Monticelli LA, Osborne LC, Noti M, Tran SV, Zaiss DMW, Artis D. IL-33 promotes an innate immune pathway of intestinal tissue protection dependent on amphiregulin–EGFR interactions. Proc Natl Acad Sci USA. (2015) 112:10762–7. doi: 10.1073/pnas.1509070112
168. Sharma R, Sharma PR, Kim Y-C, Leitinger N, Lee JK, Fu SM, et al. IL-2-controlled expression of multiple T cell trafficking genes and Th2 cytokines in the regulatory T cell-deficient scurfy mice: implication to multiorgan inflammation and control of skin and lung inflammation. J Immunol. (2011) 186:1268–78. doi: 10.4049/jimmunol.1002677
169. Stremska ME, Jose S, Sabapathy V, Huang L, Bajwa A, Kinsey GR, et al. IL233, a novel IL-2 and IL-33 hybrid cytokine, ameliorates renal injury. J Am Soc Nephrol. (2017) 28:2681–93. doi: 10.1681/ASN.2016121272
170. Sabapathy V, Cheru NT, Corey R, Mohammad S, Sharma R. A novel hybrid cytokine IL233 mediates regeneration following doxorubicin-induced nephrotoxic injury. Sci Rep. (2019) 9:3215. doi: 10.1038/s41598-019-39886-9
171. Cao Q, Wang Y, Niu Z, Wang C, Wang R, Zhang Z, et al. Potentiating tissue-resident type 2 innate lymphoid cells by il-33 to prevent renal ischemia-reperfusion injury. J Am Soc Nephrol. (2018) 29:ASN.2017070774. doi: 10.1681/ASN.2017070774
172. Cameron GJM, Cautivo KM, Loering S, Jiang SH, Deshpande A V, Foster PS, et al. Group 2 innate lymphoid cells are redundant in experimental renal ischemia-reperfusion injury. Front Immunol. (2019) 10:826. doi: 10.3389/fimmu.2019.00826
173. Wu H, Steenstra R, De Boer ECS, Zhao CY, Ma J, Van Der Stelt JM, et al. Preconditioning with recombinant high-mobility group box 1 protein protects the kidney against ischemia-reperfusion injury in mice. Kidney Int. (2014) 85:824–32. doi: 10.1038/ki.2013.475
174. Kim M, Park SW, Kim M, Chen SWC, Gerthoffer WT, D'Agati VD, et al. Selective renal overexpression of human heat shock protein 27 reduces renal ischemia-reperfusion injury in mice. Am J Physiol Ren Physiol. (2010) 299:F347–58. doi: 10.1152/ajprenal.00194.2010
175. Kubota K, Egi M, Mizobuchi S. Haptoglobin administration in cardiovascular surgery patients. Anesth Analg. (2017) 124:1771–76. doi: 10.1213/ANE.0000000000002093
176. Morimoto K, Ohta K, Yachie A, Yang Y, Shimizu M, Goto C, et al. Cytoprotective role of heme oxygenase (HO)-1 in human kidney with various renal diseases. Kidney Int. (2001) 60:1858–66. doi: 10.1046/j.1523-1755.2001.01000.x
177. Fervenza FC, Croatt AJ, Bittar CM, Rosenthal DW, Lager DJ, Leung N, et al. Induction of heme oxygenase-1 and ferritin in the kidney in warm antibody hemolytic anemia. Am J Kidney Dis. (2008) 52:972–7. doi: 10.1053/j.ajkd.2008.07.012
178. Wei Q, Hill WD, Su Y, Huang S, Dong Z. Heme oxygenase-1 induction contributes to renoprotection by G-CSF during rhabdomyolysis-associated acute kidney injury. Am J Physiol Ren Physiol. (2011) 301:F162. doi: 10.1152/ajprenal.00438.2010
179. Cheng CF, Lian WS, Chen SH, Lai PF, Li HF, Lan YF, et al. Protective effects of adiponectin against renal ischemia-reperfusion injury via prostacyclin-PPARα-Heme oxygenase-1 signaling pathway. J Cell Physiol. (2012) 227:239–49. doi: 10.1002/jcp.22726
180. Walker VJ, Agarwal A. Targeting iron homeostasis in acute kidney injury. Semin Nephrol. (2016) 36:62–70. doi: 10.1016/j.semnephrol.2016.01.003
181. Scindia Y, Dey P, Thirunagari A, Liping H, Rosin DL, Floris M, et al. Hepcidin mitigates renal ischemia-reperfusion injury by modulating systemic iron homeostasis. J Am Soc Nephrol. (2015) 26:2800–14. doi: 10.1681/ASN.2014101037
182. Young GH, Huang TM, Wu CH, Lai CF, Hou CC, Peng KY, et al. Hemojuvelin modulates iron stress during acute kidney injury: improved by furin inhibitor. Antioxidants Redox Signal. (2014) 20:1181–94. doi: 10.1089/ars.2013.5366
183. Hu JB, Li SJ, Kang XQ, Qi J, Wu JH, Wang XJ, et al. CD44-targeted hyaluronic acid-curcumin prodrug protects renal tubular epithelial cell survival from oxidative stress damage. Carbohydr Polym. (2018) 193:268–80. doi: 10.1016/j.carbpol.2018.04.011
