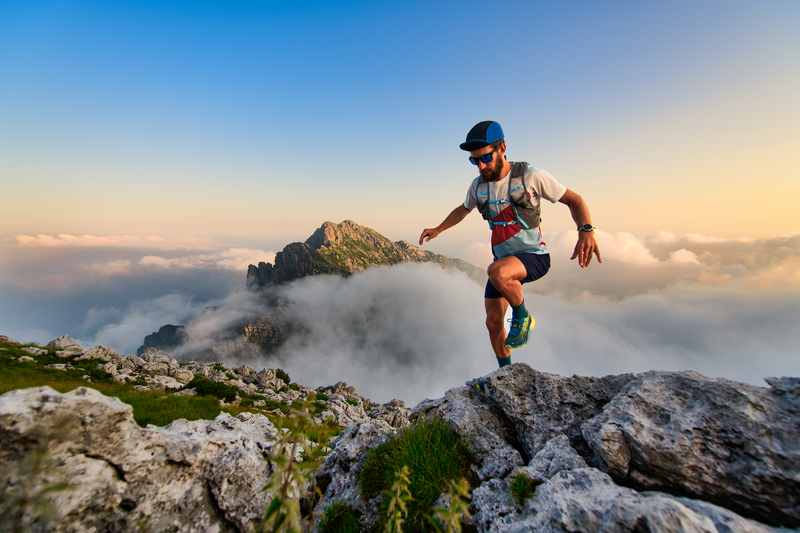
94% of researchers rate our articles as excellent or good
Learn more about the work of our research integrity team to safeguard the quality of each article we publish.
Find out more
PERSPECTIVE article
Front. Mech. Eng. , 12 May 2022
Sec. Engine and Automotive Engineering
Volume 8 - 2022 | https://doi.org/10.3389/fmech.2022.903379
We refer to the fourth state of matter as plasma, indicating ionized, electrically quasi-neutral gas. Electrical discharge in a gas medium is a normal and easy way of turning the gas into plasma in a moderate pressure condition. The electron temperature, electron density, and gas temperature characterize a quality of plasma. Particularly in the domain in terms of the electron temperature and gas temperature, we have room to design discharges to be a thermal plasma (both electron and gas temperature are in equilibrium) or non-thermal plasma (a couple of orders magnitude higher electron temperature than gas temperature). This indicates that the plasma chemistry, consisting of electron impact reactions and thermochemistry governed by the electron temperature and gas temperature, respectively, can be tailored to a certain extent. In this regard, we believe that plasma technology can be considered as a versatile reaction platform, which can replace and reinforce conventional combustion and catalyst-based ones in an electrified future. This perspective particularly highlights the opportunities for the combustion community in the field of low-temperature plasma technology, elaborating on the leashed potential of plasma chemistry and its similarities with combustion studies.
Numerous mythologies from around the world have undoubtedly shown us the importance of fire throughout humankind’s history. The acquisition, control, or theft of fire has been a recurring theme in many cultures across the globe. The importance of fire in mythology and its divine connotations are hardly surprising. Today, we are all too aware that early human’s ability to wield fire can be considered one of the pivotal moments that ignited the course of human history.
Fire provided our ancestors with the means for cooking, heating, hunting, and protecting, as well as for art and tool-making. Over time control over fire lit the path for three major socio-technological revolutions. Fire became the cornerstone of civilization, beginning with the Neolithic revolution (10,000 B.C.), followed by humankind’s first innovative manufacturing waves during the Bronze (3000–1200 B.C.) and Iron Age (1200–500 B.C.), which are both defined by humankind’s ability to control fire. The third major innovation was the replacement of manpower with man-made power through the development of the steam engine, followed by the invention of the internal combustion engine (late 19th C). These innovations sparked the Industrial Revolution (1760–1840) fueled by coal and oil, and combustion processes became the foundation of power generation, transportation, smelting, and material processing. Advances in petrochemistry rapidly followed, which expanded the applications of oil far beyond combustion, producing fertilizers, materials (like plastics, fiber, and rubber), food, medicine, household goods, and much more. Today almost everything in our day-to-day lives relies on fire and fossil fuels, in terms of the applied science of combustion and petrochemistry.
