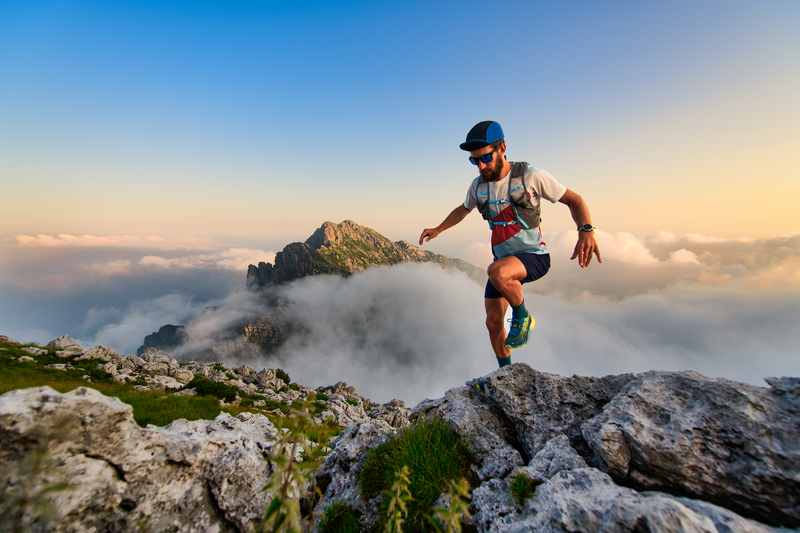
95% of researchers rate our articles as excellent or good
Learn more about the work of our research integrity team to safeguard the quality of each article we publish.
Find out more
REVIEW article
Front. Med. , 13 February 2018
Sec. Infectious Diseases: Pathogenesis and Therapy
Volume 5 - 2018 | https://doi.org/10.3389/fmed.2018.00028
Candida species are fungal pathogens known for their ability to cause superficial and systemic infections in the human host. These pathogens are able to persist inside the host due to the development of pathogenicity and multidrug resistance traits, often leading to the failure of therapeutic strategies. One specific feature of Candida species pathogenicity is their ability to form biofilms, which protects them from external factors such as host immune system defenses and antifungal drugs. This review focuses on the current threats and challenges when dealing with biofilms formed by Candida albicans, Candida glabrata, Candida tropicalis, and Candida parapsilosis, highlighting the differences between the four species. Biofilm characteristics depend on the ability of each species to produce extracellular polymeric substances (EPS) and display dimorphic growth, but also on the biofilm substratum, carbon source availability and other factors. Additionally, the transcriptional control over processes like adhesion, biofilm formation, filamentation, and EPS production displays great complexity and diversity within pathogenic yeasts of the Candida genus. These differences not only have implications in the persistence of colonization and infections but also on antifungal resistance typically found in Candida biofilm cells, potentiated by EPS, that functions as a barrier to drug diffusion, and by the overexpression of drug resistance transporters. The ability to interact with different species in in vivo Candida biofilms is also a key factor to consider when dealing with this problem. Despite many challenges, the most promising strategies that are currently available or under development to limit biofilm formation or to eradicate mature biofilms are discussed.
A biofilm consists in a community of microorganisms that are irreversibly attached to a given surface, inert material, or living tissue, producing extracellular polymers that provide a structural matrix (1, 2). The microorganisms in this type of community exhibit lower growth rates and higher resistance to antimicrobial treatment, behaving very differently from planktonic cells (2). The ability to adhere to different types of surfaces enables microorganisms to form biofilm on medical devices, such as intravascular catheters, prosthetic heart valves and joint replacements, or in different tissues in the host (3), linking biofilms to persistent colonization and infections (4). A single microbial species is able to form biofilm although a mixture of bacterial and fungal species usually underlies biofilm formation in vivo (3, 5). Given that 80% of all microorganisms live in this form, biofilm formation becomes an irrevocable field to explore (6).
Due to these general characteristics, biofilms potentiate the establishment of unyielding infections in the human host. This is the case of biofilms formed by Candida species, causing superficial and systemic fungal infections in immunocompromised patients (7). These infections are very difficult to treat due to the characteristics of these species: resistance to antifungal drugs, expression of virulence factors, and the ability to form biofilm. Indeed, mucosal infections involve biofilm formation (8), usually including the interaction with commensal bacterial flora and host components (9). Candida species are the fourth most common cause of nosocomial bloodstream infection in the United States. These infections are associated with a high mortality rate of approximately 50% (10, 11). Biofilms are an additional problem since they are usually found in medical devices, such as prostheses, cardioverter defibrillators, urinary and vascular catheters, and cardiac devices (12, 13), hindering the eradication of Candida infections.
These challenging infections may be caused by different Candida species. Candida albicans is the most frequent pathogen responsible for Candida infections, followed by Candida glabrata (14). Candida tropicalis is particularly relevant in urinary tract infections (15) while Candida parapsilosis is frequently found in the skin of healthy hosts, being the causative agent of catheter-related infections (16, 17). Each Candida species exhibits differences in terms of biofilm formation, namely at the level of their morphology, characteristics of the extracellular matrix (ECM), and ability to confer antifungal resistance (18). This variability increases the challenge of finding an effective solution to tackle the threats of Candida biofilms as a unique problem. In fact, due to the emergence of these fungal infections, there is an urgent need to find adequate therapeutic approaches that might be able to treat patients more efficiently. The path to find these therapeutics certainly involves the study of the different pathogenic features of these species such as biofilm formation.
Biofilm formation, although being a process present in all the Candida species focused above, differs significantly from species to species, and in the dependency of surface, host niche and other factors. For example, C. albicans mature biofilms exhibit a more heterogenous structure, composed by blastophores and hyphae surrounded by an ECM of polysaccharide material (19). The ECM provides structural scaffold for adhesion between cells and with different surfaces, and a barrier between the cells in the biofilm and the neighboring environment (20). Within the structure of these biofilms there is usually water channels surrounding the microcolonies (21). In the case of C. glabrata, the biofilm is exclusively composed by yeast form cells in a multilayer structure intimately packed or in clusters of cells (22). In turn, C. tropicalis biofilm corresponds to a network of yeast, pseudohyphae, and hyphae, with intense hyphal budding (23), while C. parapsilosis exhibits a biofilm formed by clusters of yeast cells adhered to the surface, with minimal ECM (24). These differences highlight the complexity of the processes underlying biofilm formation and the difficulty to find a unique way to eradicate all Candida biofilms. Candida biofilms occur mostly in the mucosa or endothelium being involved in the development of common candidiasis, such as vaginal and oral candidiasis, but also associated with medical devices, such as vascular and urinary catheters and dentures (25). Interestingly, however, in all cases, biofilm formation compromises antifungal treatment and, when occurring in implanted medical devices, such as central venous catheters, implant replacement is often required (6, 26).
This review highlights the main challenges found in the process of developing effective therapy against Candida biofilms, considering differences found in biofilm formation and its regulation, as well as antifungal resistance found in biofilms and the establishment of mixed-species biofilm. Currently proposed promising strategies are also discussed herein.
Biofilm formation is a multifaceted process well described for C. albicans. In this case, the early phase of biofilm formation starts with adhesion of yeast cells to a given surface, followed by the formation of a discrete colony. Afterwards, in the intermediate phase, cells become organized and start producing and secreting extracellular polymeric substances (EPS). These components allow the maturation of a three-dimensional structure, forming the biofilm as we know it, in the maturation phase. Once the mature biofilm is formed, there is still the possibility of dissemination of progeny biofilm cells that become detached migrating to other niches to form more biofilm (18, 27).
For C. albicans in vitro biofilm formation, the early phase takes approximately 11 h, leading to the formation of distinct microcolonies. The intermediate phase of biofilm formation may last for 12–30 h, being characterized by the production of EPS and the formation of a bilayer usually composed by yeast, germ tubes and/or young hyphae. After this phase, C. albicans biofilm maturation includes the development of a thick layer of EPS where yeasts, and hyphae are present, forming a dense network. The maturation process usually takes approximately 38–72 h (18, 19, 27). Following maturation comes the dispersal phase, where mature biofilms release budding daughter cells as non-adherent yeast cells to propagate the colonization/infection (28).
It is important to highlight that most studies on biofilm formation have been conducted in vitro. A good example of an in vivo study was that conducted by Andes et al. that followed the development of C. albicans biofilm in a rat central venous catheter (29). This study reported important differences in biofilm formation between in vivo and in vitro experiments. For instance, the duration of the early phase of biofilm formation in vivo was found to be smaller than that observed in vitro, with several layers of yeast cells and hyphae being already present after 8 h of in vivo biofilm formation (29). Furthermore, maturation of the C. albicans biofilm in vivo was observed at 24 h in the rat central venous catheter and in an avascular implantation of small catheter in rats, contrary to the 38–72 h observed in vitro (29, 30). In the case of C. glabrata, in vivo maturation of the biofilm was only observed after 48 h in serum-coated triple-lumen catheters in a rat subcutaneous model. Although the early phase was similar between in vivo and in vitro conditions, after 24 h in vitro biofilms present a confluent layer of yeast cells while in vivo biofilms only exhibit patches of aggregated yeast cells. Moreover, in vivo C. glabrata biofilm development is dependent of the number of cells attached to the catheter, while in vitro it is not. The thickness of C. glabrata biofilm, 75–90 + 5 µm, is approximately half of the thickness of C. albicans biofilm (31).
The first crucial step of biofilm formation is adhesion. This process relies on several cell wall proteins, called adhesins, that promote the attachment to other cells, both to epithelial and to other microbial cells, or abiotic surfaces by binding to specific amino acid or sugar residues. Generally, adhesins are glycosyl-phosphatidylinositol-cell wall proteins (GPI-CWPs), comprising a GPI anchor, a serine/threonine domain and a carbohydrate or peptide binding domain (32). In C. albicans, several adhesins belong to the Als (agglutinin-like sequence) family, which is part of the GPI_CWP family. Such adhesins are known to bind to several proteins through its C-terminal region. Among the 8 members of the Als family, Als3 has the most prominent role in biofilm formation, as its deletion leads to severe biofilm defects when compared to the wild-type parental strain (33). ALS-like proteins have also been described to be present in other Candida species, such as C. parapsilosis, C. tropicalis, C. dubliniensis, C. lusitaniae, and C. guilliermondii (34), although still very little is known about the role of these adhesins in each species. Another important family of adhesins in C. albicans is the hyphal wall protein (Hwp) family, including Hwp1. Hwp1 is a mannoprotein of the cell wall of germ-tubes and hyphal cells, with a role in biofilm formation (35, 36). Besides Hwp1, also four other proteins in the Hwp family are required for biofilm development: Hwp2, Rbt1, Eap1, and Ywp1 (34). In C. glabrata, the EPA (epithelial adhesion) family of adhesins is the main responsible for adhesion. Most of these are encoded by genes localized in subtelomeric regions, including the EPA1, EPA2, EPA3, and HYR1 cluster, and the EPA4 and EPA5 cluster (37). Although the EPA family in C. glabrata is predicted to comprise 17–23 genes, EPA1, EPA6, and EPA7 genes are described as the most important in adhesion (35).
