- 1Medizinische Klinik und Poliklinik II, Klinikum rechts der Isar, Technische Universität München, München, Germany
- 2Klinik für Anästhesiologie, Klinikum rechts der Isar, Technische Universität München, München, Germany
Sufficient organ perfusion essentially depends on preserved macro- and micro-circulation. The last two decades brought substantial progress in the development of less and non-invasive monitoring of macro-hemodynamics. However, several recent studies suggest a frequent incoherence of macro- and micro-circulation. Therefore, this review reports on interactions of macro- and micro-circulation as well as on specific regional and micro-circulation. Regarding global micro-circulation the last two decades brought advances in a more systematic approach of clinical examination including capillary refill time, a graded assessment of mottling of the skin and accurate measurement of body surface temperatures. As a kind of link between macro- and microcirculation, a number of biochemical markers can easily be obtained. Among those are central-venous oxygen saturation (ScvO2), plasma lactate and the difference between central-venous and arterial CO2 (cv-a-pCO2-gap). These inexpensive markers have become part of clinical routine and guideline recommendations. While their potential to replace parameters of macro-circulation such as cardiac output (CO) is limited, they facilitate the interpretation of the adequacy of CO and other macro-circulatory markers. Furthermore, they give additional hints on micro-circulatory impairment. In addition, a number of more sophisticated technical approaches to quantify and visualize micro-circulation including video-microscopy, laser flowmetry, near-infrared spectroscopy (NIRS), and partial oxygen pressure measurement have been introduced within the last 20 years. These technologies have been extensively used for scientific purposes. Moreover, they have been successfully used for educational purposes and to visualize micro-circulatory disturbances during sepsis and other causes of shock. Despite several studies demonstrating the association of these techniques and parameters with outcome, their practical application still is limited. However, future improvements in automated and “online” diagnosis will help to make these technologies more applicable in clinical routine. This approach is promising with regard to several studies which demonstrated the potential to guide therapy in different types of shock. Finally several organs have specific patterns of circulation related to their special anatomy (liver) or their auto-regulatory capacities (brain, kidney). Therefore, this review also discusses specific issues of monitoring liver, brain, and kidney circulation and function.
Introduction
Macro-Circulation
Organ function directly depends on appropriate supply of oxygen and energy. To maintain these prerequisites of cellular integrity and organ function, as well as to remove waste products and toxic metabolites, sufficient circulation, and perfusion are required.
The main determinants of macro-circulation are pressure and flow. Both can be measured directly with a variety of techniques. Both flow and pressure are obviously connected in analogy to Ohm's law of electricity:
Formula of Ohm's Law for Electricity and Its Analogy to Circulation
U = Voltage; I = Electric flow; R = Resistance; MAP = mean arterial pressure; CVP = central venous pressure; CO = cardiac output.
The electric flow (I) is driven by the difference of potential (voltage U) generated by the energy source, and it is modulated by the resistance within the circuit (R).
Similarly, blood flow (cardiac output CO) is driven by a pressure gradient between mean arterial pressure and central venous pressure (CVP) which is provided by a generator (heart). Obviously CO is modulated by systemic vascular resistance (SVR).
(In)Coherence of Macro- and Micro-Circulation
Under physiological conditions, macro- and micro-circulation are inter-dependent to a high degree. By contrast, for pathological conditions such as sepsis and other etiologies of shock, the loss of this “coherence” is almost pathognomonic (1, 2). Under these conditions, macro- and micro-circulation are additionally modulated by interactions of inflammation and heterogenic obstruction of the micro-circulation (3).
While CO and MAP are unquestioned cornerstones to provide appropriate perfusion, normal values of both parameters do neither preclude a misbalanced oxygen delivery and demand nor an impaired microcirculation.
Adequacy of Macro-Circulation: The “Bridge to Micro-Circulation”
Mixed Venous Oxygen Saturation SvO2, Central-Venous Oxygen Saturation ScvO2
To assess the adequacy of global perfusion and appropriate local oxygen supply, a number of biochemical markers have been suggested and included in guideline recommendations (4, 5). Abnormal values of ScvO2, plasma lactate and cv-a-pCO2-gap have been associated with poor outcome in a large number of studies (Table 1). Therapeutic algorithms aiming at normalization of these parameters improved outcome in a number of studies (6, 9), but failed in other trials (10). The use of these parameters is appealing due to their low costs and the easiness of measurement. Their appropriateness to guide therapy is limited in populations with a high prevalence of patients with normal values (10). This might also explain contradictory results of studies using these parameters to guide therapy.
In general, normal values of MAP and CO do not preclude pathological values of ScvO2, plasma lactate, and cv-a-pCO2-gap and vice-versa (11–13). Therefore, ScvO2, plasma lactate and cv-a-pCO2-gap are used in a combined approach with CO to reflect the adequacy of CO in a certain clinical context.
Since these parameters provide information in addition to macro-circulation and reflect metabolism, they can be considered as some kind of “bridge to micro-circulation.” Per definition, SvO2 and ScvO2 are closely associated with macro-circulation. Applying the Fick equation to O2 results in
Necessarily, with constant SaO2, VO2, and Hb, decreasing values of CO result in decreases of SvO2 due to a compensatory increase in the oxygen extraction rate. Normal values for S(c)vO2 in healthy subjects range from 70 to 75%.
While measurement of SvO2 requires withdrawal of blood from a pulmonary arterial catheter (PAC) or oximetric measurement with a special PAC, ScvO2 can be obtained easily from a conventional central venous catheter (CVC) or continuously with a specific oximetric CVC. Although ScvO2 and SvO2 may differ due to the slightly different oxygen content of blood returning from the lower and upper half of the body, it is well accepted to replace SvO2 by ScvO2 for clinical purposes.
Lactate
Among the bridges from macro- to micro-circulation plasma lactate is the parameter which is most “down-stream,” i.e., close to microcirculation and cellular metabolism (14). A variety of experimental and clinical studies demonstrated that lactate levels indicating anaerobic metabolism increase in parallel with a decreasing ratio of oxygen utilization divided by oxygen demand. Increasing lactate levels are associated with abnormal oxidative phosphorylation (4). Lactate levels of >2 mmol/L are considered to be abnormal, but also lower cut-offs (>1.5 mmol/L) have been associated with poor outcome in patients with sepsis (15).
At least two trials associated decreasing lactate levels and lactate-guided early-goal directed therapy with improved outcome (9, 16). Finally, lactate has become part of the new definition of septic shock (5).
Based on these findings several recent guidelines recommend lactate measurement every 2 h within the first 8 h and every 8–12 h thereafter after admission with shock (4).
A major advantage of guiding therapy by lactate levels is the easiness of measurement which does not require central-venous access. Similar as for SvO2 by ScvO2 devices providing continuous measurement are available (7, 17).
However, it has to be kept in mind that hypoperfusion is not the only reason cause of elevated lactate levels. Impaired liver function and stress can also contribute to increases in lactate levels.
Central-Venous—Arterial CO2 Difference (cv-a-pCO2-gap)
Among the parameters used as a bridge to micro-circulation, cv-a-pCO2-gap plays an intermediate role between ScvO2 and lactate. Similar to ScvO2 the veno-arterial difference in pCO2 facilitates interpretation of adequacy of CO and resuscitation. If O2-extraction is impaired due to micro-circulatory mal-distribution, ScvO2 may be normal despite a reduced CO. In this case, a cv-a-pCO2-gap >6 mmHg suggests inadequate perfusion, even if ScvO2 is above 70% (11, 13).
In summary, ScvO2, plasma lactate and cv-a-pCO2-gap have two important roles:
1) When extended hemodynamic monitoring including CO is not available, they can be used as easily measurable indicators of the adequacy of blood flow.
2) If CO is available, pathological values of these markers increase the likelihood of circulatory improvement by increasing CO.
SvO2: mixed venous oxygen saturation
ScvO2: central-venous oxygen saturation
cv-a-pCO2-gap: central-venous and arterial CO2 ().
Micro-Circulation
While the macro-circulatory interconnections are transparent and easy to be determined, micro-circulation is much more challenging.
Micro-circulation cannot be defined in a clear-cut formula such as Ohm's law. Consequently, assessment of micro-circulation is more complicated due to its dependency on macro-circulation, organ-specific auto-regulatory mechanisms and interactions between certain organs. Furthermore, the technical accessibility to quantify micro-circulation is much more complicated compared to macro-circulation.
Therefore, micro-circulation is assessed based on a plethora of clinical, chemical, and physical surrogates which are frequently restricted to the individual micro-circulation of a single organ.
Clinical Assessment of Micro-Circulation
The first approach to the assessment of micro-circulation is clinical examination aimed at detection of impaired general and specific circulation (Table 2).
The most “accessible” organ without any instrumental approach is the skin. Among all organs, skin has the largest weight and contributes about 16% of the body weight, i.e., about 10 kg in a normal weight adult.
A structured assessment of the microcirculation of the skin starts with inspection and palpation aiming at estimation of the surface temperature. In patients with shock this allows for primary classification of “cold” shock and “warm” shock. “Warm shock” is caused by several etiologies of distributive shock including septic, anaphylactic and neurogenic shock.
The etiology of “cold shock” can be hypovolaemic, cardiogenic, or obstructive (pulmonary embolism, pericardial tamponade, pneumo-thorax). “Warm shock” is caused by endogenous or exogenous vasodilators. Most frequently it is due to sepsis. Cold shock typically is mediated by endogenous vasoconstrictors such as nor-adrenaline which is considered as a physiological compensatory mechanism in order to provide and stabilize the perfusion of the most vital organs such as brain, heart and lungs. Most of the other organs including the skin are summarized as “shock organs” that can tolerate markedly reduced perfusion for a certain time.