Although fire has powered unprecedented advancements in society, the devastating effects of unchecked CO2 emissions on our planet’s climate and environment opened a Pandora’s box, releasing fear of a gloomy future on a scorched earth. However, hope arises through our pursuit of promising and logical solutions, such as renewable energy and carbon-free or carbon-neutral fuels (hydrogen, ammonia, biomass, solar fuels, etc.). Even though we cannot abandon fossil fuels entirely just yet because of current energy demands (Kohse-Hoinghaus, 2018), a controlled transition from the era of fire to an electrified future appears imperative.
Since the first commercial power generator, electricity has superseded combustion in many of its initial breakthrough areas. For example, lighting has long since become fully electric, and domestic heating and gas-based cooking appliances have been gradually supplanted by their electric counterparts. Even in the transport sector, the market for electric vehicles (both battery and fuel-cell-based) is growing rapidly. Additionally, the IEA (International Energy Agency) forecasts that electricity will be the primary source of energy for the fourth industrial revolution. Hence, the transition toward an electrified future is already kindling all around us, and we must prepare ourselves for both the challenges and opportunities that electrification presents.
Is it simply a curious coincidence that this modern transition from fire to electricity bears a resemblance to mythology? The Greek God Zeus, who punished Prometheus for giving fire to humankind, symbolized his might through lightning—his ultimate source of power—which, of course, is electricity! And lightning moreover is one of the most common natural plasmas on Earth. Is the use of plasma technology in an electrified future predestined? In fact, low-pressure plasma technology was an unsung hero behind the Information Revolution fabricating IC (Integrated Circuit) chips.
The term ‘plasma’ refers to ionized gas that is in an electrically quasi-neutral state, considered to be the fourth state of matter. The key element to sustain a plasma is the multiplication of its highly energetic electrons through electron impact reactions, which result in the formation of a large variety of species, such as ions, atoms, molecules, radicals, and excited species, often making plasma a highly reactive and complex physicochemical cocktail (Snoeckx and Bogaerts, 2017).
We can distinguish two groups of man-made plasma: fully ionized plasmas, such as thermonuclear fusion plasmas (Bychkov et al., 2015; Freidberg, 2007), and partially ionized plasmas (10−4–10–6) or so-called gas discharges, which are the focus of several disrupting innovations (Figure 1) (Donnelly and Kornblit, 2013; Adamovich et al., 2017; Murphy and Uhrlandt, 2018). Since plasma is a multicomponent system, it can exhibit multiple temperatures. When the temperature of all the species is the same in a localized area, the plasma is said to be in ‘local thermodynamic equilibrium’ (LTE). When the temperature of all the species (particularly electrons, Te, and neural species, Tgas) is characterized by multiple different temperatures, it is said to be in ‘non-local thermodynamic equilibrium’ (non-LTE). The former type of plasmas is usually called “thermal plasmas” and the latter ‘non-thermal plasmas’ (Donnelly and Kornblit, 2013; Adamovich et al., 2017; Murphy and Uhrlandt, 2018).
FIGURE 1. Progress of plasma research and development along with some historical moments in the combustion- and electricity-based technologies.
Regardless of these categorizations, the cascade of energy transfers from the highly energetic electrons to all the other species through collisions leads to an increase in gas temperature. Depending on the conditions, this increase can range from several Kelvin up to temperatures that are more than one order of magnitude greater than what can be achieved through the conventional combustion of fossil fuel (see Figure 2 and Table 1). Particularly, non-thermal plasmas operating at atmospheric pressure, such as Dielectric Barrier Discharges (DBD) (Brandenburg, 2017) and Nanosecond Repetitively Pulsed (NRP) discharges (Huiskamp, 2020; Wang and Namihira, 2020), generate highly energetic electrons with only a minimal increase in the gas temperature (Te >> Tgas). Arc discharges, on the other hand, representing thermal plasmas, typically demonstrate Te = Tgas (∼ 10,000 K) at their core. Between these opposites, elongated arc (including gliding arc) discharges (Fridman et al., 1999) and microwave (MW) discharges (Tiwari et al., 2020) can bridge the wide gap in Tgas, from room temperature up to several thousand Kelvin. It should be also noted that a local Tgas in the discharge channel of NRP discharges can go up to a couple of thousand Kelvin.