After adhesion, biofilm development continues through morphologic modifications, increase in cell number and production of EPS, influencing the final biofilm architecture. Analysis of the development of biofilm and production of ECM in Candida albicans, C. parapsilosis, C. tropicalis, and C. glabrata isolates of different origins have highlighted the differences in biofilm formation between species. Biofilms of C. albicans are more confluent than other Candida species biofilms (18), exhibiting different morphologic forms: oval budding, continuous septate hyphae and pseudohyphae, in infected tissues (3). On the surface of plastic coverslips, C. albicans biofilms exhibit a dense network of yeasts and filamentous cells embedded in a matrix of exopolymeric material (38). C. albicans is also considered to be the biggest biofilm producer among the Candida species focused herein (39). C. glabrata biofilms exhibit an ECM with high levels of carbohydrates and proteins (22), while isolates from the same species were observed to exhibit a scant population of blastospores, in silicone elastomer sheets (39). On the other hand, C. parapsilosis biofilm structure may vary according to the strain in study but usually comprises yeast and pseudohyphae morphologies, producing a compact multilayer or non-contiguous cell aggregates. The ECM of C. parapsilosis biofilms has been shown to be mainly composed by carbohydrates with low levels of proteins (22). Nevertheless, other evidences show that C. parapsilosis biofilms are the ones with less ECM produced when compared to C. albicans, C. glabrata, and C. tropicalis biofilms (39). Regarding C. tropicalis, a biofilm formation analysis revealed a structure composed mainly by yeast shaped cells, although some strains have exhibited filamentous forms in thick biofilms of coaggregated cells or in a discontinuous monolayer of yeasts anchored to the surface (22, 23). One specific isolate was shown to produce biofilm with a thin layer of matrix-encased hyphae (39). Although C. tropicalis biofilms have an ECM with a low content of carbohydrates and proteins (22), they are more resistant to detachment of the surface than those formed by C. albicans (40). When comparing biofilm production by C. albicans, C. parapsilosis, and C. tropicalis invasive isolates and C. glabrata blood isolates with non-invasive isolates, a significant higher production is observed in first isolates for all species, except for C. tropicalis (39).
Formed biofilms are also dependent on the EPS that are produced, which give a gel-like hydrated three-dimensional structure to the biofilm where the cells become partially immobilized (41). EPS plays different roles such as defense against phagocytosis, scaffold for biofilm integrity and prevention of drug diffusion. The production of EPS is dependent on the species and strain the carbon source and the rate of medium flow. For instance, it is known that C. tropicalis has a higher production of EPS matrix than C. glabrata. On the other hand, the increase of flow rate of the medium has shown to increase significantly the formation of the matrix (40). Usually, the major component of Candida biofilm EPS are polysaccharides (nearly 40%), although some differences are observed according to each Candida species (19, 40). In Candida albicans and C. tropicalis, the major carbohydrates present in the biofilm are glucose and hexosamine (39.6 and 27.4%, respectively). Proteins, phosphorus, and uronic acid may also be present but in small amounts (40). A biochemical analysis of C. albicans biofilm matrix in in vitro and in vivo models led to the identification of the following distribution of macromolecular components: 55% of proteins and their glycosylated counterparts, 25% of carbohydrates, 15% of lipids, and 5% of noncoding DNA. α-1,2-Branched α-1,6-mannans were the more abundant polysaccharides found associated with unbranched α-1,6-glucans, forming a mannan–glucan complex on the matrix (42). Although being relatively minor components of the ECM of C. albicans, β-1,3-glucan and β-1,6-glucan are also important components of this structure. Together with mannan, an interdependency between the synthesis or incorporation of these compounds seems to take place in the ECM since the inhibition of one of these three components leads to the decrease of the concentration of the others. Moreover, mannan and β-1,6-glucan, seem to be essential for ECM formation since the single deletion of genes encoding proteins involved in their production (ALG11, MNN9, MNN11, VAN1, MNN4-4, PMR1, and VRG4 for mannan production and BIG1 and KRE5 for β-1,6-glucan production) nearly led to the complete elimination of the biofilm matrix (43). The majority of the identified lipids were neutral glycerolipids, polar glycerolipids and, in a smaller percentage, sphingolipids (42).
Candida biofilms have thus a variety of possible architectures, adhesion properties, cellular morphologies and EPS composition, as illustrated in Figure 1, which are, not only species-, but in some cases strain-dependent, that turn the process of fighting these structures clinically very difficult. To increase the complexity of the problem, several other external factors related to the environment surrounding the biofilm also influence the final biofilm produced.
Figure 1. Comparative schematics the three stages of biofilm formation by Candida albicans, Candida glabrata, Candida tropicalis, and Candida parapsilosis, highlighting the different capacities to produce extracellular matrix (ECM), the varying components present in the ECM, and the ability to exhibit different cell morphologies.
Each step of biofilm formation depends on the environmental conditions experienced by Candida species (Table 1).
One important factor is the substratum to which the biofilm is attached. There are several options of substratum used to study Candida biofilms in vitro: polystyrene (44), polymethylmethacrylate (19), polyvinyl chloride (45), cellulose cylindrical filters (45), acrylic (46), and denture base materials (47) have been described for C. albicans biofilm formation, while silicone elastomer catheter disks have been tested for different Candida species (19, 48). Among these, hydrophobic surfaces lead to the formation of two phases in C. albicans biofilm. The first phase corresponds to the adhesion of blastospores to the surface and a second phase where hyphae elements are embedded in EPS with water channels that allow the diffusion of nutrients and passage of waste disposal between the bottom layer and the surface (19). On the other hand, substratum may influence the adhesion process itself, e.g., soft lining materials of dentures lead to higher adhesion when compared to acrylic surfaces (49). It also influences the size of the developed Candida biofilm (47). C. albicans biofilms are larger in latex and silicone elastomer surfaces, and smaller in polyurethane and on silicone, when compared to polyvinyl chloride (48). Chandra et al. have tested the effect of different modifications on the surface of biomaterials on the formation of C. albicans biofilms. This work has led to the discovery that polyetherurethane with 6% of polyethylene oxide significantly reduces the total biofilm biomass, as well as the metabolic activity of the cells in the biofilm (50). In turn, Estivill et al. have studied the biofilm formation of C. albicans, C. glabrata, C. parapsilosis, C. tropicalis, and C. krusei on polyvinyl chloride, polyurethane, and Teflon. All species exhibited increased biofilm formation on Teflon, except for C. glabrata, for which the best surface for biofilm formation was found to be polyvinyl chloride. C. tropicalis showed the highest number of cells recovered from biofilm development in a polyurethane catheter, while on polyvinyl chloride catheter, C. parapsilosis was the one with the highest number of cells (51). These differences in preference for surface material, that again appear to vary in a species- and strain-dependent manner, highlight the importance of selecting the most adequate biomaterials to prevent or, at least limit, the propensity for the development of biofilm-seeded infections by Candida species.
Besides the substratum, a relevant factor influencing biofilm formation is the available carbon source. When comparing the number of cells per biofilm formed by C. albicans and C. glabrata, a clear increase is observed when glucose is used as the carbon source, comparatively to sucrose (52) or, in the case of C. albicans, also to galactose (44). The test of different concentrations of glucose, has revealed that even low concentrations of glucose induce C. glabrata biofilm (53). Moreover, the medium used to evaluate biofilm formation in vitro has also shown to be responsible for differences in morphology. On a polymethylmethacrylate surface, C. albicans biofilm cells have been shown to predominantly include yeast shaped cells in YNB medium, and hyphal cells in RPMI 1640 medium (19). This indicates that biofilm formation does not require a given morphology, reinforcing the fact that biofilm development is intrinsically dependent on the environmental conditions. The presence of saliva has been described as another influencing factor of biofilm formation in Candida species. Its addition to the medium leads to a significant decrease of C. albicans or C. glabrata biofilm formation (52). Nonetheless, in the presence of saliva C. albicans exhibits more biofilm formation than C. glabrata, C. tropicalis, and C. parapsilosis, while the later exhibits higher adhesion capacity to abiotic surfaces. On buccal epithelial cells, C. glabrata exhibits the highest capacity of adhesion (54).
Fluid flow rate also affects biofilm formation, affecting EPS production, among other factors. Hawser et al. observed that under static conditions, EPS production was minimal when compared to the use of liquid flow. In fact, increasing the speed of flow, the ECM produced was significantly higher, totally wrapping the cells of C. albicans biofilm, whose metabolic activity did not change with such alterations. Nevertheless, a shaking speed of 60 rpm was found to inhibit biofilm formation (55). Moreover, Al-Fattani and Douglas showed that C. albicans and C. tropicalis biofilms had more ECM produced when grown under conditions of continuous flow in a modified Robbins device than in static conditions (40). Furthermore, C. albicans biofilms under shear stress were found to become thinner but denser, when compared to static conditions, and once again metabolic activity did not suffer any modification (56).
Biofilm formation is regulated by several transcription factors, including those involved in adhesion, hyphal formation, and EPS production, as assembled in Figure 2 and Table 1. The study of the elaborated regulatory network that underlies biofilm formation is a promising field of research, as it is expected to lead to the discovery of the best targets to tackle biofilm formation by Candida species in the host.
Figure 2. Transcription regulatory network described for Candida albicans, Candida glabrata, Candida tropicalis, and Candida parapsilosis, highlighting the transcriptional factors involved in adhesion, extracellular polymeric substances (EPS), filamentation, and biofilm. Green boxes indicate activators and red boxes indicate repressors. Participation of each transcription factor in these processes is indicated by the colored arrows: brown arrows correspond to adhesion, dark blue arrows correspond to EPS, light blue arrows correspond to filamentation, and yellow arrows correspond to biofilm formation.
Nobile et al. have identified important transcription factors essential for biofilm formation using a C. albicans library of 165 transcription factor deletion mutants that were tested for biofilm growth in vitro and in vivo on rat denture and catheter models (57). The biofilm defective deletion mutants identified were efg1Δ/Δ, bcr1Δ/Δ, tec1Δ/Δ, ndt80Δ/Δ, rob1Δ/Δ, and brg1Δ/Δ. Resorting to Chromatin ImmunoPrecipitation-on-chip, it was possible to unravel the promoter regions bound by each transcription factor during biofilm formation. The six transcription factors were found to regulate a high degree of overlapping target genes as well as to control the expression of each other (57).
In C. albicans, the main regulators of biofilm development are also involved in filamentous growth, given the typical hyphal differentiation that takes place during this process. That is the case of Efg1 and Cph1 that positively control the expression of genes required for hyphal growth, such as ECE1, HYR1, HWP1, and ALS3 (58–62). These transcription factors also control the expression of cell wall-related genes due to the usual morphological alterations involved in the transition from yeast to hyphae (63, 64). The Δefg1 and Δefg1Δcph1 deletion mutants have been shown to be incapable of filamentation or biofilm development, being only able to form a sparse monolayer of adherent elongated cells (65). C. albicans Efh1 was found to be a homolog of Efg1, regulating, together with Efg1, a set of genes involved in filamentation (66). In C. parapsilosis, Efg1 has been shown to be a morphological switch regulator (67), and to have a role in biofilm formation since its absence leads to reduced biofilm (68). Surprisingly, in C. glabrata, which is unable to form hyphae, a homolog of Efg1, encoded by ORF CAGL0L01771g, has been found but remains uncharacterized (69). In turn, Cph1 is the terminal transcription factor of the MAP kinase pathway in C. albicans, mediating a feedback regulation of this pathway (62). This protein belongs to the STE-like transcription factor family being present in C. albicans, C. tropicalis, and C. parapsilosis. In C. glabrata, the Cph1 ortholog is the Ste12 transcription factor involved in the regulation of filamentation induced by nitrogen starvation (69, 70).