Regarding the extent of the organ skin, its impaired perfusion is not a regional cosmetic side effect, but has systemic implications for the organism's thermal balance. Abnormal dermal vasoconstriction or vasodilatation results in reduced or increased thermal transfer from the body core to the surface and consecutive changes in the skin temperature (22). Due to the absence of auto-regulatory mechanisms found in the brain, heart and lungs, skin perfusion, and temperature closely reflect the activation of neuro-humoral mechanisms during different forms of shock.
The clinical assessment of the skin temperature should be performed with the investigator's back of the hand, since this part of the hand is most sensitive for temperature. Due to the moderate discriminatory power of this approach, a classification of the temperature as cold, slightly reduced, normal, and warm has been suggested.
To improve the assessment of skin temperature, two approaches can be used:
1) Instrumental measurement of skin temperature
2) Skin-core temperature gradients (SCTG).
The association of cutaneous temperature and cardiovascular function was first described by Hippocrates. The first validation using an instrumental approach was published in 1954 by Felder et al. who demonstrated an association of toe temperature and blood flow measured with a plethysmograph (23). The clinical use of toe temperature measurement to guide vasodilator therapy in shock was described by Ibsen and co-workers (24). Joly and Weil demonstrated a strong association of the toe temperature continuously measured with a probe and cardiac output determined with indicator dilution technique (18). The correlation (r = 0.71) was better for toe temperature compared to skin temperature on third finger, deltoid area of the arm, lateral portion of the thigh, and rectal temperature. It was slightly improved when the toe temperature was adjusted to ambient temperature. Furthermore, skin temperature on admission and in particular its changes over time excellently predicted survival. Henning and colleagues demonstrated in 71 patients with acute circulatory failure due to myocardial infarction, sepsis or hypovolaemia that the toe ambient temperature gradient better predicted mortality than cardiac index or arterial pressure (25). A study by Vincent et al. demonstrated that the association of toe-ambient temperature gradient to cardiac output was more pronounced in patients with cardiogenic shock compared to septic shock (26).
A more recent study investigating the prognostic value of the subjective assessment of peripheral perfusion in critically ill patients showed that central to toe temperature and the skin temperature gradient between the forearm and the index finger were significantly different for patients with and without abnormal peripheral perfusion which was substantially associated to outcome (27). Another recent study demonstrated that toe-to-room and central-to-toe temperature gradients correlated with tissue perfusion and predicted death of multi-organ-failure in septic patients (28).
All of the above-mentioned studies used probes attached to the skin for the measurement of the surface temperatures.
While this allows for intermittent as well as continuous measurement, the attachment of probes also carries several disadvantages: The probes have to be connected to a special monitor which is not ubiquitously available. Furthermore, connection cables maybe disturbing. Finally, continuous attachment of a probe to the skin might alter the temperature at the place of measurement and might cause hygienic problems.
All these problems can be overcome by the use of non-contact infrared thermometers. Several recent studies report on comparable predictive capacities of surface temperature measured with non-contact infrared thermometers. While these devices are ubiquitously available and easy to use, the approach of thermal imagery is predominantly of scientific and potentially clinical interest. A recent animal study demonstrated significant association of several parameters derived from a non-contact long-wav-infrared camera with MAP, shock-index, paO2, and P/F-ratio (29).
Whereas, other clinical criteria for peripheral perfusion such as mottling of the skin (see below) might fail in patients with colored skin, instrumental measurement of skin temperature is independent of its color.
In addition to the assessment of surface temperature, clinical examination of skin perfusion includes structured static, and dynamic optical examination of the peripheral perfusion.
Mottling of the Skin
Mottling of the skin has been defined as a patchy skin discoloration that frequently starts around the knees. It is caused by heterogenic constriction of micro-vessels (19). More recently, a structured assessment including a staging (see Table 3) depending on the extent of mottling has been introduced by Ait-Oufella et al. (19). Depending on the extent of the mottled area, this score ranges from 0 to 5.
High inter-observer agreement with a kappa-value of 0.87 has been reported in this study. Baseline mottling-score as well as its changes over time were strongly associated to outcome, whereas mean arterial pressure, CVP, and CI failed to predict 14-days mortality (19). Another study by the same group demonstrated a close association of the mottling score to changes in skin perfusion (30). Based on instrumental investigations with laser Doppler imaging and near-infrared spectroscopy it was shown that in mottled skin areas perfusion as well as tissue oxygenation are reduced (31). The use of the mottling score has been validated in several studies in patients with sepsis (32, 33) as well as liver cirrhosis (34).
Capillary Refill Time
Capillary refill time (CRT) is defined as the time required for the skin to return to the baseline color after application of a blanching 15s pressure to the distal phalanx of the right and left index (31, 35, 36). The association of CRT with severity of shock was first described more than 70 years ago (20). More recently, CRT has been suggested as a standard in particular for advanced pediatric life support (37) for more than four decades (21). In non-selected critically ill adult patients a CRT >4.5 s was associated with worse outcome. In another study a CRT >5 s was associated with perioperative complications and death after major abdominal surgery (38). Furthermore, a recent study suggests that changes in CRT might be used as targets to stop resuscitation in patients with septic shock (37).
Despite the appealing simplicity of CRT there are several limitations of this parameter: The inter-observer variability has been poorly investigated and resulted in contradictory findings (38–40). Furthermore, a variety of different cut-offs are suggested depending on age and gender of the patients (39, 41, 42).
A recent study demonstrated an association of visceral organ vascular tone with CRT and mottling score, but not with body surface temperature. However, skin temperature was determined in a dichotomous way (warm or cold) by subjective assessment of the examiner, but not with a thermometer or a probe (43).
Normalization of the skin perfusion might be used as a goal for resuscitation, since it occurs earlier during resuscitation than normalization of lactate levels (22, 37, 41).
Finally, a structured combination of clinical parameters of the skin perfusion might improve resuscitation compared to standard algorithms (44) (Table 4).
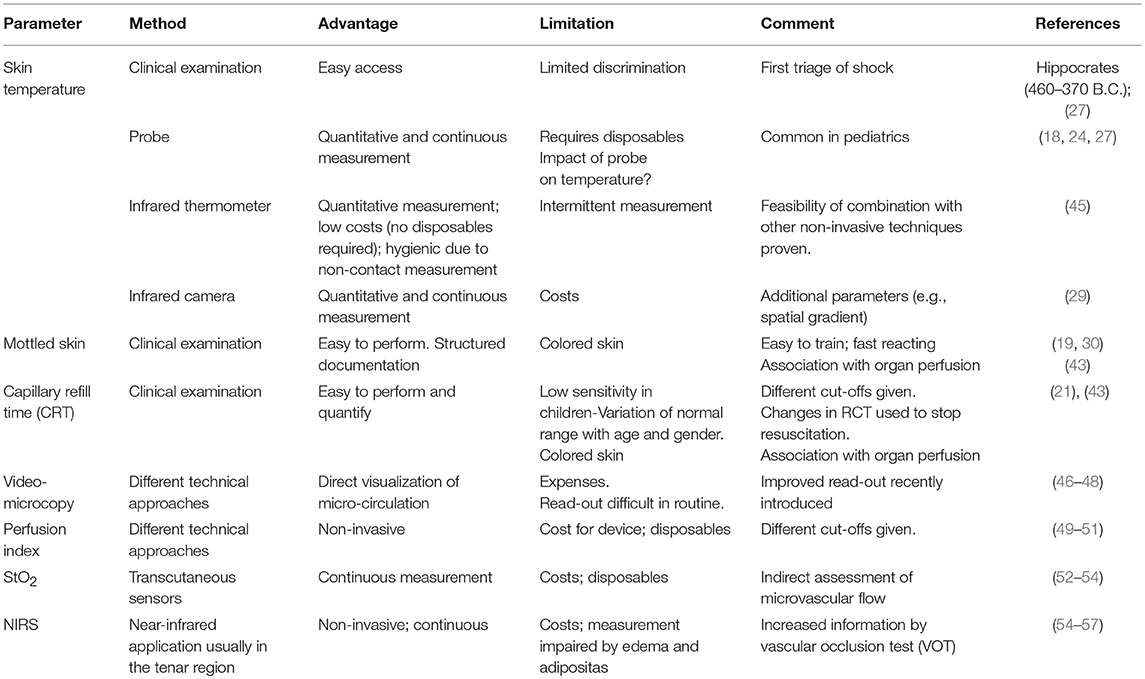
Table 4. Clinical and technical approaches to assess micro-circulation [modified according to Tafner et al. (36)].
Technical Assessment of Microcirculation
In addition to the above-mentioned structured clinical approaches to assess peripheral perfusion and body surface temperatures, a number of more sophisticated instrumental techniques have been introduced within the last two decades. Due to their technical properties they are frequently restricted to measurement of microcirculation in certain regions. Extrapolation of the findings in these specific areas to general peripheral perfusion has to be done cautiously. Finally, it has to be kept in mind that these techniques are not widely available and predominantly used for research purposes (36, 58).
Peripheral Perfusion Index
Based on pulse oximetry and the amount of absorbed infrared light, several commercially available devices provide a “perfusion index” (PI) which is calculated as the ratio of pulsatile blood-flow to non-pulsatile blood-flow (49). Absorption of light with different wavelengths can be measured percutaneously. The absorbance has a pulsatile component resulting from changes in peripheral arterial blood flow and volume, whereas the constant non-pulsatile part of absorption is due to venous blood and extravascular tissues between the light source and the detector.