FIGURE 2. Plasma characteristics in terms of electron temperature (Te) and gas temperature (Tgas) for the selected discharges at atmospheric pressure.
These properties place plasma in a unique position in an electrified future by providing two almost independent ways to control chemical reactions, allowing for energetic electron-driven chemistry, (extreme) heat-driven chemistry, and any possible combination of the two. Low-pressure non-thermal plasma-based nanoscale semiconductor fabrication is a perfect example of electron-driven chemistry, which has already led us to the dawn of the fourth industrial revolution (Donnelly and Kornblit, 2013; Adamovich et al., 2017), whereas thermal plasma-based arc welding and waste treatment well represents heat-driven plasma processes (Murphy and Uhrlandt, 2018; Sikarwar et al., 2020).
Currently, we burn chemicals (in the form of fossil fuels or biological matter) to generate electrical energy. Plasma has the potential to be a key technology to transform electrical energy into chemical energy, and thus efficiently store electrical energy in a chemical form by synthesizing chemicals and fuels of interest (Snoeckx and Bogaerts, 2017). Plasma technology provides us with the opportunity to utilize the full potential of electrical energy. Energy production is already shifting away from fossil fuels to renewable sources. In the same way, a similar shift from conventional energy sources towards electricity in industrial processes might be envisioned.
Plasmas have already been investigated for hydrocarbon reforming processes, such as partial oxidation, dry reforming, steam reforming, and conversion of pure hydrocarbons (Tao et al., 2011; Wang et al., 2018; le Saché and Reina, 2022). The conversion of CO2, through pure CO2 splitting, CO2 hydrogenation, and artificial photosynthesis have also been studied (Snoeckx and Bogaerts, 2017; Tiwari et al., 2020; Saeidi et al., 2021; Ong et al., 2022). However, despite the wide variety of plasma devices invented, plasma chemistry for higher hydrocarbons (even C2 and C3) with CO2, O2, H2O, and N2 remains a mostly undiscovered domain.
While plasmas excel at initiating reactions, tuning the selectivity remains a major challenge. Recent studies have revealed that while the electron impact reactions can initiate a chemical reaction regardless of initial conditions, the thermochemistry (governed by the gas temperature) has a dominant effect on the product selectivity and thus final product composition (Zhang and Cha, 2015). Another study showed that despite the non-thermodynamic equilibrium nature of non-thermal plasmas, it is possible to influence the product selectivity by changing the inlet composition or adding specific reaction products to shift the chemical equilibrium (Snoeckx et al., 2018). Combining plasma with a catalyst is another route to affect the process selectivity. This approach creates synergistic effects that increase selectivity and yield and/or decrease the light-off temperature (Neyts et al., 2015; Whitehead, 2016; Bogaerts et al., 2020; Lovell et al., 2022). The mechanism of this synergy highly depends on the chemical process, the plasma properties and the type of catalyst; the catalyst can be activated by a combination of heat and/or the species (charges, photons, radicals, intermediates) generated by the plasma.
Future efforts should continue in this direction, focusing on rigorous, fundamental investigations into the plasma chemistry (and possible catalyst interactions), with a final goal to design and develop plasma systems based on knowledge and understanding, where we can optimize the conditions (such as electron temperature and gas temperature) for any target process. Special attention should be given to processes for the production of carbon-free fuels such as hydrogen and ammonia (Winter and Chen, 2021), and the production of feedstock (petro)chemicals like methanol, aldehydes, C2-C3 hydrocarbons (such as ethylene and propylene).
Once control over the product formation and selectivity for plasma processes is fully understood—and further exploited, this can pave the way for using plasmas as a highly flexible platform for the on-demand production of tailored chemicals and fuels using (renewable) electricity. Also, the potential for chemically reactive plasma processes in the future will not remain limited to chemical synthesis and energy applications. Plasmas are already found in several applications in materials science (e.g., coating deposition, surface modification, nanomaterial fabrication), the microelectronics industry (for microchip manufacturing), as light sources, lasers and displays (owing to their light-emitting characteristics), as well as for many emerging environmental (waste, gas and water treatment), food, agriculture, and even medical applications (such as sterilization, wound treatment and even cancer treatment) (Donnelly and Kornblit, 2013; Adamovich et al., 2017).
This perspective shares our views on the unique opportunity to expand the combustion community’s horizons into the electrified future. There are, in fact, many analogies between combustion and plasma processes, especially considering how plasma can be defined as an electrically sustained reacting flow with heat and mass transport, while combustion processes are a thermally sustained reacting flow with heat and mass transport. As a result, they not only share fundamental properties, but they also rely on similar methodologies for their scientific exploration, such as laser diagnostics, chemical kinetics modeling, multi-physics simulation, and much more. If we recall that spark-ignition in fact utilizes plasma, we realize the enormous potential plasma has in complementing combustion (Starikovskiy and Aleksandrov, 2013). Recent advancements in the area of plasma-assisted combustion reveal how we can effectively use plasma to support existing combustion applications (Ju and Sun, 2015; Popov and Starikovskaia, 2022). In particular, plasma-generated radicals and ozone have been found to be crucial in igniting both premixed and non-premixed conditions, by supporting the chain-branching combustion chemistry to jump-start in extreme conditions, yielding extended lean-limits, reduced ignition delay, and increased ignition probability.
Faced with the realities of climate change, the combustion community is fully aware of the major challenges that lie ahead for the energy industry and energy technology (Ghoniem, 2011). As the transition towards an electrified future has already begun in earnest, we could not think of a better time for the combustion community to consider plasma as another, more adaptive, form of fire, and to explore plasma’s potential far beyond its assisted function in combustion. Looking at the similarities in the chemistry between combustion and plasma, and the unexpected as well as expected synergies that are awaiting further exploration, we are convinced that the emerging field of plasma technology has a lot to offer the mature field of energy and combustion science and—perhaps more importantly—vice versa.
The original contributions presented in the study are included in the article/Supplementary Material, further inquiries can be directed to the corresponding author.
MC and RS equally contributed to the preparation of the manuscript.
The authors declare that the research was conducted in the absence of any commercial or financial relationships that could be construed as a potential conflict of interest.
All claims expressed in this article are solely those of the authors and do not necessarily represent those of their affiliated organizations, or those of the publisher, the editors and the reviewers. Any product that may be evaluated in this article, or claim that may be made by its manufacturer, is not guaranteed or endorsed by the publisher.
Adamovich, I., Baalrud, S. D., Bogaerts, A., Bruggeman, P. J., Cappelli, M., Colombo, V., et al. (2017). The 2017 Plasma Roadmap: Low Temperature Plasma Science and Technology. J. Phys. D. Appl. Phys. 50, 323001. doi:10.1088/1361-6463/aa76f5
Bogaerts, A., Tu, X., Whitehead, J. C., Centi, G., Lefferts, L., Guaitella, O., et al. (2020). The 2020 Plasma Catalysis Roadmap. J. Phys. D. Appl. Phys. 53, 443001. doi:10.1088/1361-6463/ab9048
Brandenburg, R. (2017). Dielectric Barrier Discharges: Progress on Plasma Sources and on the Understanding of Regimes and Single Filaments. Plasma Sources Sci. Technol. 26, 053001. doi:10.1088/1361-6595/aa6426
Bychkov, V., Modestov, M., and Law, C. K. (2015). Combustion Phenomena in Modern Physics: I. Inertial Confinement Fusion. Prog. Energy Combust. Sci. 47, 32–59. doi:10.1016/j.pecs.2014.10.001
Donnelly, V. M., and Kornblit, A. (2013). Plasma Etching: Yesterday, Today, and Tomorrow. J. Vac. Sci. Technol. A Vac. Surfaces, Films 31, 050825. doi:10.1116/1.4819316
Fridman, A., Nester, S., Kennedy, L. A., Saveliev, A., and Mutaf-Yardimci, O. (1999). Gliding Arc Gas Discharge. Prog. Energy Combust. Sci. 25, 211–231. doi:10.1016/s0360-1285(98)00021-5
Ghoniem, A. F. (2011). Needs, Resources and Climate Change: Clean and Efficient Conversion Technologies. Prog. Energy Combust. Sci. 37, 15–51. doi:10.1016/j.pecs.2010.02.006
Huiskamp, T. (2020). Nanosecond Pulsed Streamer Discharges Part I: Generation, Source-Plasma Interaction and Energy-Efficiency Optimization. Plasma Sources Sci. Technol. 29, 023002. doi:10.1088/1361-6595/ab53c5
Ju, Y., and Sun, W. (2015). Plasma Assisted Combustion: Dynamics and Chemistry. Prog. Energy Combust. Sci. 48, 21–83. doi:10.1016/j.pecs.2014.12.002
Kohse-Höinghaus, K. (2018). Clean Combustion: Chemistry and Diagnostics for a Systems Approach in Transportation and Energy Conversion. Prog. Energy Combust. Sci. 65, 1–5. doi:10.1016/j.pecs.2017.10.001
le Saché, E., and Reina, T. R. (2022). Analysis of Dry Reforming as Direct Route for Gas Phase CO2 Conversion. The Past, the Present and Future of Catalytic DRM Technologies. Prog. Energy Combust. Sci. 89, 100970.
Lovell, E. C., Scott, J., Bedford, N. M., Tan, T. H., Cullen, P. J., Ostrikov, K. K., et al. (2022). Two Steps Back, One Leap Forward: Synergistic Energy Conversion in Plasmonic and Plasma Catalysis. ACS Energy Lett. 7, 300–309. doi:10.1021/acsenergylett.1c02387
Murphy, A. B., and Uhrlandt, D. (2018). Foundations of High-Pressure Thermal Plasmas. Plasma Sources Sci. Technol. 27, 063001. doi:10.1088/1361-6595/aabdce
Neyts, E. C., Ostrikov, K., Sunkara, M. K., and Bogaerts, A. (2015). Plasma Catalysis: Synergistic Effects at the Nanoscale. Chem. Rev. 115, 13408–13446. doi:10.1021/acs.chemrev.5b00362
Ong, M. Y., Nomanbhay, S., Kusumo, F., and Show, P. L. (2022). Application of Microwave Plasma Technology to Convert Carbon Dioxide (CO2) into High Value Products: A Review. J. Clean. Prod. 336, 130447. doi:10.1016/j.jclepro.2022.130447
Popov, N. A., and Starikovskaia, S. M. (2022). Relaxation of Electronic Excitation in Nitrogen/oxygen and Fuel/air Mixtures: Fast Gas Heating in Plasma-Assisted Ignition and Flame Stabilization. Prog. Energy Combust. Sci., 100928. in press[Online]. doi:10.1016/j.pecs.2021.100928
Roettgen, A., Shkurenkov, I., Simeni Simeni, M., Petrishchev, V., Adamovich, I. V., and Lempert, W. R. (2016). Time-resolved Electron Density and Electron Temperature Measurements in Nanosecond Pulse Discharges in Helium. Plasma Sources Sci. Technol. 25, 055009. doi:10.1088/0963-0252/25/5/055009
Rusterholtz, D. L., Lacoste, D. A., Stancu, G. D., Pai, D. Z., and Laux, C. O. (2013). Ultrafast Heating and Oxygen Dissociation in Atmospheric Pressure Air by Nanosecond Repetitively Pulsed Discharges. J. Phys. D. Appl. Phys. 46, 464010. doi:10.1088/0022-3727/46/46/464010
Saeidi, S., Najari, S., Hessel, V., Wilson, K., Keil, F. J., Concepción, P., et al. (2021). Recent Advances in CO2 Hydrogenation to Value-Added Products - Current Challenges and Future Directions. Prog. Energy Combust. Sci. 85, 100905. doi:10.1016/j.pecs.2021.100905
Sikarwar, V. S., Hrabovský, M., Van Oost, G., Pohořelý, M., and Jeremiáš, M. (2020). Progress in Waste Utilization via Thermal Plasma. Prog. Energy Combust. Sci. 81, 100873. doi:10.1016/j.pecs.2020.100873
Snoeckx, R., and Bogaerts, A. (2017). Plasma Technology - a Novel Solution for CO2 Conversion? