- Institute of Vegetative Physiology, Charité–Universitätsmedizin Berlin, Corporate Member of Freie Universität Berlin, Humboldt-Universität zu Berlin, Berlin, Germany
Hypertension is ranked as the third cause of disability-adjusted life-years. The percentage of the population suffering from hypertension will continue to increase over the next years. Renovascular disease is one of the most common causes of secondary hypertension. Vascular changes seen in hypertension are partially based on dysfunctional calcium signaling. This signaling can be studied using calcium indicators (loading dyes and genetically encoded calcium indicators; GECIs). Most progress in development has been seen in GECIs, which are used in an increasing number of publications concerning calcium signaling in vasculature and the kidney. The use of transgenic mouse models expressing GECIs will facilitate new possibilities to study dysfunctional calcium signaling in a cell type-specific manner, thus helping to identify more specific targets for treatment of (renal) hypertension.
Introduction
Hypertension, a disease with high incidence and a leading risk factor of mortality, is ranked as the third cause of disability-adjusted life-years (1). In particular, raised blood pressure is an important risk factor for cardiovascular diseases and chronic kidney disease. Worldwide, the estimated total number of adults with hypertension in 2000 was 972 million (26.4% of the adult population) (2). The number of adults with raised blood pressure increased to 1.13 billion in 2015, with the increase occurring largely in low-income and middle-income countries (3). The number of adults with hypertension in 2,025 is predicted to increase to a total of 1.56 billion (1.54–1.58 billion) (2). The most common causes of secondary, non-essential hypertension are renovascular disease, intrinsic renal disease, and primary hyperaldosteronism (4). These data show that hypertension is a global burden.
Calcium is one of the most important and multi-functional second messengers in cell biology, not only controlling contraction of the striated (5) and vascular smooth muscle, but also regulating cellular processes such as growth, proliferation, transcription, exocytosis, and apoptosis (6). Additionally, regulation of thick myofilament Ca2+ sensitivity, cytosolic Ca2+ induces conformational changes of thin filaments, which together determine actin-myosin and myocyte relaxation or constriction (7). Vascular smooth muscle cell (VSMC) contraction is initiated by an increase in the global intracellular calcium ([Ca2+]i) concentration, which is caused by an opening of voltage-gated calcium channels, particularly L-type CaV1.2 channels (8). Importantly, the VSMCs' phenotype can switch in cardiovascular disease. For example, de-differentiation of the VSMCs is associated with a shift of the expression of Ca2+ channels from voltage-gated to voltage-insensitive Ca2+ channels (9). In addition, Ca2+ entry in non-contractile (dedifferentiated) VSMCs occurs predominantly via store-operated Ca2+ entry (SOCE) and receptor-operated Ca2+ entry (ROCE) pathways. These changes may have physiological importance for normal smooth muscle function and may influence VSMC behavior under pathophysiological conditions (9). With age, reactivity of small arteries is lowered. This is followed by alterations of arterial stiffness, arterial wall thickening, and a reduced myogenic responsiveness, thus increasing total peripheral resistance (10, 11). Calcium antagonists (e.g., L-type channel inhibitors) are often used as anti-hypertensive drugs. Since the reasons for dedifferentiation of VSMCs are poorly understood (12), it is important to identify calcium signaling pathways in VSMCs in hypertension and cardiovascular disease and determine their functions, which may ultimately lead to new drug targets.
Calcium Indicators: Loading Dyes, Fusion Proteins, and Genetically Modified GECIs
Calcium imaging of living tissue has turned out to be a useful tool for the investigation of different intracellular calcium signals. Fluorescent free calcium-binding dyes such as Fura-2 enabled a first visualization of intracellular calcium upon loading of the tissue (13). Fura-2 is still one of the most frequently used ratiometric Ca2+ indicators. It is excited at two different wavelengths. The Ca2+ unbound form of Fura-2 is excited at 380 nm and the Ca2+ bound form at 340 nm. The emitted light is measured at around 510 nm (14). Thus, the intensity of the emitted fluorescence light changes depending on the calcium ion concentration, while a high spatial resolution can be reached. Although low temporal resolution can be a problem for recording of fast Ca2+ transients, such as Ca2+ sparks in VSMCs (14, 15), a major disadvantage of loading dyes such as Fura-2 is a possible uneven tissue distribution of the dye as well as loading of cell structures not intended for investigation (16).
Genetically encoded Ca2+ indicators (GECIs) show a huge progress for imaging to solve poor selectivity and disadvantages of loading dyes into the cytosol before measurement (Table 2). The first protein-based Ca2+ indicator was photoprotein aequorin, purified from jellyfish Aequorea Victoria, injected into cells in the early 1970s (17). After cloning of its cDNA, recombinant aequorin became the most frequently used probe to measure intracellular Ca2+. Another important step was the advancement of a green fluorescent protein (GFP), which enabled the investigation of the spatio-temporal distribution of proteins in living cells, through the formation of fused protein-GFP structures. This coupling meant a co-expression of GFP upon target protein expression, thus marking the protein via fluorescence (18–21) (Table 1). GECIs are subject to photobleaching after extended excitation, although usually less than loading dyes (29). Thus, complex photobleaching curves have to be considered when analyzing obtained data. The probe insensitivity to Mg2+ is an important issue, because changes of Mg2+ concentration can trigger cell activation. Inaccurate intracellular location is also another issue to be solved using GECIs. A kind of larger dynamic range, robust photonic and thermal stability Ca2+ probe is needed. (30) Furthermore, oligomerization is another adverse property of GFP. Therefore, mutagenesis approaches are needed to recover the functional expression of monomeric forms of GFP (31) (Tables 2, 3).
GFP is used as scaffolding for the most recent fluorescent calcium indicators, namely genetically encoded calcium indicators (GECIs) like GCaMP (26). In those proteins, circularly permuted forms of GFP are fused to calmodulin (CaM) and the M13 domain of the myosin light chain kinase (MLCK). The latter is able to bind CaM. If calcium ions are present, GCaMP changes its conformation due to calcium binding to CaM (Figure 1). This leads to a bright fluorescence through a rapid deprotonation of the chromophore (26).
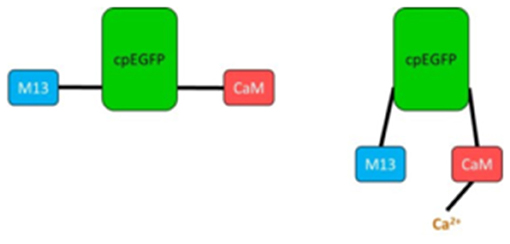
Figure 1. Simplified scheme of GCaMP unbound (Left) and bound to Ca2+ (Right). M13, fragment of myosin light chain kinase; cpEGFP, circularly permuted enhanced GFP; CaM, calmodulin.
GECIs can be used not only for Ca2+ imaging in the cytosol but also in subcellular compartments, i.e., in a wide spectrum of organelles. Among them, the GCaMP6f probe targeted to mitochondria (4mtGCaMP) has been recently developed to measure mitochondrial calcium levels (39). Other protocols of using GECIs have been developed for Ca2+ imaging in the nucleus and endoplasmic reticulum (40, 41). Importantly, the fluorescent indicators' brightness could be problematic for Ca2+ imaging in organelles because of the acidic environment which can affect fluorescence; therefore, specific tasks, e.g., “chameleon” type indicators, have been developed for target locations in different organelles to detect Ca2+ release (42). The fluorescent indicators mentioned above emit green fluorescence. Notably, there are also modified variants of GFP using different fluorescence spectra. Known fluorescence examples include RFP (red fluorescence) (43), YFP (yellow fluorescence) (44, 45), or CFP (cyan fluorescence) (46, 47). The modifications also provide the basis for GECIs as RCaMP (red fluorescence) (48), YCaMP (yellow fluorescence) (48), or CyCaMP (cyan fluorescence) (48). Different color channels allows for the imaging of tissues already expressing GFP, as well as the reduction of autofluorescence compared to GCaMP or loading dyes.
Genetically encoded calcium indicators (GECIs) can be divided into three different classes (Table 1) (49), two of them have just been described. Class 3 indicators (“chameleon”-type sensors) contain two fluorescent proteins and are applied to measurements involving fluorescent resonance energy transfer (FRET).
Vascular Expression of GCaMP Indicators
One huge advantage of GCaMP is the possibility of cell type-specific expression. It can be coupled to a promoter only expressed in the cell type of interest, so activation of this promoter leads to co-expression of GCaMP. Many studies involving GCaMP have been done in neuronal tissue (50–52), but vascular applications are possible, for example, using mice expressing GCaMP only in acta 2-positive cells (SMC-specific fluorescence), or in connexin 40 (Cx40)—positive cells (endothelial-specific fluorescence) (53–57). Not only can the expression of the protein be detected (as with GFP), but changes in fluorescence intensity reflecting changes in the calcium concentration can also be detected. If GCaMP is co-expressed with another fluorescent protein using other wavelength spectra, e.g., red fluorescent mCherry, a ratio of those two fluorescent proteins can be used for quantification of the intracellular calcium concentration. But expression levels of transgenes show considerable animal-to-animal variation, complicating the analysis of imaging results, and so linearizing the imaging measurement and background in high pixels is needed (58). As GCaMP sensors are stable at mammalian body temperatures, they can also be used for in vivo recordings of calcium signaling. GCaMP molecules have been modified and improved on since their first development, allowing higher spatio-temporal resolution, higher sensitivity, and more rapid off kinetics. GCaMP expression even allows calcium imaging of subcellular structures such as a nucleus, endoplasmic reticulum, and mitochondria (57). There is also a review available specifically on the application of GCaMP in cardiomyocytes (59).
GCaMP Indicators in Kidney Slices
In the vasculature, the above-mentioned Cx40 serves as an endothelial-specific marker protein, enabling cell type-specific calcium imaging. In the kidney, Cx40 was found to be abundantly expressed in specialized SMCs of the juxtaglomerular apparatus (JGA), namely renin-producing granular cells (60). The cells contribute to the tone of afferent arteriole and control of renin synthesis and release. Both processes rely on calcium signals (61, 62), which are positively correlated with renin secretion (63) without the involvement of voltage-gated Ca2+ channels (64). Interestingly, these cells contain, in addition to the secretory granules, contractile proteins, which are arranged in a sublemmal network. A paradoxical (inhibitory) role of intracellular calcium in renin secretion could be explained by an increased tone of this sublemmal network, which might impair the pre-exocytotic access of renin granules to the cell membrane (65).
Cx40, a gap junction protein, coordinates propagating signals between individual cells, thus Cx40-positive cells expressing a GCaMP sensor are valuable for studying propagating calcium signals in connected cells and tissues, respectively. Propagating calcium transients can be observed using an in-situ kidney slice model of Cx40-GCaMP mice, as shown in Figure 2. The slices already show spontaneous intracellular calcium transients without any treatment. Upon treatment with angiotensin II (AngII), the Ca2+ transients are elevated (Figure 2). Characterizing the spontaneous signals and comparing them to AngII-induced transients might be of great interest for (1) studying the control of afferent arteriole tone and renin release and (2) explore potential coupling of the signals regulating both processes.
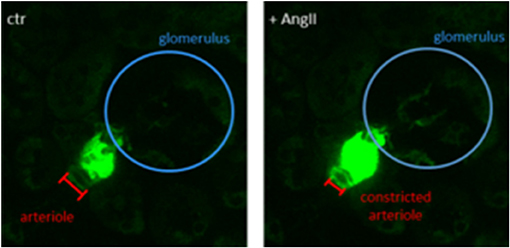
Figure 2. Single frame of a Cx40-GCaMP kidney slice recording. Left: Spontaneous calcium transients in Cx40-positive renin-producing granular cells (green). Right: Increased calcium signal after treatment with angiotensin II (AngII, 100 nM). Afferent arteriole is constricted upon AngII treatment.
GCaMP sensors have already been used in the kidney to study calcium signals in podocytes. Pathological changes of the glomerular filtration barrier have been linked to elevated intracellular calcium concentrations in podocytes (66). Signals could be measured in vivo using GCaMP sensors in podocin-positive cells (Pod-GCaMP3), providing podocyte-specific fluorescence (67).
Summary
The ongoing development of genetically modified calcium indicators provides great opportunities for studying calcium signaling in specific cell types and organs linked to the development of hypertension, e.g., in vasculature and kidneys. The improvement of the sensors allows a higher spatio-temporal resolution and a higher sensitivity for qualitative as well as quantitative measurements. Different excitation wavelengths enable simultaneous recordings of calcium signals in different colors, and thus a differentiation of parallel signals in different locations (e.g., SMCs and ECs).
Mice expressing GECIs in a cell type-specific manner can be subject to hypertension-inducing treatments (administration of AngII, or L-NAME), allowing exploration of calcium signaling changes in vasculature and organs such as the kidneys, in comparison to healthy control animals. The effects of different drugs influencing intracellular calcium signaling can be studied in vivo as well as in situ and can help in finding new therapeutic targets to reverse dysfunctional calcium signaling under hypertensive conditions in vasculature and kidneys. Thus, animal models expressing GCaMP sensors will play an important role in the future of renal hypertension research.
Author Contributions
All authors listed have made a substantial, direct and intellectual contribution to the work, and approved it for publication.
Funding
This work is supported by the Deutsche Forschungsgemeinschaft (DFG). CZ is funded by a research fellowship from Guiyang Sunshine Hospital; Guiyang, China. We acknowledge support from the Open Access Publication Fund of Charité–Universitätsmedizin Berlin.
Conflict of Interest Statement
The authors declare that the research was conducted in the absence of any commercial or financial relationships that could be construed as a potential conflict of interest.
The handling Editor declared shared affiliations, though no other collaboration on this topic or techniques, with both of the authors.
References
1. Ezzati M, Lopez AD, Rodgers A, Vander Hoorn S, Murray CJ. Selected major risk factors and global and regional burden of disease. Lancet. (2002) 360:1347–60. doi: 10.1016/S0140-6736(02)11403-6
2. Kearney PM, Whelton M, Reynolds K, Muntner P, Whelton PK, He J. Global burden of hypertension: analysis of worldwide data. Lancet. (2005) 365:217–23. doi: 10.1016/S0140-6736(05)70151-3
3. NCD Risk Factor Collaboration. Worldwide trends in blood pressure from 1975 to 2015: a pooled analysis of 1479 population-based measurement studies with 19.1 million participants. Lancet. (2017) 389:37–55. doi: 10.1016/S0140-6736(16)31919-5
4. Pullalarevu R, Akbar G, Teehan G. Secondary hypertension, issues in diagnosis and treatment. Primary care. (2014) 41:749–64. doi: 10.1016/j.pop.2014.08.001
5. Schneider MF. Control of calcium release in functioning skeletal muscle fibers. Annu Rev Physiol. (1994) 56:463–84. doi: 10.1146/annurev.ph.56.030194.002335
6. Berridge MJ, Bootman MD, Roderick HL. Calcium signalling: dynamics, homeostasis and remodelling. Nat Rev Mol Cell Biol. (2003) 4:517–29. doi: 10.1057/9780230501348_10
7. Bers DM. Cardiac excitation-contraction coupling. Nature. (2002) 415:198–205. doi: 10.1038/415198a
8. Knot HJ, Nelson MT. Regulation of arterial diameter and wall [Ca2+] in cerebral arteries of rat by membrane potential and intravascular pressure. J Physiol. (1998) 508(Pt 1):199–209. doi: 10.1111/j.1469-7793.1998.199br.x
9. Gollasch M, Haase H, Ried C, Lindschau C, Morano I, Luft FC, et al. L-type calcium channel expression depends on the differentiated state of vascular smooth muscle cells. FASEB J. (1998) 12:593–601. doi: 10.1096/fasebj.12.7.593
10. Sehgel NL, Sun Z, Hong Z, Hunter WC, Hill MA, Vatner DE, et al. Augmented vascular smooth muscle cell stiffness and adhesion when hypertension is superimposed on aging. Hypertension. (2015) 65:370–7. doi: 10.1161/HYPERTENSIONAHA.114.04456
11. Sun Z Aging, arterial stiffness, and hypertension. Hypertension. (2015) 65:252–6. doi: 10.1161/HYPERTENSIONAHA.114.03617
12. Matchkov VV, Kudryavtseva O, Aalkjaer C. Intracellular Ca2+ signalling and phenotype of vascular smooth muscle cells. Basic Clin Pharmacol Toxicol. (2011) 110:42–8. doi: 10.1111/j.1742-7843.2011.00818.x
13. Grynkiewicz G, Poenie M, Tsien RY A new generation of Ca2+ indicators with greatly improved fluorescence properties. J Biol Chem. (1985) 260:3440–50.
14. So PTC, French T, Yu WM, Berland KM, Dong CY, Gratton E. Time-resolved fIuorescence microscopy using two-photon excitation. Bioimaging. (1995) 3:49–63.
15. Nelson MT, Cheng H, Rubart M, Santana LF, Bonev AD, Knot HJ, et al. Relaxation of arterial smooth muscle by calcium sparks. Science. (1995) 270:633–7. doi: 10.1126/science.270.5236.633
16. Paredes RM, Etzler JC, Watts LT, Zheng W, Lechleiter JD. Chemical calcium indicators. Methods. (2008) 46:143–51. doi: 10.1016/j.ymeth.2008.09.025
18. Arun KH, Kaul CL, Ramarao P. Green fluorescent proteins in receptor research: an emerging tool for drug discovery. J Pharmacol Toxicol Methods. (2005) 51:1–23. doi: 10.1016/j.vascn.2004.07.006
19. Jugder BE, Welch J, Braidy N, Marquis CP. Construction and use of a Cupriavidus necator H16 soluble hydrogenase promoter (PSH) fusion to gfp (green fluorescent protein). PeerJ. (2016) 4:e2269. doi: 10.7717/peerj.2269
20. Inouye S, Noguchi M, Sakaki Y, Takagi Y, Miyata T, Iwanaga S, et al. Cloning and sequence analysis of cDNA for the luminescent protein aequorin. Proc Natl Acad Sci USA. (1985) 82:3154–8. doi: 10.1073/pnas.82.10.3154
21. Shimomura O, Johnson FH, Saiga Y Extraction, purification and properties of aequorin, a bioluminescent protein from the luminous hydromedusan, Aequorea. J Cell Compar Physiol. (1962) 59:223–39. doi: 10.1002/jcp.1030590302
22. Rizzuto R, Brini M, Pozzan T. Targeting recombinant aequorin to specific intracellular organelles. Methods Cell Biol. (1994) 40:339–58. doi: 10.1016/S0091-679X(08)61121-8
23. Robert V, Pinton P, Tosello V, Rizzuto R, Pozzan T. Recombinant aequorin as tool for monitoring calcium concentration in subcellular compartments. Methods Enzymol. (2000) 327:440–56. doi: 10.1016/S0076-6879(00)27295-9
24. Ohkura M, Matsuzaki M, Kasai H, Imoto K, Nakai J. Genetically encoded bright Ca2+ probe applicable for dynamic Ca2+ imaging of dendritic spines. Analyt Chem. (2005) 77:5861–9. doi: 10.1021/ac0506837
25. Tallini YN, Ohkura M, Choi BR, Ji G, Imoto K, Doran R, et al. Imaging cellular signals in the heart in vivo: Cardiac expression of the high-signal Ca2+ indicator GCaMP2. Proc Natl Acad Sci USA. (2006) 103:4753–8. doi: 10.1073/pnas.0509378103
26. Miyawaki A, Llopis J, Heim R, McCaffery JM, Adams JA, Ikura M, Tsien RY. Fluorescent indicators for Ca2+ based on green fluorescent proteins and calmodulin. Nature. (1997) 388:882–7. doi: 10.1038/42264
27. Palmer AE, Giacomello M, Kortemme T, Hires SA, Lev-Ram V, Baker D, et al. Ca2+ indicators based on computationally redesigned calmodulin-peptide pairs. Chem Biol. (2006) 13:521–30. doi: 10.1016/j.chembiol.2006.03.007
28. Palmer AE, Jin C, Reed JC, Tsien RY. Bcl-2-mediated alterations in endoplasmic reticulum Ca2+ analyzed with an improved genetically encoded fluorescent sensor. Proc Natl Acad Sci USA. (2004) 101:17404–9. doi: 10.1073/pnas.0408030101
29. Niswender KD, Blackman SM, Rohde L, Magnuson MA, Piston DW. Quantitative imaging of green fluorescent protein in cultured cells: comparison of microscopic techniques, use in fusion proteins and detection limits. J Microscopy. (1995) 180:109–16. doi: 10.1111/j.1365-2818.1995.tb03665.x
30. Rodriguez-Garcia A, Rojo-Ruiz J, Navas-Navarro P, Aulestia FJ, Gallego-Sandin S, Garcia-Sancho J, Alonso MT. GAP, an aequorin-based fluorescent indicator for imaging Ca2+ in organelles. Proc Natl Acad Sci USA. (2014) 111:2584–9. doi: 10.1073/pnas.1316539111
31. Kredel S, Oswald F, Nienhaus K, Deuschle K, Rocker C, Wolff M, et al. mRuby, a bright monomeric red fluorescent protein for labeling of subcellular structures. PLoS ONE. (2009) 4:e4391. doi: 10.1371/journal.pone.0004391
32. MI K. , Genetically encoded Ca2+ indicators: using genetics and molecular design to understand complex physiology. J Physiol. (2007) 578:55–67. doi: 10.1113/jphysiol.2006.120212
33. Reiff DF, Ihring A, Guerrero G, Isacoff EY, Joesch M, Nakai J, Borst A. In vivo performance of genetically encoded indicators of neural activity in flies. J Neurosci. (2005) 25:4766–78. doi: 10.1523/JNEUROSCI.4900-04.2005
34. Mank M, Reiff DF, Heim N, Friedrich MW, Borst A, Griesbeck O. A FRET-based calcium biosensor with fast signal kinetics and high fluorescence change. Biophys J. (2006) 90:1790–6. doi: 10.1529/biophysj.105.073536
35. Takahashi N, Kimura R, Tachikawa A, Miwa A, Okado H, Miyashita Y, et al. Two-photon excitation imaging of pancreatic islets with various fluorescent probes. Diabetes. (2002) S25–8. doi: 10.2337/diabetes.51.2007.S25
36. Eberhard M, Erne P. Kinetics of calcium binding to fluo-3 determined by stopped-flow fluorescence. Biochem Biophys Res Commun. (1989) 163:309–14. doi: 10.1016/0006-291X(89)92136-0
37. Eberhard M, Erne P. Calcium binding to fluorescent calcium indicators: calcium green, calcium orange and calcium crimson. Biochem Biophys Res Commun. (1991) 180:209–15. doi: 10.1016/S0006-291X(05)81278-1
38. Anderson M, Zheng Q, Dong X. Investigation of pain mechanisms by calcium imaging approaches. Neurosci Bull. (2018) 34:194–9. doi: 10.1007/s12264-017-0139-9
39. Vicario M, Cali T. Measuring Ca(2+) levels in subcellular compartments with genetically encoded GFP-based indicators. Methods Mol Biol. (2019) 1925:31–42. doi: 10.1007/978-1-4939-9018-4_3
40. Park JG, Palmer AE. Properties and use of genetically encoded FRET sensors for cytosolic and organellar Ca2+ measurements. Cold Spring Harb Protoc. (2015) 2015:pdb top066043. doi: 10.1101/pdb.top066043
41. Park JG, Palmer AE. Verifying the function and localization of genetically encoded Ca2+ sensors and converting FRET ratios to Ca2+ concentrations. Cold Spring Harb Protoc. (2015) 2015:pdb prot076547. doi: 10.1101/pdb.prot076547
42. Suzuki J, Kanemaru K, Iino M. Genetically encoded fluorescent indicators for organellar calcium imaging. Biophys J. (2016) 111:1119–31. doi: 10.1016/j.bpj.2016.04.054
43. Matz MV, Fradkov AF, Labas YA, Savitsky AP, Zaraisky AG, Markelov ML, et al. Fluorescent proteins from nonbioluminescent Anthozoa species. Nat Biotechnol. (1999) 17:969–73. doi: 10.1038/13657
44. Daubner SC, Astorga AM, Leisman GB, Baldwin TO. Yellow light emission of vibrio fischeri strain Y-1: purification and characterization of the energy-accepting yellow fluorescent protein. Proc Natl Acad Sci USA. (1987) 84:8912–6. doi: 10.1073/pnas.84.24.8912
45. Ormo M, Cubitt AB, Kallio K, Gross LA, Tsien RY, Remington SJ. Crystal structure of the Aequorea victoria green fluorescent protein. Science. (1996) 273:1392–5. doi: 10.1126/science.273.5280.1392
46. Miller DM 3rd, Desai NS, Hardin DC, Piston DW, Patterson GH, Fleenor J, et al. Two-color GFP expression system for C. elegans. BioTech. (1999) 26:920–1. doi: 10.2144/99265rr01
47. Heim R, Tsien RY. Engineering green fluorescent protein for improved brightness, longer wavelengths and fluorescence resonance energy transfer. Curr Biol. (1996) 6:178–82. doi: 10.1016/S0960-9822(02)00450-5
48. Akerboom J, Carreras Calderon N, Tian L, Wabnig S, Prigge M, Tolo J, et al. Genetically encoded calcium indicators for multi-color neural activity imaging and combination with optogenetics. Front Mol Neurosci. (2013) 6:2. doi: 10.3389/fnmol.2013.00002
49. McCombs JE, Palmer AE. Measuring calcium dynamics in living cells with genetically encodable calcium indicators. Methods. (2008) 46:152–9. doi: 10.1016/j.ymeth.2008.09.015
50. Hires SA, Tian L, Looger LL. Reporting neural activity with genetically encoded calcium indicators. Brain Cell Biol. (2008) 36:69–86. doi: 10.1007/s11068-008-9029-4
51. Tian L, Akerboom J, Schreiter ER, Looger LL. Neural activity imaging with genetically encoded calcium indicators. Progress Brain Res. (2012) 196:79–94. doi: 10.1016/B978-0-444-59426-6.00005-7
52. Tian L, Hires SA, Looger LL. Imaging neuronal activity with genetically encoded calcium indicators. Cold Spring Harbor protocols. (2012) 2012:647–56. doi: 10.1101/pdb.top069609
53. Ledoux J, Taylor MS, Bonev AD, Hannah RM, Solodushko V, Shui B, et al. Functional architecture of inositol 1,4,5-trisphosphate signaling in restricted spaces of myoendothelial projections. Proc Natl Acad Sci USA. (2008) 105:9627–32. doi: 10.1073/pnas.0801963105
54. Nausch LW, Bonev AD, Heppner TJ, Tallini Y, Kotlikoff MI, Nelson MT. Sympathetic nerve stimulation induces local endothelial Ca2+ signals to oppose vasoconstriction of mouse mesenteric arteries. Am J Physiol Heart Circ Physiol. (2012) 302:H594–602. doi: 10.1152/ajpheart.00773.2011
55. Nelson M, Ledoux J, Taylor M, Bonev A, Hannah R, Solodushko V, et al. Spinning disk confocal microscopy of calcium signalling in blood vessel walls. Microscopy Anal. (2010) 24:5–8.
56. Tallini YN, Brekke JF, Shui B, Doran R, Hwang SM, Nakai J, et al. Propagated endothelial Ca2+ waves and arteriolar dilation in vivo: measurements in Cx40BAC GCaMP2 transgenic mice. Circul Res. (2007) 101:1300–9. doi: 10.1161/CIRCRESAHA.107.149484
57. Shui B, Lee JC, Reining S, Lee FK, Kotlikoff MI. Optogenetic sensors and effectors: CHROMus-the cornell heart lung blood institute resource for optogenetic mouse signaling. Front Physiol. (2014) 5:428. doi: 10.3389/fphys.2014.00428
58. Akerboom J, Chen TW, Wardill TJ, Tian L, Marvin JS, Mutlu S, et al. Optimization of a GCaMP calcium indicator for neural activity imaging. J Neurosci. (2012) 32:13819–40. doi: 10.1523/JNEUROSCI.2601-12.2012
59. Kaestner L, Scholz A, Tian Q, Ruppenthal S, Tabellion W, Wiesen K, et al. Genetically encoded Ca2+ indicators in cardiac myocytes. Circul Res. (2014) 114:1623–39. doi: 10.1161/CIRCRESAHA.114.303475
60. Abed AB, Kavvadas P, Chadjichristos CE. Functional roles of connexins and pannexins in the kidney. Cell Mol Life Sci. (2015) 72:2869–77. doi: 10.1007/s00018-015-1964-5
61. Steppan D, Pan L, Gross KW, Kurtz A. Analysis of the calcium paradox of renin secretion. Am J Physiol. Renal physiol. (2018) 315:F834–f843. doi: 10.1152/ajprenal.00554.2017
62. Navar LG. Integrating multiple paracrine regulators of renal microvascular dynamics. Am J Physiol. (1998) 274:F433–44. doi: 10.1152/ajprenal.1998.274.3.F433
63. Skott O, Jensen BL. Cellular and intrarenal control of renin secretion. Clin Sci. (1993) 84:1–10. doi: 10.1042/cs0840001
64. Kurtz SA, Chegini S, Penner R. Lack of direct evidence for a functional role of voltageoperated calcium channels in juxtaglomerular cells. Pfliigen Arch. (1990) 416:281–7. doi: 10.1007/BF00392064
65. Taugner R, Nobiling R, Metz R, Taugner F, Buhrle C, Hackenthal E. Hypothetical interpretation of the calcium paradox in renin secretion. Cell Tissue Res. (1988) 252:687–90. doi: 10.1007/BF00216658
66. Greka, Mundel P. Balancing calcium signals through TRPC5 and TRPC6 in podocytes. J Am Soc Nephrol. (2011) 22:1969–80. doi: 10.1681/ASN.2011040370
Keywords: calcium, GCaMP, hypertension, kidney, imaging
Citation: Zhong C and Schleifenbaum J (2019) Genetically Encoded Calcium Indicators: A New Tool in Renal Hypertension Research. Front. Med. 6:128. doi: 10.3389/fmed.2019.00128
Received: 01 February 2019; Accepted: 23 May 2019;
Published: 13 June 2019.
Edited by:
Maik Gollasch, Charité Medical University of Berlin, GermanyReviewed by:
Rudolf Schubert, Universität Heidelberg, GermanyChristian Aalkjaer, Aarhus University, Denmark
Copyright © 2019 Zhong and Schleifenbaum. This is an open-access article distributed under the terms of the Creative Commons Attribution License (CC BY). The use, distribution or reproduction in other forums is permitted, provided the original author(s) and the copyright owner(s) are credited and that the original publication in this journal is cited, in accordance with accepted academic practice. No use, distribution or reproduction is permitted which does not comply with these terms.
*Correspondence: Johanna Schleifenbaum, am9oYW5uYS5zY2hsZWlmZW5iYXVtJiN4MDAwNDA7Y2hhcml0ZS5kZQ==