Baseline values and changes of PI correlate to core-to-toe temperature difference (49). In this study the best cut-off to predict poor tissue perfusion was a PI <1.4. By contrast, a more recent study using a different device demonstrated that a PI >3.5 was associated with detrimental vasodilatation and a higher incidence of hypotension following spinal anesthesia (50). In a study in septic patients a PI <0.2 was associated with poor outcome (51). The main advantage of PI monitoring it is easy to use and inexpensive. On the other hand—as for many of these techniques—the information about micro-circulation is restricted to the investigated area. Furthermore, detection of the signal is impeded by a more pronounced endogenous vasoconstriction or high-dosage vasopressor use.
Video-Microscopy
Video-microscopy is among the more generally known techniques (58, 59). It is usually performed in the sublingual area (46). Sublingual microcirculatory abnormalities include decreases in the proportion of perfused vessels (PPV), in the total density of small micro-vessels, and in microvascular flow index (MFI) as well as an increased heterogeneity of the micro-perfusion.
These alterations have been associated with hyperlactataemia, vasopressor dependency, organ dysfunction, and mortality (60). Resuscitation and inotropes have been shown to normalize sublingual microcirculatory abnormalities (61). Moreover, reduction in MFI has been identified as an independent risk factor for mortality (62).
The technique has been refined over time. Three generations of devices have been introduced within the last two decades: Orthogonal polarization spectral imaging (OPS), side-stream dark field (SDF; 2nd generation), and incident dark field illumination (IDF; 3rd generation). The 3rd generation technology provides better image resolution and visualization of more capillaries than OPS and SDF-devices (46). Video-microscopy has been used to some extent for educational purposes to make micro-circulatory disturbances more evident. While the educational merits of the technique are unquestioned, its clinical use is limited due to several limitations.
The currently available technologies are time consuming, expensive and require a thorough training as well as a complex analysis of the measurements. Several pitfalls including artifacts induced by compression and saliva have to be considered (46).
The normal ranges of PPV and MFI still are discussed and in part contradictory (22, 63, 64). The large variation of PPV and MFI in healthy volunteers might be in part related to compression artifacts induced by the hand-held video-microscopes (22).
To improve the quality of imaging and to standardize the description of the findings, several scores and consensus recommendations have been established (46, 65).
Despite the use of standardized examination and supporting software the examination remains to be time-consuming (22) and limits a more widespread use.
This might be improved by automated devices. However, at present there are still concerns regarding their reliability. Several studies demonstrated poor agreement of manual with automated measurements of sublingual micro-circulation (66, 67).
If technical development will provide these techniques more readily available for clinical use at a reasonable price, this could be a major step forward to bring microcirculatory parameters into the first line of shock assessment and guidance of therapy (68, 69). However, the additional value of these technologies in addition to thorough clinical examination has been questioned (70).
Laser Doppler Flowmetry
Laser Doppler Flowmetry (LDF) is based on a confocal technique which analyses vascular density, diameters and flow in arterioles, capillaries and venules (36, 71). While the method has the limitation not to differentiate the flow in these different types of vessels, it has the advantage that it can be used at any region of the skin (36).
Partial Oxygen Pressure Measurement, Near Infrared Spectroscopy (NIRS)
This method uses transcutaneous electrodes for continuous measurement of oxygen (PtcO2) and carbon dioxide concentrations in the tissue.
NIRS detects and measures chromophores which are related to the color of molecules. Among those molecules are myoglobin, oxyhemoglobin, and deoxyhemoglobin. Among the parameters derived from NIRS StO2 is the most commonly applied one (72). StO2 usually is measured in the tenar region where the lack of adipose tissue allows for a close contact with the muscle tissue. The signal detected by NIRS is not directly related to blood flow within a specific vascular bed, since it reflects StO2 based on signals from arterioles, capillaries, and venules (36). NIRS signals may be misleading in case of cold extremities (70). The clinical usefulness might be increased by a standardized test with a short term ischemia (vascular occlusion test VOT) which can help to assess the microvascular reserve and oxygen consumption in the tenar muscle (55–57).
In summary, all the above-mentioned techniques provide better insight in micro-circulation than traditional parameters of macro-circulation. Structured clinical assessment of micro-circulation is mandatory in critically ill patients. Future developments will facilitate the use of technical approaches to assess and quantify micro-circulation. This might help to use these parameters to guide resuscitation and to avoid of over-hydration in septic shock (69). However, the superiority of these techniques to meticulous and standardized clinical examination remains to be proven.
Organ Specific Micro-Circulation
Several organs have specific patterns of circulation related to their special anatomy (liver) or their auto-regulatory capacities (brain, kidney).
Therefore, the following paragraphs will address specific issues of monitoring brain, liver, and kidney circulation and function.
Monitoring of Microcirculation and Function of the Brain
Although the brain accounts for only 2% of the total body weight in humans, it requires about 20% of the body's blood supply. Brain function depends on the continuous delivery of oxygen and nutrients. Even short interruptions of this supply may lead to decrease of neuronal function and subsequent brain cell death.
Transcranial Doppler Sonography
Cerebral blood flow (CBF) is of critical importance for cerebral oxygen delivery (CDO2). Transcranial Doppler sonography (TCD) can be employed to assess CBF. With TCD, erythrocyte flow velocity is measured in large cerebral arteries using low-frequency ultrasound. Blood flow velocity depends not only on blood flow, but also on the diameter of the arterial vessel, and thus blood flow velocity only provides an estimate of CBF (73).
A flow reduction in cerebral arteries may lead to cerebral ischemia. Unfortunately, a generally valid threshold value cannot be defined. Some authors suggest absolute values of 25 or 30 cm/s (74, 75), others suggest relative reductions ranging from at least 60–80% (76, 77).
TCD has several limitations:
1) Exact quantification of CBF is not possible with TCD, since the exact vessel diameter cannot be determined.
2) Skull bone is largely impermeable for ultrasound waves. Therefore, TCD can only be performed at sites where the bone is rather thin.
3) Reflection of ultrasound (i.e., measured blood flow velocity) depends on the angle between probe and direction of blood flow. Therefore, the result significantly depends on probe position and user experience. This may in part be overcome by the use of transcranial duplex.
Due to these limitations, specific skills and knowledge are a prerequisite in order to obtain reproducible and reliable results with TCD.
Near-Infrared Spectroscopy
While measuring CBF can give valuable information on cerebral oxygen delivery, it cannot provide information on cerebral oxygen consumption. Therefore, continuous real-time monitoring of cerebral oxygenation using near infrared spectroscopy (NIRS) may provide important therapeutic information. This technique was introduced into clinical practice in the 1980s for the assessment of cerebral oxygenation in preterm infants (78). Similar to pulse oximetry, the technical principle of NIRS is based on differences in the absorption of light by oxygenated and desoxygenated hemoglobin (Hb). Photons emitted at wavelengths in the near-infrared spectrum penetrate scalp, skull bone and brain tissue (79). In contrast to pulse oximetry, NIRS is not based on transluminescence, but analyses reflected photons. The ratio of oxygenated Hb to total Hb, expressed as regional cerebral oxygen saturation (rScO2), can be estimated using principles of optical spectrometry. Usually, optodes containing sender and receiver are placed on the patients' forehead (often bilaterally). Measured rScO2 values then resemble oxygen saturation in the frontal cortex, an area that is very sensitive to hypoxemia. Since the near-infrared light penetrates extra-cerebral (scalp, skull bone) and intra-cerebral tissues, all of which absorb light, a low cerebral oxygen saturation may be masked by a high extra-cerebral oxygen saturation, which is called “extra-cerebral contamination” (80). This phenomenon can be reduced technically in modern NIRS monitors, but not totally eliminated.
Depending on the algorithm and the number of photon emission wavelengths used, rScO2 values may be measured as “relative” or “absolute” values. Although “absolute” values are sometimes claimed to be superior over “relative” values, there is no data yet available supporting this view.
Cerebral blood volume constitutes of ~20% arterial, ~5% capillary, and ~75% venous blood. Therefore, saturation measured by NIRS essentially resembles venous saturation. At room air, rScO2 was found to be around 70% in healthy volunteers (81), and around 60% in older general surgery patients (82) as well as in a mixed population of cardiac surgery patients (83). Due to a high individual variance of rScO2, it is crucial to obtain baseline readings in all patients. Furthermore, preoperative rScO2 values strongly correlate with cardiac performance (84) and are also associated with outcome in cardiac surgery (85). The threshold value of rScO2 below which the likelihood of complications increases, is currently under intensive investigation. Furthermore, it is currently unclear for how long or how often rScO2 has to go below such a limit in order to negatively influence outcome. Due to the high variability in baseline values, some authors suggest to use relative decreases in rScO2 of 20 (86) or 25% (87) as indicators for hypoxemia, rather than absolute values.
Regardless of the threshold used, several studies concerning general and cardiac surgery show, that avoidance of perioperative cerebral desaturation is accompanied by a lower rate of stroke, postoperative cognitive decline, delirium, overall major organ dysfunction and mortality (82, 86, 88–90). Therefore, an acute decrease in rScO2 below a threshold needs a thorough work-up of possible causes and consecutive therapy. The algorithm suggested by Denault et al. that includes optimization of head positioning, arterial blood pressure, oxygenation, ventilation, and Hb-concentration has proved effective for this purpose (91).
Today, NIRS monitoring is mainly used in cardiac surgery and surgery of the thoracic aorta. Its use is recommended for correction of congenital heart defects during childhood as well as operations of the aortic arch in children and adults. It may help detect cannula misplacement during cardiopulmonary bypass and identify patients, in which unilateral ante-grade cerebral perfusion is insufficient. Furthermore, NIRS can be helpful to regulate flowrate during unilateral ante-grade cerebral perfusion in order to prevent hypo-perfusion.