184. Lee EJ, Kim HS. The anti-inflammatory role of tissue inhibitor of metalloproteinase-2 in lipopolysaccharide-stimulated microglia. J Neuroinflammation. (2014) 11:116. doi: 10.1186/1742-2094-11-116
185. Holloway JL. Alerting stem cells to regenerate. Sci Transl Med. (2018) 10:eaat8523. doi: 10.1126/scitranslmed.aat8523
186. Joseph J, Dyson M. The effect of abdominal wounding on the rate of tissue regeneration. Experientia. (1970) 26:66–7. doi: 10.1007/BF01900396
187. Davis TA, Longcor JD, Hicok KC, Lennon GG. Prior injury accelerates subsequent wound closure in a mouse model of regeneration. Cell Tissue Res. (2005) 320:417–26. doi: 10.1007/s00441-005-1107-7
188. Lee G, Santo AIE, Zwingenberger S, Cai L, Vogl T, Feldmann M, et al. Fully reduced HMGB1 accelerates the regeneration of multiple tissues by transitioning stem cells to GAlert. Proc Natl Acad Sci USA. (2018) 115:E4463–72. doi: 10.1073/pnas.1802893115
189. Zarbock A, Kellum JA. Remote ischemic preconditioning and protection of the kidney-a novel therapeutic option. Crit Care Med. (2016) 44:607–16. doi: 10.1097/CCM.0000000000001381
190. Bidmon B, Endemann M, Müller T, Arbeiter K, Herkner K, Aufricht C. Heat shock protein-70 repairs proximal tubule structure after renal ischemia. Kidney Int. (2000) 58:2400–7. doi: 10.1046/j.1523-1755.2000.00423.x
191. Pockley AG. Heat shock proteins as regulators of the immune response. Lancet. (2003) 362:469–76. doi: 10.1016/S0140-6736(03)14075-5
192. de Kleer IM, Kamphuis SM, Rijkers GT, Scholtens L, Gordon G, de Jager W, et al. The spontaneous remission of juvenile idiopathic arthritis is characterized by CD30+ T cells directed to human heat-shock protein 60 capable of producing the regulatory cytokine interleukin-10. Arthritis Rheum. (2003) 48:2001–10. doi: 10.1002/art.11174
193. Kim MG, Jung Cho E, Won Lee J, Sook Ko Y, Young Lee H, Jo SK, et al. The heat-shock protein-70-induced renoprotective effect is partially mediated by CD4+ CD25+ Foxp3+ regulatory T cells in ischemia/reperfusion-induced acute kidney injury. Kidney Int. (2014) 85:62–71. doi: 10.1038/ki.2013.277
194. Prakken BJ, Roord S, Ronaghy A, Wauben M, Albani S, Van Eden W. Heat shock protein 60 and adjuvant arthritis: a model for T cell regulation in human arthritis. Springer Semin Immunopathol. (2003) 25:47–63. doi: 10.1007/s00281-003-0128-7
195. Gandolfo MT, Jang HR, Bagnasco SM, Ko G-J, Agreda P, Satpute SR, et al. Foxp3+ regulatory T cells participate in repair of ischemic acute kidney injury. Kidney Int. (2009) 76:717–29. doi: 10.1038/ki.2009.259
196. Sabapathy V, Stremska ME, Mohammad S, Corey RL, Sharma PR, Sharma R. Novel immunomodulatory cytokine regulates inflammation, diabetes, and obesity to protect from diabetic nephropathy. Front Pharmacol. (2019) 10:572. doi: 10.3389/fphar.2019.00572
Keywords: alarmins, AKI, inflammation, regeneration, IL-33, T-regulatory cells, Cytokines, DAMP
Citation: Sabapathy V, Venkatadri R, Dogan M and Sharma R (2020) The Yin and Yang of Alarmins in Regulation of Acute Kidney Injury. Front. Med. 7:441. doi: 10.3389/fmed.2020.00441
Received: 23 April 2020; Accepted: 06 July 2020;
Published: 21 August 2020.
Edited by:
Giuseppe Castellano, University of Bari Aldo Moro, ItalyReviewed by:
Hee-Seong Jang, University of Nebraska Medical Center, United StatesSergey Brodsky, Ohio State University Hospital, United States
Copyright © 2020 Sabapathy, Venkatadri, Dogan and Sharma. This is an open-access article distributed under the terms of the Creative Commons Attribution License (CC BY). The use, distribution or reproduction in other forums is permitted, provided the original author(s) and the copyright owner(s) are credited and that the original publication in this journal is cited, in accordance with accepted academic practice. No use, distribution or reproduction is permitted which does not comply with these terms.
*Correspondence: Rahul Sharma, cnMzd25AdmlyZ2luaWEuZWR1