Also involved in filamentous growth regulation in C. albicans is the Ndt80 transcription factor. This regulator has also been associated with the control of cell separation, azole resistance, virulence and biofilm formation (57, 71, 72). Likewise, Brg1 has a role in the transcriptional control of hyphal growth-related genes in C. albicans, since the absence of both copies of the BRG1 gene leads to impaired hyphal development. Meanwhile, its overexpression was found to lead to the increased expression of hyphae-specific genes, including ALS3, HWP1, and ECE1. C. albicans Brg1 is, in turn, repressed by the Nrg1 C2H2 zinc finger transcription factor, involved in the control of cell morphology and pathogenicity, by repressing filamentous growth (73, 74). The C. tropicalis Nrg1 homolog was similarly found to have a role in repressing filamentation (75), while other predicted homologs in C. parapsilosis, C. tropicalis and C. glabrata remain uncharacterized (69). Besides Nrg1, C. albicans and C. tropicalis count with Cup9 and Slf1 regulators to suppress filamentous growth (75). In turn, Ume6 transcription factor has also been found in C. parapsilosis, playing an important role in biofilm development (68), and in C. albicans and C. tropicalis, as a regulator of filamentous growth (75). Another negative regulator of filamentous growth and biofilm formation in C. albicans is Rfg1 (74, 76, 77). Together with Nrg1, Rfg1 down-regulates the expression of ALS3, ECE1, and HWP1 hyphae-specific genes (78, 79).
Bcr1, a C2H2 zinc finger transcription factor, is also an important regulator of biofilm formation, but not of hyphal formation. Bcr1 regulates the expression of cell surface proteins encoded by the HYR1, ECE1, RBT5, ECM331, HWP1, ALS3, ALS1, and ALS9 genes (80), controlling the adhesion process in C. albicans, during the early phase of biofilm formation. Moreover, the absence of Bcr1 in an in vivo rat model of catheter-based infection was found to abrogate biofilm formation after 48 h of infection. Interestingly, the overexpression of ALS3 in a bcr1/bcr1 background completely restored the biofilm formation phenotype (33). In C. parapsilosis, a homolog of Bcr1 was identified as one of the most important transcription factors regulating biofilm formation (68, 69, 81, 82). Bcr1 is itself positively regulated by the transcription factor Tec1, in C. albicans (80). Tec1 positively regulates morphogenesis and is essential for hyphae formation upon serum induction, macrophage evasion and expression of aspartyl proteinase genes (65, 83). In turn, Efg1 and Cph2 are known to regulate Tec1 (84, 85). C. parapsilosis has been found to have a homolog of Tec1 (69). Tec1 of C. tropicalis is an important transcription regulator promoting filamentation (75).
Regulation of biofilm matrix biogenesis has also been studied, although not so extensively. C. albicans Csr1 regulator is involved in the control of adherence (86) and development of the ECM of C. albicans biofilms (87). More specifically, Csr1 negatively controls the concentration of β-1,3-glucan present in the matrix by activating CSH1 and IFD6, and inhibiting the expression of GCA1, GCA2, and ADH5. Csr1 has also been found to participate in the control of filamentous growth (87). On the other hand, a transcription factor that positively controls glycolytic genes, Tye7, was found to be essential for biofilm cohesiveness in C. albicans (88).
The Rca1/Cst6 in C. albicans is a regulator of hyphal formation by positively controlling the expression of hyphal genes like GWP1, ECE1, HGC1, and ALS3 and the transcription factor Efg1 (89). In C. glabrata, Cst6 is another important transcription regulator of biofilm formation. This protein is a bZIP transcription factor of the ATF/CREB family that negatively controls the expression of the EPA6 gene, which encodes an important adhesin found in C. glabrata biofilms (90).
In C. albicans, an Ace2 transcriptional regulator was also found conserving the C2H2 zinc finger domain (69). The absence of Ace2 leads to hyperfilamentation and hypervirulence in C. albicans (91, 92), although it has been shown to be required for biofilm formation in normoxia conditions (93) and filamentous growth under hypoxic conditions (94). The C. parapsilosis and C. glabrata Ace2 homologs also play important roles in biofilm formation (68, 95).
Although having specific transcription factors regulating biofilm formation, C. glabrata has a number of related genes whose expression is controlled by subtelomeric silencing. HYR1, EPA1, EPA2, EPA3, EPA4, EPA5, EPA6, and EPA7 genes of C. glabrata have been found to be repressed by this process, given their loci proximity to a telomere. This regulation is mediated by the Sir complex (Sir2–Sir4), Rap1, Rif1, yKu70, and yKu80 (37, 96), as well as the Swi/Snf complex (90). Both Swi/Snf and Sir complexes appear to underlie the regulation of biofilm formation which is specific for C. glabrata, when compared with other Candida species.
Given the multifactorial nature of biofilm formation, its regulatory network is highly complex, involving the control of adhesion, hyphal formation, ECM production, and other processes (Figure 2). Finding a general regulator to tackle the regulatory control of biofilm in all Candida species is thus a very challenging goal.
Biofilm formation has been tightly linked to the development of resistance to antifungal drugs (Table 1). For example, resistance acquisition during biofilm formation was observed in a study where 48 h biofilms of C. albicans were found to exhibit five- to eightfold higher resistance to all antifungals when compared to planktonic cells (97). Increased resistance exhibited by C. parapsilosis biofilms to fluconazole, voriconazole, ravuconazole, amphotericin B, nystatin, chlorhexidine, and terbinafine have also been registered (98).
This enhancement of resistance when Candida species grow as biofilms might be explained by several factors. One of them is the increased metabolic activity occurring in the early development of biofilm. Chandra et al. have shown that although MICs of amphotericin B, fluconazole, nystatin, and chlorhexidine were 0.5, 1, 8, and 16 µg/mL, respectively, in the early stage of C. albicans biofilm formation on polymethylmethacrylate strips, after 72 h of biofilm formation, MICs of amphotericin B, fluconazole, nystatin, and chlorhexidine had changed to 8, 128, 32, and 256 µg/mL, respectively (19). Interestingly, however, the defective biofilm formation mutants, Δefg1 and Δefg1Δcph1, which only exhibit an adherent monolayer of elongated cells, show the same resistance toward fluconazole and amphotericin B as the wild-type. These results suggest that resistance to antifungal drugs is related to adherence, regardless of normal biofilm formation (65).
Another issue to consider is the possible role of the ECM in antifungal drug resistance. Some authors advocate that EPS contributes as a barrier to prevent the diffusion of drugs, showing that Candida cells resist 20% better to amphotericin B with EPS, when compared to the same cells upon EPS removal (18). Indeed, the extracellular β-1,3-glucan matrix has been proven to bind to amphotericin B, while its absence from the matrix, increases C. albicans susceptibility to fluconazole and amphotericin B (99). Other evidences were given in a study where radiolabeled drug (3H-fluconazole) is only sequestrated in the presence of matrix mannan and β-1,6-glucan, demonstrating the importance of these molecules in antifungal resistance (43).
Alterations in gene expression during C. albicans biofilm formation include the upregulation of CDR and MDR genes encoding azole resistance transporters (38). This upregulation seems to be important for the development of antifungal resistance in the early phase of the biofilm formation, while in the maturation process, changes in sterol composition appear to be more relevant (18). Nevertheless, it has been shown that a set of isogenic C. albicans strains carrying single or double deletions of genes encoding efflux pumps (Δcdr1, Δcdr2, Δmdr1, Δcdr1/cdr2, and Δmdr1/cdr1) could form biofilm like the parental strains, still displaying antifungal resistance. This gives evidence that antifungal resistance in biofilm does not depend on a single mechanism (38). Interestingly, overexpression of CDR1, CDR2 and TPO1_2 genes has also been observed in C. glabrata biofilms (100, 101). Tpo1_2 is a major facilitator superfamily transporter known to have a role in antifungal resistance (102) and to be necessary for the normal expression of ALS1, EAP1, and EPA1 genes involved in adhesion (101). In fact, C. glabrata biofilms have shown to be significantly more resistant than planktonic cells (100, 103).
Showing that some specific mechanism of biofilm formation might be behind the resistance to antifungal drugs, ALS3 and HWP1 were found to be induced upon exposure to caspofungin in C. albicans biofilms but not in planktonic growing cells (104). Additionally, the deletion of MNN9, MNN11, VAN1, MNN4-4, VRG4, PMR1, BIG1, KRE5, and FKS1 genes was found to lead to higher susceptibility to fluconazole in biofilm cells, but not in planktonic cells (43). Interestingly, exposure to 80 µg/mL of fluconazole was found to lead to almost no changes in gene expression in SC5314 C. albicans biofilms, while exposure to caspofungin or amphotericin B results in massive gene expression changes, suggesting that biofilm cells were innately protected against azole drugs (104).
Similar to what has been described for other microorganisms, persister cells are also found within biofilms formed by C. albicans. Persister cells are dormant, non-dividing cells that have high tolerance to antimicrobial drugs. This tolerance is believed to be possible thanks to the dormancy of the cell, which enables the binding of antimicrobial drugs to their specific target, while making it impossible to the drug to inhibit the function of the target molecule. This tolerance, however comes at the price of non-proliferation (105). In C. albicans, biofilm persister cells display resistance to fluconazole, while the same cells grown in non-biofilm planktonic culture are sensitive to fluconazole. Interestingly, these persister cells cultivated in biofilm exhibit increased expression of the CDR genes (38). This phenomenon was also observed for C. albicans cells in biofilm exposed to amphotericin B and chlorhexidine, but independently of the expression of drug efflux pumps (106).
Candida species biofilms have been usually studied as single species biofilm, although in vivo a very different reality is observed. Generally, biofilms are composed by multiple species that interact as a community having synergistic and/or antagonistic relationships. The synergistic interactions involve metabolic cooperation while antagonistic interactions include competition for nutrients and the generation of inhibitory compounds (107).
The formation of mixed biofilms of Candida species has been described with different combinations between Candida species or with Candida and bacteria. C. albicans, C. glabrata, C. krusei, and C. tropicalis have been shown to form biofilm with each other (52, 108). Although increased biofilm formation was observed for most combinations of Candida species, for C. tropicalis this increase was only observed when in interaction with C. albicans (108).
Candida albicans biofilms have also been shown to be favored by combination with four species of bacteria: Streptococcus mutans, Streptococcus sanguinis, Actinomyces viscosus, and Actinomyces odontolyticus. Increased hyphae development, accompanied by the overexpression of HWP1, ALS3, and EPA1, was observed in mixed-species biofilm, both on acrylic surfaces or in reconstituted human oral epithelia (RHOE). Moreover, tissue damage and invasiveness is higher when all these five species are infecting and forming biofilm on RHOE. When compared to single Candida or single bacteria biofilms, mixed-species biofilm are significantly more invasive (109).
Multispecies biofilm formed upon denture stomatitis that frequently involves C. albicans, S. mutans, S. sanguinis, and Actinomyces naeslundii has also been investigated. Evidence of the increased pathogenicity of C. albicans in the presence of these bacteria was obtained, suggesting that in these conditions the use of antibacterial agents could have an effect in decreasing fungal proliferation (110). Interestingly, S. mutants and C. albicans have been shown to have a synergetic relationship since their coexistence leads to increased biofilm formation, when compared to single biofilms, despite the negative effect S. mutants has on hyphae formation. This dual-species biofilm displays two layers: S. mutans cells attached to the surface followed by C. albicans cells as a second layer (52). Interestingly, S. mutans was also reported to increase biofilm formation of C. glabrata (52). Multispecies biofilm formation on infected mice has also been investigated, considering C. albicans in coexistence with epithelial cells, neutrophils and commensal oral bacteria, such as Enterococcus/Lactobacillus sp. and Staphylococcus sp. (9).
Staphylococcus aureus interaction with C. albicans has been described in more detailed. Both species are able to cooperate in order to form a polymicrobial biofilm, even in the presence of serum which does not allow the formation of S. aureus single species biofilm. Visualization of these dual-species biofilm showed a first layer of C. albicans biofilm attached to the surface covered by a S. aureus monolayer that is included in the ECM formed by Candida cells. This ECM has proven to protect S. aureus from killing by vancomycin (111). This positive interaction has shown to be also dependent on hyphae formation by C. albicans (112). Staphylococcus epidermis has also been shown to cooperate with C. albicans, forming a multispecies biofilm that allows the protection of S. epidermidis against vancomycin and of C. albicans against fluconazole (40, 113) and amphotericin B (40).
When a synergistic relationship takes place, quorum sensing is an essential process that allows communication between species. Quorum sensing has been described mostly in bacteria, although several studies have already reported the use of quorum sensing between bacteria and Candida species (9, 114). This process comprises the production and release of a signal molecule (autoinducer), which, according to the cell density, will increase in concentration and orchestrate a collective and coordinated expression of given genes, in all the species involved for the development of biofilm (107). One example of quorum sensing has been described for the interaction between C. albicans and Streptococcus gordonii. These two species have been shown to coaggregate thanks to two cell wall anchored proteins of S. gordonii, SspA and SspB (114, 115), the later interacting directly with exposed C. albicans Als3 (116). The positive interaction between these two species is based on the production and release of a chemical molecule denominated autoinducer 2 by S. gordonii (117). This interaction has several advantages to C. albicans, such as the enhancement of hyphae formation and resistance to farnesol (114).
Farnesol is also a quorum-sensing molecule but produced as a secondary product of sterol biosynthesis and secreted by C. albicans (118). When accumulated, it prevents the formation of filamentous growth and biofilm (119–122). Generally, farnesol is mostly secreted in the later stages of biofilm formation by C. albicans (122). Among pathogenic Candida species, only C. albicans and C. dubliniensis are known to secrete farnesol (123). Farnesol is considered to be an autoinducer molecule that influences the expression of genes involved in antifungal resistance, cell wall maintenance, phagocytic response, surface hydrophobicity, iron metabolism and heat shock (120). Given its effect and synergistic action with antifungal therapy, it has been considered as a potential therapeutic agent (124). In C. parapsilosis, farnesol has also been described as having an inhibitory effect on growth and biofilm formation (125, 126). The presence of exogenous farnesol has been shown to lead to increased expression of oxidoreductases and a change in the expression of genes related to sterol metabolism (125). Moreover, reference strains of C. parapsilosis, C. tropicalis, C. dubliniensis, and C. albicans were found to have reduced biofilm production upon exposure to farnesol. However, C. glabrata and Candida krusei biofilm development was found to remain unaltered upon exposure of this quorum-sensing molecule.
Along with farnesol, a few other molecules produced by C. albicans have been proposed to work as quorum-sensing molecules, including morphogenic autoregulatory substance, phenylethyl alcohol and tryptophol (127), tyrosol, and farnesoic acid. Although through different pathways, farnesoic acid, just like farnesol, is involved in regulating the yeast-to-hyphae transition (119, 127). Depending on the strain, C. albicans might produce farnesol or farnesoic acid, both having an inhibitory effect of filamentous growth (127). Tyrosol, on the other hand, has an opposite effect on yeast-to-hyphae transition, increasing cell density and promoting the formation of germ tubes and the transition from yeast to hyphae (128). Tyrosol is produced by planktonic and biofilm cells, having a stimulating effect in hyphae formation in the early stage of biofilm development (122).
Interaction of C. albicans with Aspergillus nidulans has been shown to be competitive thanks to the production of farnesol. In fact, the presence of exogenous farnesol or the co-culture of A. nidulans with C. albicans activates apoptosis in A. nidulans cells. This activation takes place in a mitochondria dependent way, through the FadA G protein complex signal transduction pathway (129).
Another antagonistic relationship is the one described between C. albicans and Pseudomonas aeruginosa. In this specific case, P. aeruginosa is known to attach to C. albicans filaments, forming biofilm over the hyphae instead of the surface. The formation of biofilm ultimately leads to the death of C. albicans filamentous cells, although yeast cells remain viable (130). In fact, the presence of secreted factors by P. aeruginosa causes downregulation of genes involved in adhesion and biofilm formation, and increases the expression of genes encoding drug exporters, such as CDR1 and SNQ2, and the YWP1 gene encoding a protein involved in biofilm dispersal, in C. albicans biofilms (131). This negative effect over C. albicans is related to a quorum sensing molecule produced and secreted by P. aeruginosa, the 3-oxo-C12 homoserine lactone. Other compounds with a 12-carbon backbone, like dodecanol, have a similar effect on C. albicans filamentous growth (132). On the other hand, farnesol produced by C. albicans leads to a decreased quinolone signal from Pseudomonas that regulates the production of extracellular proteases, hydrogen cyanide and redox-active phenazines (133). According to Holcombe et al., the factors secreted by P. aeruginosa seem to have a specific effect in the maturation phase of biofilm formation by Candida species (131). C. glabrata, C. tropicalis, C. parapsilosis, and C. dubliniensis biofilm formation is also inhibited upon interaction with P. aeruginosa (134), which has a growth inhibitory effect on these Candida species (135, 136). Interestingly, P. aeruginosa proliferation is also inhibited in the presence of C. albicans, C. glabrata, and C. krusei (134).
Although no definitive solution for Candida biofilms has been found yet, there are several promising strategies being implemented nowadays, as well as intense research being developed in the search for novel solutions that alone or together with others might become the answer to this problem.
The current therapies with partial success to fight this challenge are based on two different approaches. The first, called “lock” therapy is directed to eradicate biofilm formation in catheters prior to their contact with the patient. The strategy consists in diffusing a high concentration of an antimicrobial drug into the catheter lumen, letting the agent act during a period of several hours or days. This technique avoids systemic toxicity since the antimicrobial drug only acts in the catheter (137). Examples of success using the “lock” therapy are the tests performed on silicone catheters infected with different C. albicans and C. glabrata isolates, where micafungin (5 and 15 mg/L), caspofungin (5 and 25 mg/L), and posaconazole (10 mg/L) were used as antimicrobial agents. All antifungals used with this technique help decrease Candida biofilms, being micafungin the most effective (138). Nevertheless, when using amphotericin B lipid formulation (1,000 mg/L), known to have activity against Candida biofilms (98), in a “lock” therapy approach, an effective eradication of Candida biofilms was not observed (139). Moreover, an interesting antimicrobial agent tested in “lock” therapy is ethanol. Combined “lock” therapy using 0.3 mL of 70% ethanol and 5 mg/L of micafungin was effective eliminating a catheter-related candidemia in a male infant (140). Ethanol is an advantageous antimicrobial agent given its low cost and effectiveness against Candida species.
The other approach is catheter coating. Modification of the coating of the catheter with minocycline-rifampin, chlorhexidine, or silver sulfadiazine decreases the number of bloodstream infections caused by central venous catheters (141). Coating with nanomaterials or nanoparticles has been studied as a possible improvement in the effect of reducing the occurrence of these infections. Although this technology has been developed with the main focus of eliminating bacteria, the use of silane system coatings on titanium and zirconia specimens has been described in a process of implant modification for the elimination of Candida albicans biofilms (142).
Given the absence of a real solution, new fields have been explored in order to find a different path to eliminate Candida biofilms. Research has been developed to find new natural products or synthetic peptides that might have an antifungal action. The study of different compounds has revealed interesting candidates with antifungal activity. Such is the case of phenylpropanoids and terpenoids of plant origin (143, 144), and phenazines produced by P. aeruginosa (145), which inhibit yeast-to-hyphal transition and biofilm formation in C. albicans. Besides these compounds, several high-throughput screenings have been performed trying to identify the best candidates to inhibit Candida biofilms. Siles et al. have screened a library of 1,200 off-patent drugs approved by the Food and Drug Administration, finding 38 active compounds against C. albicans biofilms. Another high-throughput screening has identified the SM21 compound as the best inhibitor of yeast-to-hypha transition, from a library of 50,240 small molecules, not having any toxic effect when tested in different human cell lines (146). Moreover, some synthetic peptides also display significant antifungal activity, as is the case of KSL-W. This peptide significantly affects the growth, yeast-to-hypha transition and biofilm formation of C. albicans (147). Karlsson et al. have used an antifungal β-peptide in coating nanotechnology. This approach was based on the design of polymer films that enable the localized release of the β-peptide from the surface. When testing film surfaces coated with this polymer, a significant reduction of cell viability, metabolic activity and hyphal elongation was observed (148).
Besides coating the surfaces with given molecules, another strategy is based on the modification of polymers incorporated in medical devices. Synthesis of water insoluble and organo-soluble polyethylenimine derivates with subsequent quaternization of N-methyl polyethylenimine with alkyl bromides was evaluated regarding the antifungal activity obtained. The resulting cationic polymers are capable of inhibiting C. albicans, C. tropicalis, and C. dubliniensis growth very effectively. The mode of action of these polymers is believed to be related to the disruption of membrane integrity (149). Furthermore, chitosan hydrogel is another available polymer from natural origin that may be used to modify the surface of medical devices. Chitosan is very effective in impairing biofilm formation of C. albicans, C. parapsilosis, C. glabrata, C. tropicalis, C. krusei, and C. guilliermondii, having been tested positively in a in vivo catheter mouse model against C. parapsilosis biofilm formation (150).
Although most antifungal agents fail to eliminate Candida biofilms, there is still hope thanks to some relatively new drug formulations. That is the case of lipid formulations of polyene antifungals, such as liposomal amphotericin B and amphotericin B lipid complex. When used against C. albicans biofilms formed in a bioprosthetic model, the results show MICs like those obtained with planktonic cells. Also, the echinocandins, caspofungin and micafungin, have effective action against C. albicans and C. parapsilosis biofilms in the same model (98). Interestingly, when biofilm precursor planktonic cells were exposed to subinhibitory levels of voriconazole, nystatin or chlorhexidine, and subsequently left to form biofilm, the same antifungal resistance of biofilms was not observed. In fact, a significant decrease of the capacity of the cells to form biofilm was observed (98). Another interesting drug that has been discovered to have a role in acting against in vitro filamentous growth in C. albicans and against biofilms in C. albicans, C. glabrata, and C. parapsilosis is acetylsalicylic acid, usually known as aspirin. A decrease in biofilm formation was observed for aspirin concentrations between 0.43 and 1.73 mM (151).
An alternative approach to eradicate Candida biofilms consists in the photodynamic inactivation. This technique is based on the use of visible light and a nontoxic dye called photosensitizer that when activated leads to the production of reactive oxygen species which subsequently kill the targeted microbial cells due to the damage provoked on DNA, proteins, and/or cell membrane. Different photosensitizers have been tested against Candida biofilms demonstrating the great potential of this technique as an antimicrobial therapy. The photosensitizer toluidine blue (0.1 mg/mL) has been used successfully, reducing up to 60% the formation of C. albicans biofilms (152). Another promising photosensitizer is methylene blue, capable of preventing C. parapsilosis biofilm formation ex vivo on mouse tongues after being activated by red LED light (153). Interestingly, the combination of photodynamic inactivation strategy, using toluidine blue, together with chitosan, results in an enhancement of the effect of both approaches against Candida biofilms (154). The nontoxicity of the dyes and the low cost of the technique highlight the great potential of this alternative approach (155).
This review highlights the overwhelming complexity of the intracellular mechanisms leading to the formation of Candida biofilms, including those controlling adhesion, changes in cell morphology and EPS production, especially considering that these have been shown to be in many cases species, or even strain, specific. Additionally, external factors, including the surface where the biofilm forms, which may be a layer of epithelial cells or very diversified plastic polymers, the cocktail of nutrients and inhibitors that are present in the surrounding environment or the presence of other synergistic or antagonistic microorganisms, have a tremendous effect on the final characteristics of the formed biofilm.
All this variability poses concerning challenges from the clinical point of view, leading to the successful persistence of Candida infections associated with high mortality rates. All the tactics against Candida biofilms implemented so far are only able to address this problem partially. Therefore, treatment and prevention of Candida biofilms must suffer improvements so that an effective solution might be applied. As discussed, more recent strategies have the potential to be improved although continuous research for new therapies or for the best combination between the available ones are also possible approaches. Nonetheless, the specific knowledge on all the mechanisms behind biofilm formation and antifungal resistance in each Candida species will be crucial for the delineation of a more effective strategic plan in the fight against Candida infections.
Despite all gathered knowledge, there is still a lot to be scrutinized, including many aspects regarding the formation of biofilms by non-albicans Candida species, whose study is decades behind that of C. albicans. Table 1 and Figure 2 highlight a lot of the blanks that are still to be filled regarding the specific mechanisms used by C. glabrata, C. parapsilosis, and C. tropicalis, which are emerging as important human pathogens. Additionally, it will also be highly relevant to ascertain the differences between biofilms formed by commensal Candida populations and those related to increased pathogenesis and persistent infections.
MC gathered the bibliographic material. MC and MT wrote the manuscript. MT made the conceptual design of the manuscript and guided its production.
The authors declare that the research was conducted in the absence of any commercial or financial relationships that could be construed as a potential conflict of interest.
Research conducted by the authors within the scope of this article was supported by “Fundação para a Ciência e a Tecnologia” (FCT) [Contracts PTDC/BBB-BIO/4004/2014 and PhD grant to MC (PD/BD/116946/2016)]. Funding received by iBB-Institute for Bioengineering and Biosciences from FCT-Portuguese Foundation for Science and Technology (UID/BIO/04565/2013) and from Programa Operacional Regional de Lisboa 2020 (Project N. 007317) is acknowledged.
1. Lewis KIM. Riddle of biofilm resistance. Antimicrob Agents Chemother (2001) 45:999–1007. doi:10.1128/AAC.45.4.999-1007.2001
2. Donlan RM. Biofilms and device-associated infections. Emerg Infect Dis (2001) 7:277–81. doi:10.3201/eid0702.010226
3. Douglas LJ. Candida biofilms and their role in infection. Trends Microbiol (2003) 11:30–6. doi:10.1016/S0966-842X(02)00002-1
4. Potera C. Forging a link between biofilms and disease. Science (1999) 283:1837–9. doi:10.1126/science.283.5409.1837
5. O’Toole G, Kaplan HB, Kolter R. Biofilm formation as microbial development. Annu Rev Microbiol (2000) 54:49–79. doi:10.1146/annurev.micro.54.1.49
6. Enfert C, Janbon G. Biofilm formation in Candida glabrata: what have we learnt from functional genomics approaches? FEMS Yeast Res (2016) 16:1–13. doi:10.1093/femsyr/fov111
7. Sims CR, Ostrosky-zeichner L, Rex JH. Invasive candidiasis in immunocompromised hospitalized patients. Arch Med Res (2005) 36:660–71. doi:10.1016/j.arcmed.2005.05.015
8. Ganguly S, Mitchell AP. Mucosal biofilms of Candida albicans. Curr Opin Microbiol (2011) 14:380–5. doi:10.1016/j.mib.2011.06.001
9. Dongari-bagtzoglou A, Kashleva H, Dwivedi P, Diaz P, Vasilakos J. Characterization of mucosal Candida albicans biofilms. PLoS One (2009) 4:e7967. doi:10.1371/journal.pone.0007967
10. Geffers C, Gastmeier P. Nosocomial infections and multidrug-resistant organisms in Germany: epidemiological data from KISS (The Hospital Infection Surveillance System). Dtsch Arztebl Int (2011) 108:87–93. doi:10.3238/arztebl.2011.0087
11. Wenzel RP, Gennings C. Bloodstream infections due to Candida species in the intensive care unit: identifying especially high-risk patients to determine prevention strategies. Clin Infect Dis (2005) 41:389–93. doi:10.1086/430923
12. Elving GJ, Van Der Mei HC, Busscher HJ, Van Weissenbruch R, Albers FWJ. Comparison of the microbial composition of voice prosthesis biofilms from patients requiring frequent versus infrequent replacement. Ann Otol Rhinol Laryngol (2002) 111:200–3. doi:10.1177/000348940211100302
13. Kojic EM, Darouiche RO. Candida infections of medical devices. Clin Microbiol Rev (2004) 17:255–67. doi:10.1128/CMR.17.2.255-267.2004
14. Tscherner M, Schwarzmüller T, Kuchler K. Pathogenesis and antifungal drug resistance of the human fungal pathogen Candida glabrata. Pharmaceuticals (2011) 4:169–86. doi:10.3390/ph4010169
15. Rho J, Shin JH, Song JW, Park MR, Kee SJ, Jang SJ, et al. Molecular investigation of two consecutive nosocomial clusters of Candida tropicalis candiduria using pulsed-field gel electrophoresis. J Microbiol (2004) 42:80–6.
16. Bonassoli LA, Bertoli M, Svidzinski TIE. High frequency of Candida parapsilosis on the hands of healthy hosts. J Hosp Infect (2005) 59:159–62. doi:10.1016/j.jhin.2004.06.033
17. Yapar N. Epidemiology and risk factors for invasive candidiasis. Ther Clin Risk Manag (2014) 10:95–105. doi:10.2147/TCRM.S40160
18. Seneviratne CJ, Jin L, Samaranayake LP. Biofilm lifestyle of Candida: a mini review. Oral Dis (2008) 14:582–90. doi:10.1111/j.1601-0825.2007.01424.x
19. Chandra J, Kuhn DM, Mukherjee PK, Hoyer LL, McCormick T, Ghannoum MA. Biofilm formation by the fungal pathogen Candida albicans: development, architecture, and drug resistance. J Bacteriol (2001) 183:5385–94. doi:10.1128/JB.183.18.5385-5394.2001
20. Mitchell KF, Zarnowski R, Andes DR. The extracellular matrix of fungal biofilms. Adv Exp Med Biol (2016) 931:21–35. doi:10.1007/5584_2016_6
21. Ramage G, Vande Walle K, Wickes BL, Lopez-Ribot JL. Biofilm formation by Candida dubliniensis. J Clin Microbiol (2001) 39:3234–40. doi:10.1128/JCM.39.9.3234-3240.2001
22. Silva S, Henriques M, Martins A, Oliveira R, Williams D, Azeredo J. Biofilms of non-Candida albicans Candida species: quantification, structure and matrix composition. Med Mycol (2009) 47:681–9. doi:10.3109/13693780802549594
23. Bizerra FC, Nakamura CV, de Poersch C, Estivalet Svidzinski TI, Borsato Quesada RM, Goldenberg S, et al. Characteristics of biofilm formation by Candida tropicalis and antifungal resistance. FEMS Yeast Res (2008) 8:442–50. doi:10.1111/j.1567-1364.2007.00347.x
24. Lattif AA, Mukherjee PK, Chandra J, Swindell K, Lockhart SR, Diekema DJ, et al. Characterization of biofilms formed by Candida parapsilosis, C. metapsilosis, and C. orthopsilosis. Int J Med Microbiol (2010) 300:265–70. doi:10.1016/j.ijmm.2009.09.001
25. Nett J. The host’s reply to Candida biofilm. Pathogens (2016) 5:1–10. doi:10.3390/pathogens5010033
26. Mermel LA, Farr BM, Sherertz RJ, Raad II, O’Grady N, Harris JS, et al. Guidelines for the management of intravascular catheter-related infections. Clin Infect Dis (2001) 32:1249–72. doi:10.1086/320001
27. Chandra J, Mukherjee P. Candida biofilms: development, architecture, and resistance. Microbiol Spectr (2015) 3:157–76. doi:10.1128/microbiolspec.MB-0020-2015
28. Blankenship JR, Mitchell AP. How to build a biofilm: a fungal perspective. Curr Opin Microbiol (2006) 9:588–94. doi:10.1016/j.mib.2006.10.003
29. Andes D, Nett J, Oschel P, Albrecht R, Marchillo K, Pitula A. Development and characterization of an in vivo central venous catheter Candida albicans biofilm model. Infect Immun (2004) 72:6023–31. doi:10.1128/IAI.72.10.6023-6031.2004
30. Řičicová M, Kucharíková S, Tournu H, Hendrix J, Bujdáková H, Van Eldere J, et al. Candida albicans biofilm formation in a new in vivo rat model. Microbiology (2010) 156:909–19. doi:10.1099/mic.0.033530-0
31. Kucharikova S, Neirinck B, Sharma N, Vleugels J, Lagrou K, Van Dijck P, et al. In vivo Candida glabrata biofilm development on foreign bodies in a rat subcutaneous model. J Antimicrob Chemother (2014) 70:846–56. doi:10.1093/jac/dku447
32. Verstrepen KJ, Klis FM. Flocculation, adhesion and biofilm formation in yeasts. Mol Microbiol (2006) 60:5–15. doi:10.1111/j.1365-2958.2006.05072.x
33. Nobile CJ, Andes DR, Nett JE, Smith FJ, Yue F, Phan QT, et al. Critical role of Bcr1-dependent adhesins in C. albicans biofilm formation in vitro and in vivo. PLoS Pathog (2006) 2:e63. doi:10.1371/journal.ppat.0020063
34. de Groot PWJ, Bader O, de Boer AD, Weig M, Chauhan N. Adhesins in human fungal pathogens: glue with plenty of stick. Eukaryot Cell (2013) 12:470–81. doi:10.1128/EC.00364-12
35. Modrezewka B, Kurnatowski P. Adherence of Candida sp. to host tissues and cells as one of its pathogenicity features. Ann Parasitol (2015) 61:3–9.
36. Henriques M, Azeredo J, Oliveira R. Candida species adhesion to oral epithelium: factors involved and experimental methodology used. Crit Rev Microbiol (2006) 32:217–26. doi:10.1080/10408410601023524
37. De Las Peñas A, Pan SJ, Castaño I, Alder J, Cregg R, Cormack BP. Virulence-related surface glycoproteins in the yeast pathogen Candida glabrata are encoded in subtelomeric clusters and subject to RAP1- and SIR-dependent transcriptional silencing. Genes Dev (2003) 17:2245–58. doi:10.1101/gad.1121003.cans
38. Ramage G, Bachmann S, Patterson TF, Wickes BL, López-ribot JL. Investigation of multidrug efflux pumps in relation to fluconazole resistance in Candida albicans biofilms. J Antimicrob Chemother (2002) 49:973–80. doi:10.1093/jac/dkf049
39. Kuhn DM, Chandra J, Mukherjee PK, Ghannoum MA. Comparison of biofilms formed by Candida albicans and Candida parapsilosis on bioprosthetic surfaces. Infect Immun (2002) 70:878–88. doi:10.1128/IAI.70.2.878-888.2002
40. Al-Fattani MA, Douglas LJ. Biofilm matrix of Candida albicans and Candida tropicalis: chemical composition and role in drug resistance. J Med Microbiol (2006) 55:999–1008. doi:10.1099/jmm.0.46569-0
41. Flemming H, Wingender J. The biofilm matrix. Nat Rev Microbiol (2010) 8:623–33. doi:10.1038/nrmicro2415
42. Zarnowski R, Westler WM, Lacmbouh GA, Marita JM, Bothe JR, Bernhardt J, et al. Novel entries in a fungal biofilm matrix encyclopedia. MBio (2014) 5:e1333–1314. doi:10.1128/mBio.01333-14
43. Mitchell KF, Zarnowski R, Sanchez H, Edward JA, Reinicke EL, Nett JE, et al. Community participation in biofilm matrix assembly and function. Proc Natl Acad Sci U S A (2015) 112:4092–7. doi:10.1073/pnas.1421437112
44. Jin Y, Samaranayake LP, Samaranayake Y, Yip HK. Biofilm formation of Candida albicans is variably affected by saliva and dietary sugars. Arch Oral Biol (2004) 49:789–98. doi:10.1016/j.archoralbio.2004.04.011
45. Baillie GS, Douglas LJ. Role of dimorphism in the development of Candida albicans biofilms. J Med Microbiol (1999) 48:671–9. doi:10.1099/00222615-48-7-671
46. Nikawa H, Nishimura H, Yamamoto T, Hamada T, Samaranayake LP. The role of saliva and serum in Candida albicans biofilm formation on denture acrylic surfaces. Microb Ecol Health Dis (1996) 9:35–48. doi:10.3109/08910609609167727
47. Susewind S, Lang R, Hahnel S. Biofilm formation and Candida albicans morphology on the surface of denture base materials. Mycoses (2015) 58:719–27. doi:10.1111/myc.12420
48. Hawser SP, Douglas LJ. Biofilm formation by Candida species on the surface of catheter materials in vitro. Infect Immun (1994) 62:915–21.
49. Radford DR, Sweet SP, Challacombe SJ, Walter JD. Adherence of Candida albicans to denture-base materials with different surface finishes. J Dent (1998) 26:577–83. doi:10.1016/S0300-5712(97)00034-1
50. Chandra J, Patel JD, Li J, Zhou G, Mukherjee PK, McCormick TS, et al. Modification of surface properties of biomaterials influences the ability of Candida albicans to form biofilms. Appl Environ Microbiol (2005) 71:8795–801. doi:10.1128/AEM.71.12.8795-8801.2005
51. Estivill D, Arias A, Torres-Lana A, Carrillo-Muñoz AJ, Arévalo MP. Biofilm formation by five species of Candida on three clinical materials. J Microbiol Methods (2011) 86:238–42. doi:10.1016/j.mimet.2011.05.019
52. Pereira-Cenci T, Deng DM, Kraneveld EA, Manders EM, Del Bel Cury AA, Ten Cate JM, et al. The effect of Streptococcus mutans and Candida glabrata on Candida albicans biofilms formed on different surfaces. Arch Oral Biol (2008) 53:755–64. doi:10.1016/j.archoralbio.2008.02.015
53. Ng TS, Desa MNM, Sandai D, Chong PP, Than LTL. Growth, biofilm formation, antifungal susceptibility and oxidative stress resistance of Candida glabrata are affected by different glucose concentrations. Infect Genet Evol (2016) 40:331–8. doi:10.1016/j.meegid.2015.09.004
54. Queiroz PA, Godoy JS, Mendonça Pde S, Pedroso RB, Svidzinski TI, Negri M. Adhesion and biofilm formation in artificial saliva and susceptibility of yeasts isolated from chronic kidney patients undergoing haemodialysis. J Med Microbiol (2015) 64:960–6. doi:10.1099/jmm.0.000122
55. Hawser SP, Baillie GS, Douglas LJ. Production of extracellular matrix by Candida albicans biofilms. J Med Microbiol (1998) 47:253–6. doi:10.1099/00222615-47-3-253
56. Mukherjee PK, Chand DV, Chandra J, Anderson JM, Ghannoum MA. Shear stress modulates the thickness and architecture of Candida albicans biofilms in a phase-dependent manner. Mycoses (2009) 52:440–6. doi:10.1111/j.1439-0507.2008.01632.x
57. Nobile CJ, Fox EP, Nett JE, Sorrells TR, Mitrovich QM, Hernday AD, et al. A recently evolved transcriptional network controls biofilm development in Candida albicans. Cell (2013) 148:126–38. doi:10.1016/j.cell.2011.10.048
58. Birse CE, Irwin MY, Fonzi WA, Sypherdt PS. Cloning and characterization of ECE1, a gene expressed in association with cell elongation of the dimorphic pathogen Candida albicans. Infect Immun (1993) 61:3648–55.
59. Bailey DA, Feldmann PJF, Bovey M, Gow NAR, Brown AJP. The Candida albicans HYR1 gene, which is activated in response to hyphal development, belongs to a gene family encoding yeast cell wall proteins. J Bacteriol (1996) 178:5353–60. doi:10.1128/jb.178.18.5353-5360.1996
60. Staab JF, Ferrer CA, Sundstrom P. Developmental expression of a tandemly repeated, proline- and glutamine-rich amino acid motif on hyphal surfaces of Candida albicans. J Biol Chem (1996) 271:6298–305. doi:10.1074/jbc.271.11.6298
61. Hoyer LL, Payne TL, Bell M, Myers AM, Scherer S. Candida albicans ALS3 and insights into the nature of the ALS gene family. Curr Genet (1998) 33:451–9. doi:10.1007/s002940050359
62. Maiti P, Ghorai P, Ghosh S, Kamthan M, Tyagi RK, Datta A. Mapping of functional domains and characterization of the transcription factor Cph1 that mediate morphogenesis in Candida albicans. Fungal Genet Biol (2015) 83:45–57. doi:10.1016/j.fgb.2015.08.004
63. Chaffin WL, López-Ribot JL, Casanova M, Gozalbo D, Martinez JP. Cell wall and secreted proteins of Candida albicans: identification, function, and expression. Microbiol Immunol (1998) 62:130–80.
64. Sohn K, Urban C, Brunner H, Rupp S. EFG1 is a major regulator of cell wall dynamics in Candida albicans as revealed by DNA microarrays. Mol Microbiol (2003) 47:89–102. doi:10.1046/j.1365-2958.2003.03300.x
65. Ramage G, VandeWalle K, López-Ribot JL, Wickes BL. The filamentation pathway controlled by the Efg1 regulator protein is required for normal biofilm formation and development in Candida albicans. FEMS Microbiol Lett (2002) 214:95–100. doi:10.1111/j.1574-6968.2002.tb11330.x
66. Doedt T, Krishnamurthy S, Bockmühl DP, Tebarth B, Stempel C, Russell CL, et al. APSES proteins regulate morphogenesis and metabolism in Candida albicans. Mol Biol Cell (2004) 15:3167–80. doi:10.1091/mbc.E03-11-0782
67. Connolly LA, Riccombeni A, Grózer Z, Holland LM, Lynch DB, Andes DR, et al. The APSES transcription factor Efg1 is a global regulator that controls morphogenesis and biofilm formation in Candida parapsilosis. Mol Microbiol (2013) 90:36–53. doi:10.1111/mmi.12345
68. Holland LM, Schröder MS, Turner SA, Taff H, Andes D, Grózer Z, et al. Comparative phenotypic analysis of the major fungal pathogens Candida parapsilosis and Candida albicans. PLoS Pathog (2014) 10:e1004365. doi:10.1371/journal.ppat.1004365
69. Pais P, Costa C, Cavalheiro M, Romão D, Teixeira MC. Transcriptional control of drug resistance, virulence and immune system evasion in pathogenic fungi: a cross-species comparison. Front Cell Infect Microbiol (2016) 6:1–26. doi:10.3389/fcimb.2016.00131
70. Calcagno AM, Bignell E, Warn P, Jones MD, Denning DW, Mühlschlegel FA, et al. Candida glabrata STE12 is required for wild-type levels of virulence and nitrogen starvation induced filamentation. Mol Microbiol (2003) 50:1309–18. doi:10.1046/j.1365-2958.2003.03755.x
71. Sellam A, Tebbji F, Nantel A. Role of Ndt80p in sterol metabolism regulation and azole resistance in Candida albicans. Eukaryot Cell (2009) 8:1174–83. doi:10.1128/EC.00074-09
72. Sellam A, Askew C, Epp E, Tebbji F, Mullick A, Whiteway M, et al. Role of transcription factor CaNdt80p in cell separation, hyphal growth, and virulence in Candida albicans. Eukaryot Cell (2010) 9:634–44. doi:10.1128/EC.00325-09
73. Cleary IA, Lazzell AL, Monteagudo C, Thomas DP, Stephen P. BRG1 and NRG1 form a novel feedback circuit regulating C. albicans hyphal formation and virulence. Mol Microbiol (2012) 85:557–73. doi:10.1111/j.1365-2958.2012.08127.x
74. Braun BR, Kadosh D, Johnson AD. NRG1, a repressor of filamentous growth in C. albicans, is down-regulated during filament induction. EMBO J (2001) 20:4753–61. doi:10.1093/emboj/20.17.4753
75. Zhang Q, Tao L, Guan G, Yue H, Liang W, Cao C, et al. Regulation of filamentation in the human fungal pathogen Candida tropicalis. Mol Microbiol (2016) 99:528–45. doi:10.1111/mmi.13247
76. Murad AM, d’Enfert C, Gaillardin C, Tournu H, Tekaia F, Talibi D, et al. Transcript profiling in Candida albicans reveals new cellular functions for the transcriptional repressors CaTup1, CaMig1 and CaNrg1. Mol Microbiol (2001) 42:981–93. doi:10.1046/j.1365-2958.2001.02713.x
77. Khalaf RA, Zitomer RS. The DNA binding protein Rfg1 is a repressor of filamentation in Candida albicans. Genetics (2001) 157:1503–12.
78. Kadosh D, Johnson AD. Induction of the Candida albicans filamentous growth program by relief of transcriptional repression: a genome-wide analysis. Mol Biol Cell (2005) 16:2903–12. doi:10.1091/mbc.E05-01-0073
79. Kadosh D, Johnson AD. Rfg1, a protein related to the to the Saccharomyces cerevisiae hypoxic regulator Rox1, controls filamentous growth and virulence in Candida albicans. Mol Cell Biol (2001) 21:2496–505. doi:10.1128/MCB.21.7.2496-2505.2001
80. Nobile CJ, Mitchell AP. Regulation of cell-surface genes and biofilm formation by the C. albicans transcription factor Bcr1p. Curr Biol (2005) 15:1150–5. doi:10.1016/j.cub.2005.05.047
81. Ding C, Butler G. Development of a gene knockout system in Candida parapsilosis reveals a conserved role for BCR1 in biofilm formation. Eukaryot Cell (2007) 6:1310–9. doi:10.1128/EC.00136-07
82. Ding C, Vidanes GM, Maguire SL, Guida A, Synnott JM, Andes DR, et al. Conserved and divergent roles of Bcr1 and CFEM proteins in Candida parapsilosis and Candida albicans. PLoS One (2011) 6:e28151. doi:10.1371/journal.pone.0028151
83. Schweizer A, Rupp S, Taylor BN, Rollinghoff M, Schroppel K. The TEA/ATTS transcription factor CaTec1p regulates hyphal development and virulence in Candida albicans. Mol Microbiol (2000) 38:2–12. doi:10.1046/j.1365-2958.2000.02132.x
84. Lane S, Birse C, Zhou S, Matson R, Liu H. DNA array studies demonstrate convergent regulation of virulence factors by Cph1, Cph2, and Efg1 in Candida albicans. J Biol Chem (2001) 276:48988–96. doi:10.1074/jbc.M104484200
85. Lane S, Zhou S, Pan T, Dai Q, Liu H. The basic helix-loop-helix transcription factor Cph2 regulates hyphal development in Candida albicans partly via Tec1. Mol Cell Biol (2001) 21:6418–28. doi:10.1128/MCB.21.19.6418-6428.2001
86. Finkel JS, Xu W, Huang D, Hill EM, Desai JV, Woolford CA, et al. Portrait of Candida albicans adherence regulators. PLoS Pathog (2012) 8:e1002525. doi:10.1371/journal.ppat.1002525
87. Nobile CJ, Nett JE, Hernday AD, Homann OR, Deneault JS, Nantel A, et al. Biofilm matrix regulation by Candida albicans Zap1. PLoS Biol (2009) 7:e1000133. doi:10.1371/journal.pbio.1000133
88. Bonhomme J, Chauvel M, Goyard S, Roux P, Rossignol T, d’Enfert C. Contribution of the glycolytic flux and hypoxia adaptation to efficient biofilm formation by Candida albicans. Mol Microbiol (2011) 80:995–1013. doi:10.1111/j.1365-2958.2011.07626.x
89. Vandeputte P, Pradervand S, Ischer F, Coste AT, Ferrari S, Harshman K, et al. Identification and functional characterization of Rca1, a transcription factor involved in both antifungal susceptibility and host response in Candida albicans. Eukaryot Cell (2012) 11:916–31. doi:10.1128/EC.00134-12
90. Riera M, Mogensen E, d’Enfert C, Janbon G. New regulators of biofilm development in Candida glabrata. Res Microbiol (2012) 163:297–307. doi:10.1016/j.resmic.2012.02.005
91. Kelly MT, MacCallum DM, Clancy SD, Odds FC, Brown AJ, Butler G. The Candida albicans CaACE2 gene affects morphogenesis, adherence and virulence. Mol Microbiol (2004) 53:969–83. doi:10.1111/j.1365-2958.2004.04185.x
92. MacCallum DM, Findon H, Kenny CC, Butler G, Haynes K, Odds FC. Different consequences of ACE2 and SWI5 gene disruptions for virulence of pathogenic and nonpathogenic yeasts. Infect Immun (2006) 74:5244–8. doi:10.1128/IAI.00817-06
93. Stichternoth C, Ernst JF. Hypoxic adaptation by Efg1 regulates biofilm formation by Candida albicans. Appl Environ Microbiol (2009) 75:3663–72. doi:10.1128/AEM.00098-09
94. Mulhern SM, Logue ME, Butler G. Candida albicans transcription factor Ace2 regulates metabolism and is required for filamentation in hypoxic conditions. Eukaryot Cell (2006) 5:2001–13. doi:10.1128/EC.00155-06
95. Stead DA, Walker J, Holcombe L, Gibbs SR, Yin Z, Selway L, et al. Impact of the transcriptional regulator, Ace2, on the Candida glabrata secretome. Proteomics (2009) 10:212–23. doi:10.1002/pmic.200800706
96. Gallegos-García V, Pan SJ, Juárez-Cepeda J, Ramírez-Zavaleta CY, Martin-del-Campo MB, Martínez-Jiménez V, et al. A novel downstream regulatory element cooperates with the silencing machinery to repress EPA1 expression in Candida glabrata. Genetics (2012) 190:1285–97. doi:10.1534/genetics.111.138099
97. Hawser SP, Douglas LJ. Resistance of Candida albicans biofilms to antifungal agents in vitro. Antimicrob Agents Chemother (1995) 39:2128–31. doi:10.1128/AAC.39.9.2128
98. Kuhn DM, George T, Chandra J, Mukherjee PK, Ghannoum MA. Antifungal susceptibility of Candida biofilms: unique efficacy of amphotericin B lipid formulations and echinocandins. Antimicrob Agents Chemother (2002) 46:1773–80. doi:10.1128/AAC.46.6.1773-1780.2002
99. Nett J, Lincoln L, Marchillo K, Massey R, Holoyda K, Hoff B, et al. Putative role of β-1,3 glucans in Candida albicans biofilm resistance. Antimicrob Agents Chemother (2007) 51:510–20. doi:10.1128/AAC.01056-06
100. Song JW, Shin JH, Kee SJ, Kim SH, Shin MG, Suh SP, et al. Expression of CgCDR1, CgCDR2, and CgERG11 in Candida glabrata biofilms formed by bloodstream isolates. Med Mycol (2009) 47:545–8. doi:10.1080/13693780802210726
101. Santos R, Costa C, Mil-Homens D, Romão D, de Carvalho CC, Pais P, et al. The multidrug resistance transporters CgTpo1_1 and CgTpo1_2 play a role in virulence and biofilm formation in the human pathogen Candida glabrata. Cell Microbiol (2017) 19:1–13. doi:10.1111/cmi.12686
102. Pais P, Costa C, Pires C, Shimizu K, Chibana H, Teixeira MC. Membrane proteome-wide response to the antifungal drug clotrimazole in Candida glabrata: role of the transcription factor CgPdr1 and the drug:H+ antiporters CgTpo1_1 and CgTpo1_2. Mol Cell Proteomics (2016) 15:57–72. doi:10.1074/mcp.M114.045344
103. Seneviratne CJ, Wang Y, Jin L, Abiko Y, Samaranayake LP. Proteomics of drug resistance in Candida glabrata biofilms. Proteomics (2010) 10:1444–54. doi:10.1002/pmic.200900611
104. Vediyappan G, Rossignol T, D’Enfert C. Interaction of Candida albicans biofilms with antifungals: transcriptional response and binding of antifungals to beta-glucans. Antimicrob Agents Chemother (2010) 54:2096–111. doi:10.1128/AAC.01638-09
105. Lewis K. Persister cells, dormancy and infectious disease. Nat Rev Microbiol (2007) 5:48–56. doi:10.1038/nrmicro1557
106. Lafleur MD, Kumamoto CA, Lewis K. Candida albicans biofilms produce antifungal-tolerant persister cells. Antimicrob Agents Chemother (2006) 50:3839–46. doi:10.1128/AAC.00684-06
107. Elias S, Banin E. Multi-species biofilms: living with friendly neighbors. FEMS Microbiol Rev (2012) 36:990–1004. doi:10.1111/j.1574-6976.2012.00325.x
108. Pathak AK, Sharma S, Shrivastva P. Multi-species biofilm of Candida albicans and non-Candida albicans Candida species on acrylic substrate. J Appl Oral Sci (2012) 20:70–5. doi:10.1590/S1678-77572012000100013
109. Cavalcanti YW, Morse DJ, da Silva WJ, Del-Bel-Cury AA, Wei X, Wilson M, et al. Virulence and pathogenicity of Candida albicans is enhanced in biofilms containing oral bacteria. Biofouling (2015) 31:27–38. doi:10.1080/08927014.2014.996143
110. Zhang K, Ren B, Zhou X, Xu HH, Chen Y, Han Q, et al. Effect of antimicrobial denture base resin on multi-species biofilm formation. Int J Mol Sci (2016) 17:1–13. doi:10.3390/ijms17071033
111. Harriott MM, Noverr MC. Candida albicans and Staphylococcus aureus form polymicrobial biofilms: effects on antimicrobial resistance. Antimicrob Agents Chemother (2009) 53:3914–22. doi:10.1128/AAC.00657-09
112. Harriott MM, Noverr MC. Ability of Candida albicans mutants to induce Staphylococcus aureus vancomycin resistance during polymicrobial biofilm formation. Antimicrob Agents Chemother (2010) 54:3746–55. doi:10.1128/AAC.00573-10
113. Adam B, Baillie GS, Douglas LJ. Mixed species biofilms of Candida albicans and Staphylococcus epidermidis. J Med Microbiol (2002) 51:344–9. doi:10.1099/0022-1317-51-4-344
114. Bamford CV, d’Mello A, Nobbs AH, Dutton LC, Vickerman MM, Jenkinson HF. Streptococcus gordonii modulates Candida albicans biofilm formation through intergeneric communication. Infect Immun (2009) 77:3696–704. doi:10.1128/IAI.00438-09
115. Jenkinson HF, Lala HC, Shepherd MG. Coaggregation of Streptococcus sanguis and other Streptococci with Candida albicans. Infect Immun (1990) 58:1429–36.
116. Silverman RJ, Nobbs AH, Vickerman MM, Barbour ME, Jenkinson HF. Interaction of Candida albicans cell wall Als3 protein with Streptococcus gordonii SspB adhesin promotes development of mixed-species communities. Infect Immun (2010) 78:4644–52. doi:10.1128/IAI.00685-10
117. De Keersmaecker SCJ, Sonck K, Vanderleyden J. Let LuxS speak up in AI-2 signaling. Trends Microbiol (2006) 14:114–9. doi:10.1016/j.tim.2006.01.003
118. Hornby JM, Jensen EC, Lisec AD, Tasto JJ, Jahnke B, Shoemaker R, et al. Quorum sensing in the dimorphic fungus Candida albicans is mediated by farnesol. Appl Environ Microbiol (2001) 67:2982–92. doi:10.1128/AEM.67.7.2982-2992.2001
119. Riekhof WR, Nickerson KW. Quorum sensing in Candida albicans: farnesol versus farnesoic acid. FEBS Lett (2017) 591:1637–40. doi:10.1002/1873-3468.12694
120. Polke M, Leonhardt I, Kurzai O, Jacobsen ID. Farnesol signalling in Candida albicans – more than just communication. Crit Rev Microbiol (2017) 44:1–14. doi:10.1080/1040841X.2017.1337711
121. Ramage G, Saville SP, Wickes BL, López-Ribot JL. Inhibition of Candida albicans biofilm formation by farnesol, a quorum-sensing molecule. Appl Environ Microbiol (2002) 68:5459–63. doi:10.1128/AEM.68.11.5459-5463.2002
122. Alem MAS, Oteef MDY, Flowers TH, Douglas LJ. Production of tyrosol by Candida albicans biofilms and its role in quorum sensing and biofilm development. Eukaryot Cell (2006) 5:1770–9. doi:10.1128/EC.00219-06
123. Weber K, Schulz B, Ruhnke M. The quorum-sensing molecule E,E-farnesol – its variable secretion and its impact on the growth and metabolism of Candida species. Yeast (2010) 27:727–39. doi:10.1002/yea.1769
124. Katragkou A, McCarthy M, Alexander EL, Antachopoulos C, Meletiadis J, Jabra-Rizk MA, et al. In vitro interactions between farnesol and fluconazole, amphotericin B or micafungin against Candida albicans biofilms. J Antimicrob Chemother (2015) 70:470–8. doi:10.1093/jac/dku374
125. Rossignol T, Logue ME, Reynolds K, Grenon M, Lowndes NF, Butler G. Transcriptional response of Candida parapsilosis following exposure to farnesol. Antimicrob Agents Chemother (2007) 51:2304–12. doi:10.1128/AAC.01438-06
126. Laffey SF, Butler G. Phenotype switching affects biofilm formation by Candida parapsilosis. Microbiology (2005) 151:1073–81. doi:10.1099/mic.0.27739-0
127. Kruppa M. Quorum sensing and Candida albicans. Mycoses (2008) 52:1–10. doi:10.1111/j.1439-0507.2008.01626.x
128. Chen H, Fujita M, Feng Q, Clardy J, Fink GR. Tyrosol is a quorum-sensing molecule in Candida albicans. Proc Natl Acad Sci U S A (2004) 101:5048–52. doi:10.1073/pnas.0401416101
129. Semighini CP, Hornby JM, Dumitru R, Nickerson KW, Harris SD. Farnesol-induced apoptosis in Aspergillus nidulans reveals a possible mechanism for antagonistic interactions between fungi. Mol Microbiol (2006) 59:753–64. doi:10.1111/j.1365-2958.2005.04976.x
130. Hogan DA, Kolter R. Pseudomonas-Candida interactions: an ecological role for virulence factors. Science (2002) 296:2229–32. doi:10.1126/science.1070784
131. Holcombe LJ, McAlester G, Munro CA, Enjalbert B, Brown AJP, Gow NAR, et al. Pseudomonas aeruginosa secreted factors impair biofilm development in Candida albicans. Microbiology (2010) 156:1476–85. doi:10.1099/mic.0.037549-0
132. Hogan DA, Vik Å, Kolter R. A Pseudomonas aeruginosa quorum-sensing molecule influences Candida albicans morphology. Mol Microbiol (2004) 54:1212–23. doi:10.1111/j.1365-2958.2004.04349.x
133. Cugini C, Calfee MW, Farrow JM III, Morales DK, Pesci EC, Hogan DA. Farnesol, a common sesquiterpene, inhibits PQS production in Pseudomonas aeruginosa. Mol Microbiol (2007) 65:896–906. doi:10.1111/j.1365-2958.2007.05840.x
134. Bandara H, Yau JYY, Watt RM, Jin LJ, Samaranayake LP. Pseudomonas aeruginosa inhibits in-vitro Candida biofilm development. BMC Microbiol (2010) 10:125. doi:10.1186/1471-2180-10-125
135. Kerr JR. Suppression of fungal growth exhibited by Pseudomonas aeruginosa. J Clin Microbiol (1994) 32:525–7.
136. Kaleli I, Cevahir N, Demir M, Yildirim U, Sahin R. Anticandidal activity of Pseudomonas aeruginosa strains isolated from clinical specimens. Mycoses (2006) 50:74–8. doi:10.1111/j.1439-0507.2006.01322.x
137. Carratalà J. The antibiotic-lock technique for therapy of ‘highly needed’ infected catheters. Clin Microbiol Infect (2002) 8:282–9. doi:10.1046/j.1469-0691.2002.00388.x
138. Cateau E, Berjeaud JM, Imbert C. Possible role of azole and echinocandin lock solutions in the control of Candida biofilms associated with silicone. Int J Antimicrob Agents (2011) 37:380–4. doi:10.1016/j.ijantimicag.2010.12.016
139. Toulet D, Debarre C, Imbert C. Could liposomal amphotericin B (L-AMB) lock solutions be useful to inhibit Candida spp. biofilms on silicone biomaterials? J Antimicrob Chemother (2012) 67:430–2. doi:10.1093/jac/dkr473
140. Piersigilli F, Auriti C, Bersani I, Goffredo B, Bianco G, Savarese I, et al. Antifungal lock therapy with combined 70% ethanol and micafungin in a critically ill infant. Pediatr Infect Dis J (2014) 33:419–20. doi:10.1097/INF.0000000000000116
141. Bonne S, Mazuski JE, Sona C, Schallom M, Boyle W, Buchman TG, et al. Effectiveness of minocycline and rifampin vs chlorhexidine and silver sulfadiazine-impregnated central venous catheters in preventing central line-associated bloodstream infection in a high-volume academic intensive care unit: a before and after trial. J Am Coll Surg (2015) 221:739–47. doi:10.1016/j.jamcollsurg.2015.05.013
142. Villard N, Seneviratne C, Tsoi JKH, Heinonen M, Matinlinna J. Candida albicans aspects of novel silane system-coated titanium and zirconia implant surfaces. Clin Oral Implants Res (2015) 26:332–41. doi:10.1111/clr.12338
143. Raut JS, Shinde RB, Chauhan NM, Karuppayil SM. Phenylpropanoids of plant origin as inhibitors of biofilm formation by Candida albicans. J Microbiol Biotechnol (2014) 24:1216–25. doi:10.4014/jmb.1402.02056
144. Raut JS, Shinde RB, Chauhan NM, Mohan Karuppayil S. Terpenoids of plant origin inhibit morphogenesis, adhesion, and biofilm formation by Candida albicans. Biofouling (2013) 29:87–96. doi:10.1080/08927014.2012.749398
145. Morales DK, Grahl N, Okegbe C, Dietrich LE, Jacobs NJ, Hogan DA. Control of Candida albicans metabolism and biofilm formation by Pseudomonas aeruginosa phenazines. MBio (2013) 4:1–9. doi:10.1128/mBio.00526-12
146. Wong SS, Kao RY, Yuen KY, Wang Y, Yang D, Samaranayake LP, et al. In vitro and in vivo activity of a novel antifungal small molecule against Candida infections. PLoS One (2014) 9:e85836. doi:10.1371/journal.pone.0085836
147. Theberge S, Semlali A, Alamri A, Leung KP, Rouabhia M. C. albicans growth, transition, biofilm formation, and gene expression modulation by antimicrobial decapeptide KSL-W. BMC Microbiol (2013) 13:246. doi:10.1186/1471-2180-13-246
148. Karlsson AJ, Flessner RM, Gellman SH, Lynn DM, Palecek SP. Polyelectrolyte multilayers fabricated from antifungal β-peptides: design of surfaces that exhibit antifungal activity against Candida albicans. Biomacromolecules (2010) 11:2321–8. doi:10.1021/bm100424s
149. Hoque J, Akkapeddi P, Yadav V, Manjunath GB, Uppu DS, Konai MM, et al. Broad spectrum antibacterial and antifungal polymeric paint materials: synthesis, structure-activity relationship, and membrane-active mode of action. ACS Appl Mater Interfaces (2015) 7:1804–15. doi:10.1021/am507482y
150. Silva-Dias A, Palmeira-de-Oliveira A, Miranda IM, Branco J, Cobrado L, Monteiro-Soares M, et al. Anti-biofilm activity of low-molecular weight chitosan hydrogel against Candida species. Med Microbiol Immunol (2014) 203:25–33. doi:10.1007/s00430-013-0311-4
151. Stepanovic S, Vukovic D, Jesic M, Ranin L. Influence of acetylsalicylic acid (aspirin) on biofilm production by Candida species. J Chemother (2004) 16:134–8. doi:10.1179/joc.2004.16.2.134
152. Rosseti IB, Chagas LR, Costa MS. Photodynamic antimicrobial chemotherapy (PACT) inhibits biofilm formation by Candida albicans, increasing both ROS production and membrane permeability. Lasers Med Sci (2014) 29:1059–64. doi:10.1007/s10103-013-1473-4
153. Černáková L, Chupáčová J, Židlíková K, Bujdáková H. Effectiveness of the photoactive dye methylene blue versus caspofungin on the Candida parapsilosis biofilm in vitro and ex vivo. Photochem Photobiol (2015) 91:1181–90. doi:10.1111/php.12480
154. Chien HF, Chen CP, Chen YC, Chang PH, Tsai T, Chen CT. The use of chitosan to enhance photodynamic inactivation against Candida albicans and its drug-resistant clinical isolates. Int J Mol Sci (2013) 14:7445–56. doi:10.3390/ijms14047445
Keywords: Candida genus, biofilm formation, biofilm regulators, antibiofilm strategies, antifungal resistance, multi-species biofilm
Citation: Cavalheiro M and Teixeira MC (2018) Candida Biofilms: Threats, Challenges, and Promising Strategies. Front. Med. 5:28. doi: 10.3389/fmed.2018.00028
Received: 18 December 2017; Accepted: 26 January 2018;
Published: 13 February 2018
Edited by:
Marc Thilo Figge, Leibniz-Institut für Naturstoff-Forschung und Infektionsbiologie, Hans Knöll Institut, GermanyReviewed by:
Slavena Vylkova, Friedrich Schiller Universität Jena, GermanyCopyright: © 2018 Cavalheiro and Teixeira. This is an open-access article distributed under the terms of the Creative Commons Attribution License (CC BY). The use, distribution or reproduction in other forums is permitted, provided the original author(s) and the copyright owner are credited and that the original publication in this journal is cited, in accordance with accepted academic practice. No use, distribution or reproduction is permitted which does not comply with these terms.
*Correspondence: Miguel Cacho Teixeira, bW5wY3RAdGVjbmljby51bGlzYm9hLnB0
Disclaimer: All claims expressed in this article are solely those of the authors and do not necessarily represent those of their affiliated organizations, or those of the publisher, the editors and the reviewers. Any product that may be evaluated in this article or claim that may be made by its manufacturer is not guaranteed or endorsed by the publisher.
Research integrity at Frontiers
Learn more about the work of our research integrity team to safeguard the quality of each article we publish.