NIRS monitoring has also been evaluated outside of cardiac surgery. During carotid endarterectomy, measurement of rScO2 would appear to provide valuable information, since hypoxemic stroke strongly contributes to perioperative morbidity and mortality. However, current data are controversial and the use of NIRS during carotid endarterectomy can only be recommended as second line strategy, when monitoring of evoked potentials is not available.
Surgical procedures in beach chair position have been associated with very rare cases of ischemic brain damage in healthy patients, possibly due to cerebral hypo-perfusion. Using NIRS, some studies could identify significant decreases in rScO2 when beach chair position was applied, whereas others could not. Due to the very low incidence of cerebral hypoxemia during beach chair position, routinely measuring rScO2 seems difficult to justify.
In summary, NIRS monitoring provides a simple, fast, non-invasive, and continuous measurement of rScO2 that can be applied on patients of all ages. It may be of particular value when both intra- and extracranial perfusion is altered. Limitations are high costs of single-use optodes, contamination with extracranial signals and reduced sensitivity for detection of focal ischemia due to limited spatial resolution of commercial monitors.
Table 5 summarizes the discussed methods of monitoring brain circulation and function.
Regional Perfusion of the Liver and Markers of Liver Function
Assessment of liver function is based on markers of excretory function, synthesis, and cellular integrity within the liver. All these criteria are substantially dependent on appropriate perfusion of the liver. Macro-circulation is usually assessed by (Doppler-) ultrasound and CT-(angiography). Direct angiography using arterial, portal-venous or venous access is less frequently required. Furthermore, hepatic hemodynamics can be assessed by catheterization of the liver vein. Use of a balloon catheter and measurement of the occlusion pressure may help to analyze the extent and etiology of portal hypertension.
Impaired hepatic perfusion and metabolism usually affect excretion, cellular integrity, and synthesis of the liver.
To quantify these three qualities of liver function numerous parameters can be determined routinely. Most of them are considered as static, since these parameters except parameters of cellular integrity are slow-reacting and reflect liver function with substantial latency. This applies particularly to the parameters of liver synthesis: The most commonly measured biochemical parameters reflecting liver synthesis cholinesterase ChE and albumin have half-life times of 10 and 20 d, respectively. Fast-reacting parameters such as factor V (half-life time: 4 h) and factor VII (half-life time: 5 h) usually lack a 24/7-availability.
Another draw-back of the static parameters is that many of them are not specific for hepatic pathologies. This applies to elevations of bilirubin which can have pre-hepatic (hemolysis), intra-hepatic (cirrhosis, hepatitis), and post-hepatic (biliary obstruction) origin. Similarly, increases in alkaline phosphatase may result from bone pathologies as well as from cholestasis. Furthermore, due to their specific properties and distribution, several enzymes reflect certain hepatic pathologies to a different degree: this applies to glutamate dehydrogenase GLDH and glutamate-oxalacetate-transaminase GOT (= ASAT aspartate-amino-transferase) which reflect particularly peri-central and peri-portal cellular damage, respectively. Both enzymes are not specific for the liver.
Furthermore, the distribution of liver enzymes within the hepatocyte is different: While glutamate-pyruvate-transaminase (= ALAT alanine- amino-transferase) is restricted to the cytoplasm, GOT/ASAT is found in the cytoplasm and mitochondria, and GLDH is restricted to the mitochondria.
To overcome some of these disadvantages, combined scores, and models of liver function have been introduced (Tables 6, 7). These score take into account different qualities of liver function such as excretion and synthesis and combine this information with clinical findings in liver failure (Child-Pugh-score; Table 6) or laboratory data reflecting organ failure associated with severe liver impairment (model of end-stage liver disease (MELD; Table 7).
Although these classifications and scores have been shown to better reflect severity and prognosis of severe liver impairment compared to single static markers, they can be influenced to a large extent by therapeutic measures such as substitution of plasma and coagulation factors, extracorporeal organ support such as dialysis and liver support, blood transfusion, plasma separation, and clinical interventions such as paracentesis with and without substitution of albumin. One early and important criticism regarding the MELD score was the potential misuse and manipulation of the score by therapeutic interventions influencing serum creatinine, bilirubin, and INR to increase LTX-likelihood. Indeed, it is a general weakness of MELD that all three components can be influenced with and without intent (Table 8).
By contrast, most of the dynamic tests of liver function are not directly influenced by the above-mentioned measures with a potential impact on the association of MELD with liver function.
Interestingly, at least one study demonstrated an independent association of a dynamic liver function test (indocyanine green disappearance rate ICG-PDR; see below) in addition to MELD with survival of patients on the transplantation list (92).
Dynamic Markers of Liver Function
To overcome the above-mentioned short-comings of static parameters of liver function, a number of dynamic tests have been suggested (Table 9). In general, they have in common that they repeatedly or continuously analyze liver function over a short period of time (minutes to hours). Depending on the read-out the results can be obtained online at bedside.
Dynamic liver tests are based on excretion, elimination, and metabolism of a test agent that is orally or parenterally applied (Table 10).
For most of the tests repetitive blood samples are required to measure the kinetics of the agent or its metabolites. Among others, this applies to lidocaine, caffeine, galactose, and bromsulfophtaleine.
After injection of 1 mg/kg lidocaine the agent is N-dealkylated to mono-ethylglycinexylidide (MEGX). To asses liver function, MEGX-concentrations before and in intervals of 15 min after application can be measured, and the MEGX-half-life time can be calculated. MEGX-testing has been used to some extent in clinical studies, and it has been clearly associated with morbidity and mortality (93). Nevertheless, at present the availability within routine laboratories is poor.
The same applies to the caffeine test which is based on measurement of the serum concentrations of the caffeine metabolites paraxanthine, theobromine and theophylline and their ratio to caffeine 4, 8, and 12 h after the oral ingestion of 300 mg of caffeine.
Similarly, the elimination of D-galactose by metabolization to galactose-1-phosphate and further degradation can be quantified (galactose elimination capacity GEC) by repeated blood drawings after infusion of 0.5 g/kg 25% D-galactose solution. Also due to potentially life threatening side effects in case of galactose-intolerance, this test has lost its clinical use. Finally, the elimination of bromsulfopthaleine administered at a dosage of 5 mg/kg can be quantified by drawing blood samples after 30 and 45 min.
While these biochemical metabolic tests all have a pathophysiological rationale and documented associations to morbidity and outcome, their practical use is low due to the need of elaborate non-routine biochemical analyses.
Further limitations of tests based on oral application of the test-agent are the frequently impaired gastrointestinal motility and absorption in case of critically ill and patients with liver failure.
As a consequence, in particular two tests with online and bedside availability remain to be more commonly used:
The most widespread method is the indocyanine green (ICG) elimination.
ICG is an infra-red absorbing and fluorescent dye which was originally introduced around WW-II as a dye in photography.
Due to its physical properties and overall low toxicity ICG became wide-spread in critical care in the 1990s. It was used for indicator dilution techniques to derive cardiac output (trans-pulmonary thermo-dilution TPTD) and extravascular lung water EVLW (double-indicator TPTD). Double-indicator TPTD used ice-cold ICG for indicator dilution with two different distribution volumes for the dye (remaining in the vasculature) and the thermal indicator (also diluting in EVLW). Concomitant measurement of the distribution volumes of the thermal indicator and the dye indicator allowed for exact calculation of EVLW. This technique required extra-corporeal or in vivo measurement of ICG-concentrations over time. While the time to derive CO and EVLW is short (time for complete transit of the indicator to the site of detection), the prolonged measurement of the ICG elimination curve allows for assessment of the liver function. Double indicator TPTD technique and liver function were combined in at least one commercially available bedside device (COLD: Cardiac Output and Liver Diagnostic; Pulsion Medical Systems, Germany). While the main interest regarding this device might have been advanced hemodynamic monitoring, each measurement necessarily provided dynamic assessment of liver function.
Another bedside available dynamic liver function test which is routinely used to a certain degree in clinical practice is the LiMAX-test (maximum liver function capacity). As for other exhalation tests, the LiMAX test analyses the exhalation of a substrate of a 13C or 14C-labeled test agent. To overcome the problem of potentially impaired resorption of an orally applied test agent, the LiMAX test quantifies the exhalation of 13CO2 derived from metabolism of intravenously applied 13C-methacetin. The test agent 13C-methacetin is metabolized by cytochrome-P450-isoenzyme 1A2 (CYP450 1A2) to acetaminophen (paracetamol) and 13CO2 which is measured by a breath-test. Similar as for ICG-PDR the results from the LiMAX-test have been associated to outcome and liver-resectability of patients with impaired liver function (95–99).
Non-Invasive Assessment of Renal Perfusion and Function
Acute kidney injury (AKI) is one of the most common and life-threatening complications which significantly affects morbidity and mortality of intensive care unit patients (100). The most challenging issue in these patients is to identify high-risk patients who should experience early recognition of AKI (100, 101). Overall, diagnosis of AKI is still based on laboratory testing and/or oliguria and its normalization.
However, these more clinical criteria have some limitations. Distinguishing transient AKI from persistent AKI is of clinical relevance, thus stressing the need for criteria to predict its reversibility (102, 103).
One major problem in AKI is that the definition suffered for a long time from a lack of standardized system of identifying and classifying this syndrome. First, the RIFLE criteria (Risk, Injury; Failure, Loss, End stage renal disease) were proposed by the Acute Dialysis Quality Initiative. In the meanwhile, the Kidney Disease Improving Global Outcomes (KDIGO) group defined an unified version of all criteria which now present global consensus (104).
Although urinary analysis and urinary biochemistry have limited clinical utility, the diagnosis of AKI is traditionally based on a rise in serum creatinine and/or fall in urine output (UO). UO is important not only for diagnosis, but also for risk prediction of AKI (105, 106).
Typical causes of AKI in critically ill patients are sepsis, heart failure, hemodynamic instability, hypovolaemia, and exposure to nephrotoxic substances (103). The specific diagnostic workup in individual patients with AKI depends on the clinical context, severity, and duration of AKI, and also on the local availability of the tests. As mentioned above, urinalysis, examination of the urinary sediment, and imaging studies should be performed as a minimum, with additional tests depending on the clinical presentation (107).
Over the last years, basic diagnostics are increasingly being completed by novel biomarkers of AKI. Biomarkers for AKI can be stratified into markers primarily reflecting glomerular filtration (i.e., serum cystatin C), glomerular integrity (i.e., albuminuria and proteinuria), tubular stress [i.e., insulin-like growth factor binding protein 7 (IGFBP-7)], tubular damage [i.e., neutrophil gelatinase-associated lipocalin (NGAL), kidney injury molecule-1 (KIM-1)], and intra-renal inflammation (i.e., interleukin-18) (108).
The interest in biomarkers is combined with the desire to achieve early diagnosis and detection of renal stress or damage before functional change is evident.
The tubular damage marker “Neutrophil gelatinase associated lipocalin” (NGAL) is one of the most investigated renal biomarker (109). NGAL is typically upregulated in kidney tissue when exposed to nephrotoxic or inflammatory stress, but also released by activated neutrophils with specific forms of the molecule released from the kidney (monomeric) and neutrophils (dimeric) (108, 109). In one analysis of >2,000 critically ill patients, 20% were NGAL-positive without an increase in serum creatinine which can be interpreted as subclinical AKI or false positive results. However, these patients are at great risk of subsequent renal replacement therapy (RRT), longer ICU and hospital stay, and death. Similar findings were observed in emergency department patients (109).
Other molecules such as the kidney injury molecule (KIM-1), tissue inhibitor of metalloproteinases-2 (TIMP-2), and (110) insulin-like growth factor binding protein-7 (IGFBP-7) appears to perform similarly to NGAL, but have not been studied to the extent of NGAL in critically ill patients.
Beyond early diagnosis and risk stratification, these biomarkers may also help to change the definition of AKI in the future and contribute to a better understanding, diagnosis, prevention, and treatment of AKI (110).
In certain circumstances, it may be necessary to use additional tools to diagnose AKI, especially in cases where creatinine and urine values change slowly, are misleading, or cannot be interpreted accurately. This is particularly relevant for critically ill patients where the presence of fluid overload, muscle wasting, sepsis, and reduced effective circulating volume may completely mask the diagnosis of AKI (104).
Renal ultrasonography is useful for evaluating existing structural renal disease and diagnosing obstruction of the urinary collecting system. In detail, the presence of reduced corticomedullary differentiation and decreased kidney size is indicative of underlying CKD (111).
Up to now, there are no good markers of medullary oxygenation as well on how to assess an improvement in medullary oxygenation in AKI (43, 112).
Renal Doppler ultrasound and contrast-enhanced ultrasound are two techniques that may be used at the bedside to estimate renal perfusion and renal cortical microcirculation, respectively (113).
Especially, Doppler-based renal resistive index (RRI) measurement is rapid, non-invasive, and repeatable and may therefore hold promise for monitoring renal function or renal perfusion in critically ill patients (114, 115).
The renal resistive index [RRI = (peak systolic velocity–end diastolic velocity)/peak systolic velocity] consists of the measurement of renal arterial resistances to blood flow detected by echo-color-Doppler system. RRI is reliably correlated with kidney injuries and its severity. In several trials, RRI showed a direct correlation with cardiovascular damage, acute tubular necrosis, and—in septic shock patients—with the prediction of AKI occurrence (114, 115).
Furthermore, depending on the clinical context, patients may require specific immunological tests, including anti-neutrophil cytoplasmic antibody (ANCA), anti-nuclear antibody (ANA), anti-glomerular basement membrane antibody (anti-GBM), and complement component 3 and 4 to rule out immune-mediated diseases (i.e., vasculitis, connective tissue diseases) (104). These investigations should be considered mandatory in patients with AKI presenting primarily with a pulmonary-renal syndrome, hemoptysis, or hemolysis/thrombocytopenia.
Renal biopsies are rarely performed in critically ill patients, mainly due to the perceived risk of bleeding complications and general lack of therapeutic consequences (114). However, a renal biopsy may offer information that is not available through other means and should be considered if underlying parenchymal or glomerular renal disease is suspected (114).
Future techniques try to achieve the ability to rapidly and accurately measure and monitor GFR in real time (115). Optical measurement techniques using minimally invasive or non-invasive techniques that can quantify renal function independent of serum creatinine or urine output are under research (115). In the past few years, some progress has been made in using two-photon excitation fluorescence microscopy to study kidney function (116). It is likely that some of these approaches will enter clinical phase studies in the near future. In the best way, these techniques will enable an earlier diagnosis of AKI and also provide opportunities to improve clinical management, including the use of nephrotoxic substances and appropriate drug dosing (116).
Furthermore, new imaging techniques such as cine phase-contrast magnetic resonance imaging or intra-vital multiphoton studies may be used in AKI detection. However, based on the complexity, financial costs and need for patient transport, it is likely that these methods will remain research tools (117).
Author Contributions
WH coordinated the review. WH, RZ, and TL drafted the manuscript. GS and RS also participated in the analysis of the data and helped to draft the manuscript.
Conflict of Interest Statement
WH collaborates with Pulsion Medical Systems SE, Feldkirchen, Germany as member of the Medical Advisory Board.
The remaining authors declare that the research was conducted in the absence of any commercial or financial relationships that could be construed as a potential conflict of interest.
References
1. Dünser MW, Takala J, Brunauer A, Bakker J. Re-thinking resuscitation: leaving blood pressure cosmetics behind and moving forward to permissive hypotension and a tissue perfusion-based approach. Crit Care. (2013) 17:326. doi: 10.1186/cc12727
2. Ince C. Personalized physiological medicine. Crit Care. (2017) 21(Suppl 3):308. doi: 10.1186/s13054-017-1907-7
3. Secomb TW, Pries AR. The microcirculation: physiology at the mesoscale. J Physiol. (2011) 589(Pt 5):1047–52. doi: 10.1113/jphysiol.2010.201541
4. Cecconi M, De Backer D, Antonelli M, Beale R, Bakker J, Hofer C, et al. Consensus on circulatory shock and hemodynamic monitoring. Task force of the European Society of Intensive Care Medicine. Intens Care Med. (2014) 40:1795–815. doi: 10.1007/s00134-014-3525-z
5. Singer M, Deutschman CS, Seymour CW, Shankar-Hari M, Annane D, Bauer M, et al. The Third International consensus definitions for sepsis and septic shock (Sepsis-3). JAMA. (2016) 315:801–10. doi: 10.1001/jama.2016.0287
6. Rivers E, Nguyen B, Jaehne AK, Jayaprakash N, Semler MW, Hegab S, et al. Early goal-directed therapy in the treatment of severe sepsis and septic shock. N Engl J Med. (2001) 345:1368–77. doi: 10.1056/NEJMoa010307
7. Herner A, Haller B, Mayr U, Rasch S, Offman L, Schmid R, et al. Accuracy and precision of ScvO2 measured with the CeVOX-device: a prospective study in patients with a wide variation of ScvO2-values. PLoS ONE. (2018) 13:e0192073. doi: 10.1371/journal.pone.0192073
8. Molnar Z, Umgelter A, Toth I, Livingstone D, Weyland A, Sakka SG, et al. Continuous monitoring of ScvO(2) by a new fibre-optic technology compared with blood gas oximetry in critically ill patients: a multicentre study. Intens Care Med. (2007) 33:1767–70. doi: 10.1007/s00134-007-0743-7
9. Jones AE, Shapiro NI, Trzeciak S, Arnold RC, Claremont HA, Kline JA, et al. Lactate clearance vs central venous oxygen saturation as goals of early sepsis therapy: a randomized clinical trial. JAMA. (2010) 303:739–46. doi: 10.1001/jama.2010.158
10. Angus DC, Barnato AE, Bell D, Bellomo R, Chong CR, Coats TJ, et al. A systematic review and meta-analysis of early goal-directed therapy for septic shock: the ARISE, ProCESS and ProMISe Investigators. Intens Care Med. (2015) 41:1549–60. doi: 10.1007/s00134-015-3822-1
11. Vallee F, Vallet B, Mathe O, Parraguette J, Mari A, Silva S, et al. Central venous-to-arterial carbon dioxide difference: an additional target for goal-directed therapy in septic shock? Intens Care Med. (2008) 34:2218–25. doi: 10.1007/s00134-008-1199-0
12. Hernandez G, Castro R, Romero C, de la Hoz C, Angulo D, Aranguiz I, et al. Persistent sepsis-induced hypotension without hyperlactatemia: is it really septic shock? J Crit Care. (2011) 26:435.e9–e14. doi: 10.1016/j.jcrc.2010.09.007
13. Vallet B, Pinsky MR, Cecconi M. Resuscitation of patients with septic shock: please mind the gap! Intens Care Med. (2013) 39:1653–5. doi: 10.1007/s00134-013-2998-5
14. Kiyatkin ME, Bakker J. Lactate and microcirculation as suitable targets for hemodynamic optimization in resuscitation of circulatory shock. Curr Opin Crit Care. (2017) 23:348–54. doi: 10.1097/MCC.0000000000000423
15. Wacharasint P, Nakada TA, Boyd JH, Russell JA, and Walley KR. Normal-range blood lactate concentration in septic shock is prognostic and predictive. Shock. (2012) 38:4–10. doi: 10.1097/SHK.0b013e318254d41a
16. Jansen TC, van Bommel J, Schoonderbeek FJ, Sleeswijk Visser SJ, van der Klooster JM, Lima AP, et al. Early lactate-guided therapy in intensive care unit patients: a multicenter, open-label, randomized controlled trial. Am J Respir Crit Care Med. (2010) 182:752–61. doi: 10.1164/rccm.200912-1918OC
17. Leopold JH, van Hooijdonk RTM, Boshuizen M, Winters T, Bos LD, Abu-Hanna A, et al. Point and trend accuracy of a continuous intravenous microdialysis-based glucose-monitoring device in critically ill patients: a prospective study. Ann Intens Care. (2016) 6:68. doi: 10.1186/s13613-016-0171-3
18. Joly HR, Weil MH. Temperature of the great toe as an indication of the severity of shock. Circulation. (1969) 39:131–8. doi: 10.1161/01.CIR.39.1.131
19. Ait-Oufella H, Lemoinne S, Boelle PY, Galbois A, Baudel JL, Lemant J, et al. Mottling score predicts survival in septic shock. Intens Care Med. (2011) 37:801–7. doi: 10.1007/s00134-011-2163-y
20. Beecher HK, Simeone FA, Burnett CH, Shapiro SL, Sullivan ER, Mallory TB. The internal state of the severely wounded man on entry to the most forward hospital. Surgery. (1947) 22:672–711.
21. Tibby SM, Hatherill M, Murdoch IA. Capillary refill and core-peripheral temperature gap as indicators of haemodynamic status in paediatric intensive care patients. Arch Dis Child. (1999) 80:163–6. doi: 10.1136/adc.80.2.163
22. Dubin A, Henriquez E, Hernández G. Monitoring peripheral perfusion and microcirculation. Curr Opin Crit Care. (2018) 24:173–80. doi: 10.1097/MCC.0000000000000495
23. Felder D, Russ E, Montgomery H, Horwitz O. Relationship in the toe of skin surface temperature to mean blood flow measured with a plethysmograph. Clin Sci. (1954) 13:251–6.
24. Ibsen B. Treatment of shock with vasodilators measuring skin temperature on the big toe. Ten years' experience in 150 cases. Dis Chest. (1967) 52:425–9. doi: 10.1378/chest.52.4.425
25. Henning RJ, Wiener F, Valdes S, Weil MH. Measurement of toe temperature for assessing the severity of acute circulatory failure. Surg Gynecol Obstet. (1979) 149:1–7.
26. Vincent JL, Moraine JJ, van der Linden P. Toe temperature versus transcutaneous oxygen tension monitoring during acute circulatory failure. Intens Care Med. (1988) 14:64–8. doi: 10.1007/BF00254125
27. Lima A, Jansen TC, van Bommel J, Ince C, Bakker J. The prognostic value of the subjective assessment of peripheral perfusion in critically ill patients. Crit Care Med. (2009) 37:934–8. doi: 10.1097/CCM.0b013e31819869db
28. Bourcier S, Pichereau C, Boelle PY, Nemlaghi S, Dubée V, Lejour G, et al. Toe-to-room temperature gradient correlates with tissue perfusion and predicts outcome in selected critically ill patients with severe infections. Ann Intens Care. (2016) 6:63. doi: 10.1186/s13613-016-0164-2
29. Pereira CB, Czaplik M, Blanik N, Rossaint R, Blazek V, Leonhardt S. Contact-free monitoring of circulation and perfusion dynamics based on the analysis of thermal imagery. Biomed Opt Express. (2014) 5:1075–89. doi: 10.1364/BOE.5.001075
30. Ait-Oufella H, Bourcier S, Alves M, Galbois A, Baudel JL, Margetis D, et al. Alteration of skin perfusion in mottling area during septic shock. Ann Intens Care. (2013) 3:31. doi: 10.1186/2110-5820-3-31
31. Ait-Oufella H, Bakker J. Understanding clinical signs of poor tissue perfusion during septic shock. Intens Care Med. (2016) 42:2070–2. doi: 10.1007/s00134-016-4250-6
32. Coudroy R, Jamet A, Frat JP, Veinstein A, Chatellier D, Goudet V, et al. Incidence and impact of skin mottling over the knee and its duration on outcome in critically ill patients. Intens Care Med. (2015) 41:452–9. doi: 10.1007/s00134-014-3600-5
33. de Moura EB, Amorim FF, da Cruz Santana AN, Kanhouche G, de Souza Godoy LG, de Jesus Almeida L, et al. Skin mottling score as a predictor of 28-day mortality in patients with septic shock. Intens Care Med. (2016) 42:479–80. doi: 10.1007/s00134-015-4184-4
34. Galbois A, Bigé N, Pichereau C, Boëlle PY, Baudel JL, Bourcier S, et al. Exploration of skin perfusion in cirrhotic patients with septic shock. J Hepatol. (2015) 62:549–55. doi: 10.1016/j.jhep.2014.10.012
35. Schriger DL, Baraff L. Defining normal capillary refill: variation with age, sex, and temperature. Ann Emerg Med. (1988) 17:932–5. doi: 10.1016/S0196-0644(88)80675-9
36. Tafner PFDA, Chen FK, Rabello R, Corrêa TD, Chaves RCF, Serpa A. Recent advances in bedside microcirculation assessment in critically ill patients. Rev Bras Ter Intens. (2017) 29:238–47. doi: 10.5935/0103-507X.20170033
37. Hernandez G, Luengo C, Bruhn A, Kattan E, Friedman G, Ospina-Tascon GA, et al. When to stop septic shock resuscitation: clues from a dynamic perfusion monitoring. Ann Intensive Care. (2014) 4:30. doi: 10.1186/s13613-014-0030-z
38. van Genderen ME, Paauwe J, de Jonge J, van der Valk RJ, Lima A, Bakker J, et al. Clinical assessment of peripheral perfusion to predict postoperative complications after major abdominal surgery early: a prospective observational study in adults. Crit Care. (2014) 18:R114. doi: 10.1186/cc13905
39. Espinoza ED, Welsh S, Dubin A. Lack of agreement between different observers and methods in the measurement of capillary refill time in healthy volunteers: an observational study. Rev Bras Ter Intens. (2014) 26:269–76. doi: 10.5935/0103-507X.20140038
40. Alsma J, van Saase J, Nanayakkara PWB, Schouten WEMI, Baten A, Bauer MP, et al. The power of flash mob research: conducting a Nationwide observational clinical study on capillary refill time in a single day. Chest. (2017) 151:1106–13. doi: 10.1016/j.chest.2016.11.035
41. Hernandez G, Pedreros C, Veas E, Bruhn A, Romero C, Rovegno M, et al. Evolution of peripheral vs metabolic perfusion parameters during septic shock resuscitation. A clinical-physiologic study. J Crit Care. (2012) 27:283–8. doi: 10.1016/j.jcrc.2011.05.024
42. Lara B, Enberg L, Ortega M, Leon P, Kripper C, Aguilera P, et al. Capillary refill time during fluid resuscitation in patients with sepsis-related hyperlactatemia at the emergency department is related to mortality. PLoS ONE. (2017) 12:e0188548. doi: 10.1371/journal.pone.0188548
43. Brunauer A, Koköfer A, Bataar O, Gradwohl-Matis I, Dankl D, Bakker J, et al. Changes in peripheral perfusion relate to visceral organ perfusion in early septic shock: a pilot study. J Crit Care. (2016) 35:105–9. doi: 10.1016/j.jcrc.2016.05.007
44. van Genderen ME, Engels N, van der Valk RJ, Lima A, Klijn E, Bakker J, et al. Early peripheral perfusion-guided fluid therapy in patients with septic shock. Am J Respir Crit Care Med. (2015) 191:477–80. doi: 10.1164/rccm.201408-1575LE
45. Matsukawa T, Ozaki M, Nishiyama T, Imamura M, Kumazawa T. Comparison of infrared thermometer with thermocouple for monitoring skin temperature. Crit Care Med. (2000) 28:532–6. doi: 10.1097/00003246-200002000-00041
46. Ince C, Boerma EC, Cecconi M, De Backer D, Shapiro NI, Duranteau J, et al. Second consensus on the assessment of sublingual microcirculation in critically ill patients: results from a task force of the European Society of Intensive Care Medicine. Intens Care Med. (2018) 44:281–99. doi: 10.1007/s00134-018-5070-7
47. Ospina-Tascon G, Neves AP, Occhipinti G, Donadello K, Büchele G, Simion D, et al. Effects of fluids on microvascular perfusion in patients with severe sepsis. Intens Care Med. (2010) 36:949–55. doi: 10.1007/s00134-010-1843-3
48. Aykut G, Veenstra G, Scorcella C, Ince C, Boerma C. Cytocam-IDF (incident dark field illumination) imaging for bedside monitoring of the microcirculation. Intens Care Med Exp. (2015) 3:40. doi: 10.1186/s40635-015-0040-7
49. Lima AP, Beelen P, Bakker J. Use of a peripheral perfusion index derived from the pulse oximetry signal as a noninvasive indicator of perfusion. Crit Care Med. (2002) 30:1210–3. doi: 10.1097/00003246-200206000-00006
50. Duggappa DR, Lokesh M, Dixit A, Paul R, Raghavendra Rao RS, Prabha P. Perfusion index as a predictor of hypotension following spinal anaesthesia in lower segment caesarean section. Indian J Anaesth. (2017) 61:649–54. doi: 10.4103/ija.IJA_429_16
51. He HW, Liu DW, Long Y, Wang XT. The peripheral perfusion index and transcutaneous oxygen challenge test are predictive of mortality in septic patients after resuscitation. Crit Care. (2013) 17:R116. doi: 10.1186/cc12788
52. Vesterager P. Transcutaneous pO2 electrode. Scand J Clin Lab Invest Suppl. (1977) 146:27–30. doi: 10.3109/00365517709098929
53. Vesterager P. Effect of electrode temperatures on monitoring of transcutaneous carbon dioxide (TcPCO2) in prematures. Biotelem Patient Monit. (1982) 9:18–27.
54. Lima A. Current status of tissue monitoring in the management of shock. Curr Opin Crit Care. (2016) 22:274–8. doi: 10.1097/MCC.0000000000000300
55. Gomez H, Torres A, Polanco P, Kim HK, Zenker S, Puyana JC, et al. Use of non-invasive NIRS during a vascular occlusion test to assess dynamic tissue O(2) saturation response. Intens Care Med. (2008) 34:1600–7. doi: 10.1007/s00134-008-1145-1
56. Gómez H, Mesquida J, Simon P, Kim HK, Puyana JC, Ince C, et al. Characterization of tissue oxygen saturation and the vascular occlusion test: influence of measurement sites, probe sizes and deflation thresholds. Crit Care. (2009) 13(Suppl 5):S3. doi: 10.1186/cc8001
57. Lipcsey M, Woinarski NC, Bellomo R. Near infrared spectroscopy (NIRS) of the thenar eminence in anesthesia and intensive care. Ann Intens Care. (2012) 2:11. doi: 10.1186/2110-5820-2-11
58. Bezemer R, Bartels SA, Bakker J, Ince C. Clinical review: clinical imaging of the sublingual microcirculation in the critically ill–where do we stand? Crit Care. (2012) 16:224. doi: 10.1186/cc11236
59. Groner W, Winkelman JW, Harris AG, Ince C, Bouma GJ, Messmer K, et al. Orthogonal polarization spectral imaging: a new method for study of the microcirculation. Nat Med. (1999) 5:1209–12. doi: 10.1038/13529
60. Hernandez G, Boerma EC, Dubin A, Bruhn A, Koopmans M, Edul VK, et al. Severe abnormalities in microvascular perfused vessel density are associated to organ dysfunctions and mortality and can be predicted by hyperlactatemia and norepinephrine requirements in septic shock patients. J Crit Care. (2013) 28:538.e9–e14. doi: 10.1016/j.jcrc.2012.11.022
61. Kanoore Edul VS, Ince C, Dubin A. What is microcirculatory shock? Curr Opin Crit Care. (2015) 21:245–52. doi: 10.1097/MCC.0000000000000196
62. Vellinga NA, Boerma EC, Koopmans M, Donati A, Dubin A, Shapiro NI, et al. International study on microcirculatory shock occurrence in acutely ill patients. Crit Care Med. (2015) 43:48–56. doi: 10.1097/CCM.0000000000000553
63. Reynolds T, Vivian-Smith A, Jhanji S, Pearse RM. Observational study of the effects of age, diabetes mellitus, cirrhosis and chronic kidney disease on sublingual microvascular flow. Perioper Med. (2013) 2:7. doi: 10.1186/2047-0525-2-7
64. Kanoore Edul VS, Ince C, Estenssoro E, Ferrara G, Arzani Y, Salvatori C, et al. The Effects of arterial hypertension and age on the sublingual microcirculation of healthy volunteers and outpatients with cardiovascular risk factors. Microcirculation. (2015) 22:485–92. doi: 10.1111/micc.12219
65. Massey MJ, Larochelle E, Najarro G, Karmacharla A, Arnold R, Trzeciak S, et al. The microcirculation image quality score: development and preliminary evaluation of a proposed approach to grading quality of image acquisition for bedside videomicroscopy. J Crit Care. (2013) 28:913–7. doi: 10.1016/j.jcrc.2013.06.015
66. Arnemann PH, Hessler M, Kampmeier T, Morelli A, Van Aken HK, Westphal M, et al. Comparison of an automatic analysis and a manual analysis of conjunctival microcirculation in a sheep model of haemorrhagic shock. Intens Care Med Exp. (2016) 4:37. doi: 10.1186/s40635-016-0110-5
67. Carsetti A, Aya HD, Pierantozzi S, Bazurro S, Donati A, Rhodes A, et al. Ability and efficiency of an automatic analysis software to measure microvascular parameters. J Clin Monit Comput. (2017) 31:669–76. doi: 10.1007/s10877-016-9928-3
68. Massey MJ, Shapiro NI. A guide to human in vivo microcirculatory flow image analysis. Crit Care. (2016) 20:35. doi: 10.1186/s13054-016-1213-9
69. Legrand M, Ait-Oufella H. Could resuscitation be based on microcirculation data? Yes. Intens Care Med. (2018) 2018:10. doi: 10.1007/s00134-018-5121-0
70. Naumann DN, Lima A. Could resuscitation be based on microcirculation data? No. Intens Care Med. (2018) 2018:5095. doi: 10.1007/s00134-018-5095-y
71. Klijn E, van Velzen MH, Lima AP, Bakker J, van Bommel J, Groeneveld AB. Tissue perfusion and oxygenation to monitor fluid responsiveness in critically ill, septic patients after initial resuscitation: a prospective observational study. J Clin Monit Comput. (2015) 29:707–12. doi: 10.1007/s10877-014-9653-8
72. Duret J, Pottecher J, Bouzat P, Brun J, Harrois A, Payen JF, et al. Skeletal muscle oxygenation in severe trauma patients during haemorrhagic shock resuscitation. Crit Care. (2015) 19:141. doi: 10.1186/s13054-015-0854-4
73. D'Andrea A, Conte M, Scarafile R, Riegler L, Cocchia R, Pezzullo E, et al. Transcranial Doppler ultrasound: physical principles and principal applications in neurocritical care unit. J Cardiovasc Echogr. (2016) 26:28–41. doi: 10.4103/2211-4122.183746
74. Kahn RA, Slogoff FB, Reich DL, Konstadt SN. Transcranial Doppler ultrasonography: what is its role in cardiac and vascular surgical patients? J Cardiothorac Vasc Anesth. (1995) 9:589–97. doi: 10.1016/S1053-0770(05)80148-X
75. Moritz S, Kasprzak P, Arlt M, Taeger K, Metz C. Accuracy of cerebral monitoring in detecting cerebral ischemia during carotid endarterectomy: a comparison of transcranial Doppler sonography, near-infrared spectroscopy, stump pressure, and somatosensory evoked potentials. Anesthesiology. (2007) 107:563–9. doi: 10.1097/01.anes.0000281894.69422.ff
76. Kirkpatrick PJ, Lam J, Al-Rawi P, Smielewski P, Czosnyka M. Defining thresholds for critical ischemia by using near-infrared spectroscopy in the adult brain. J Neurosurg. (1998) 89:389–94. doi: 10.3171/jns.1998.89.3.0389
77. Doblar DD. Intraoperative transcranial ultrasonic monitoring for cardiac and vascular surgery. Semin Cardiothorac Vasc Anesth. (2004) 8:127–45. doi: 10.1177/108925320400800206
78. Brazy JE, Lewis DV, Mitnick MH, Jöbsis vander Vliet FF. Noninvasive monitoring of cerebral oxygenation in preterm infants: preliminary observations. Pediatrics. (1985) 75:217–25.
79. Jöbsis FF. Noninvasive, infrared monitoring of cerebral and myocardial oxygen sufficiency and circulatory parameters. Science. (1977) 198:1264–67. doi: 10.1126/science.929199
80. Davie SN, Grocott HP. Impact of extracranial contamination on regional cerebral oxygen saturation: a comparison of three cerebral oximetry technologies. Anesthesiology. (2012) 116:834–40. doi: 10.1097/ALN.0b013e31824c00d7
81. Ito H, Kanno I, Fukuda H. Human cerebral circulation: positron emission tomography studies. Ann Nucl Med. (2005) 19:65–74. doi: 10.1007/BF03027383
82. Casati A, Fanelli G, Pietropaoli P, Proietti R, Tufano R, Danelli G, et al. Continuous monitoring of cerebral oxygen saturation in elderly patients undergoing major abdominal surgery minimizes brain exposure to potential hypoxia. Anesth Analg. (2005) 101:740–7. doi: 10.1213/01.ane.0000166974.96219.cd
83. Heringlake M, Garbers C, äbler JH, Anderson I, Heinze H, Schön J, et al. Preoperative cerebral oxygen saturation and clinical outcomes in cardiac surgery. Anesthesiology. (2011) 114:58–69. doi: 10.1097/ALN.0b013e3181fef34e
84. Paquet C, Deschamps A, Denault AY, Couture P, Carrier M, Babin D, et al. Baseline regional cerebral oxygen saturation correlates with left ventricular systolic and diastolic function. J Cardiothorac Vasc Anesth. (2008) 22:840–6. doi: 10.1053/j.jvca.2008.02.013
85. Apostolidou I, Morrissette G, Sarwar MF, Konia MR, Kshettry VR, Wahr JA, et al. Cerebral oximetry during cardiac surgery: the association between cerebral oxygen saturation and perioperative patient variables. J Cardiothorac Vasc Anesth. (2012) 26:1015–21. doi: 10.1053/j.jvca.2012.07.011
86. Edmonds HL Jr. Protective effect of neuromonitoring during cardiac surgery. Ann N Y Acad Sci. (2005) 1053:12–9. doi: 10.1196/annals.1344.002
87. Murkin JM, Adams SJ, Novick RJ, Quantz M, Bainbridge D, Iglesias I, et al. Monitoring brain oxygen saturation during coronary bypass surgery: a randomized, prospective study. Anesth Analg. (2007) 104:51–8. doi: 10.1213/01.ane.0000246814.29362.f4
88. Goldman S, Sutter F, Ferdinand F, Trace C. Optimizing intraoperative cerebral oxygen delivery using noninvasive cerebral oximetry decreases the incidence of stroke for cardiac surgical patients. Heart Surg Forum. (2004) 7:E376–81. doi: 10.1532/HSF98.20041062
89. Slater JP, Guarino T, Stack J, Vinod K, Bustami RT, Brown JM III, et al. Cerebral oxygen desaturation predicts cognitive decline and longer hospital stay after cardiac surgery. Ann Thorac Surg. (2009) 87:36–44; discussion 44–35. doi: 10.1016/j.athoracsur.2008.08.070
90. Mohandas BS, Jagadeesh AM, Vikram SB. Impact of monitoring cerebral oxygen saturation on the outcome of patients undergoing open heart surgery. Ann Card Anaesth. (2013) 16:102–6. doi: 10.4103/0971-9784.109740
91. Denault A, Deschamps A, Murkin JM. A proposed algorithm for the intraoperative use of cerebral near-infrared spectroscopy. Semin Cardiothorac Vasc Anesth. (2007) 11:274–81. doi: 10.1177/1089253207311685
92. Zipprich A, Kuss O, Rogowski S, Kleber G, Lotterer E, Seufferlein T, et al. Incorporating indocyanin green clearance into the Model for End Stage Liver Disease (MELD-ICG) improves prognostic accuracy in intermediate to advanced cirrhosis. Gut. (2010) 59:963–8. doi: 10.1136/gut.2010.208595
93. Sakka SG. Assessment of liver perfusion and function by indocyanine green in the perioperative setting and in critically ill patients. J Clin Monit Comput. (2017) 32:787–96. doi: 10.1007/s10877-017-0073-4
94. Sakka SG. Assessing liver function. Curr Opin Crit Care. (2007) 13:207–14. doi: 10.1097/MCC.0b013e328012b268
95. Braden B, Faust D, Sarrazin U, Zeuzem S, Dietrich CF, Caspary WF, et al. 13C-methacetin breath test as liver function test in patients with chronic hepatitis C virus infection. Aliment Pharmacol Ther. (2005) 21:179–85. doi: 10.1111/j.1365-2036.2005.02317.x
96. Holtmeier J, Leuschner M, Schneider A, Leuschner U, Caspary WF, Braden B. 13C-methacetin and 13C-galactose breath tests can assess restricted liver function even in early stages of primary biliary cirrhosis. Scand J Gastroenterol. (2006) 41:1336–41. doi: 10.1080/00365520600670125
97. Schneider A, Caspary WF, Saich R, Dietrich CF, Sarrazin C, Kuker W, et al. 13C-methacetin breath test shortened: 2-point-measurements after 15 minutes reliably indicate the presence of liver cirrhosis. J Clin Gastroenterol. (2007) 41:33–7. doi: 10.1097/MCG.0b013e31802dd4b9
98. Dinesen L, Caspary WF, Chapman RW, Dietrich CF, Sarrazin C, Braden B. 13C-methacetin-breath test compared to also noninvasive biochemical blood tests in predicting hepatic fibrosis and cirrhosis in chronic hepatitis C. Dig Liver Dis. (2008) 40:743–8. doi: 10.1016/j.dld.2008.01.013
99. Jara M, Malinowski M, Lüttgert K, Schott E, Neuhaus P, Stockmann M. Prognostic value of enzymatic liver function for the estimation of short-term survival of liver transplant candidates: a prospective study with the LiMAx test. Transpl Int. (2015) 28:52–8. doi: 10.1111/tri.12441
100. Joannidis M, Druml W, Forni LG, Groeneveld ABJ, Honore PM, Hoste E, et al. Prevention of acute kidney injury and protection of renal function in the intensive care unit. Expert opinion of the Working Group for Nephrology, ESICM. Intens Care Med. (2010) 36:392–411. doi: 10.1007/s00134-009-1678-y
101. Metnitz PG, Krenn CG, Steltzer H, Lang T, Ploder J, Lenz K, et al. Effect of acute renal failure requiring renal replacement therapy on outcome in critically ill patients. Crit Care Med. (2002) 30:2051–8. doi: 10.1097/00003246-200209000-00016
102. Uchino S, Kellum JA, Bellomo R, Doig GS, Morimatsu H, Morgera S, et al. Acute renal failure in critically ill patients: a multinational, multicenter study. JAMA. (2005) 294:813–8. doi: 10.1001/jama.294.7.813
103. Bellomo R, Kellum JA, Ronco C, Wald R, Martensson J, Maiden M, et al. Acute kidney injury in sepsis. Intens Care Med. (2017) 43:816–28. doi: 10.1007/s00134-017-4755-7
104. Kellum JA, Lameire N. Diagnosis, evaluation, and management of acute kidney injury: a KDIGO summary (Part 1). Crit Care. (2013) 17:204. doi: 10.1186/cc11454
105. Schrier RW, Wang W. Acute renal failure and sepsis. N Engl J Med. (2004) 351:159–69. doi: 10.1056/NEJMra032401
106. Lameire N, Van Biesen W, et al. Acute renal failure. Lancet. (2005) 365:417–30. doi: 10.1016/S0140-6736(05)70238-5
107. Ostermann M, Joannidis M. Acute kidney injury 2016: diagnosis and diagnostic workup. Crit Care. (2016) 20:299. doi: 10.1186/s13054-016-1478-z
108. Murray PT, Mehta RL, Shaw A, Ronco C, Endre Z, Kellum JA, et al. Potential use of biomarkers in acute kidney injury: report and summary of recommendations from the 10th Acute Dialysis Quality Initiative consensus conference. Kidney Int. (2014) 85:513–21. doi: 10.1038/ki.2013.374
109. Haase M, Devarajan P, Haase-Fielitz A, Bellomo R, Cruz DN, Wagener G, et al. The outcome of neutrophil gelatinase-associated lipocalin-positive subclinical acute kidney injury: a multicenter pooled analysis of prospective studies. J Am Coll Cardiol. (2011) 57:1752–761. doi: 10.1016/j.jacc.2010.11.051
110. Honore PM, Jacobs R, Hendrickx I. Biomarkers in critical illness: have we made progress? Int J Nephrol Renovasc Dis. (2016) 9:253–6. doi: 10.2147/IJNRD.S113219
111. Schnell D, Camous L, Guyomarc'h S, Duranteau J, Canet E, Gery P, et al. Renal perfusion assessment by renal Doppler during fluid challenge in sepsis. Crit Care Med. (2013) 41:1214–20. doi: 10.1097/CCM.0b013e31827c0a36
112. Lahmer T, Rasch S, Schnappauf C, Schmid RM, Huber W. Influence of volume administration on Doppler-based renal resistive index, renal hemodynamics and renal function in medical intensive care unit patients with septic-induced acute kidney injury: a pilot study. Int Urol Nephrol. (2016) 48:1327–34. doi: 10.1007/s11255-016-1312-1
113. Lerolle N, Guérot E, Faisy C, Bornstain C, Diehl JL, Fagon JY. Renal failure in septic shock: predictive value of Doppler-based renal arterial resistive index. Intens Care Med. (2006) 32:1553–9. doi: 10.1007/s00134-006-0360-x
114. Augusto JF, Lassalle V, Fillatre P, Perrotin D, Meziani F, Schenck-Dhif M, et al. Safety and diagnostic yield of renal biopsy in the intensive care unit. Intens Care Med. (2012) 38:1826–33. doi: 10.1007/s00134-012-2634-9
115. Molitoris BA, Reilly ES. Quantifying glomerular filtration rates in acute kidney injury: a requirement for translational success. Semin Nephrol. (2016) 36:31–41. doi: 10.1016/j.semnephrol.2016.01.008
116. Prowle JR, Molan MP, Hornsey E, Bellomo R. Cine phase-contrast magnetic resonance imaging for the measurement of renal blood flow. Contrib Nephrol. (2010) 165:329–36. doi: 10.1159/000313774
Keywords: liver function, neuromonitoring, capillary refill time, body surface temperature, near infra-red spectroscopy, hemodynamic monitoring, renal failure, mottling score
Citation: Huber W, Zanner R, Schneider G, Schmid R and Lahmer T (2019) Assessment of Regional Perfusion and Organ Function: Less and Non-invasive Techniques. Front. Med. 6:50. doi: 10.3389/fmed.2019.00050
Received: 23 March 2018; Accepted: 25 February 2019;
Published: 22 March 2019.
Edited by:
Samir G. Sakka, Universität Witten/Herdecke, GermanyReviewed by:
Inge Bauer, Universitätsklinikum Düsseldorf, GermanyAlexander Koch, Uniklinik RWTH Aachen, Germany
Copyright © 2019 Huber, Zanner, Schneider, Schmid and Lahmer. This is an open-access article distributed under the terms of the Creative Commons Attribution License (CC BY). The use, distribution or reproduction in other forums is permitted, provided the original author(s) and the copyright owner(s) are credited and that the original publication in this journal is cited, in accordance with accepted academic practice. No use, distribution or reproduction is permitted which does not comply with these terms.
*Correspondence: Wolfgang Huber, V29sZmdhbmcuSHViZXJAdHVtLmRl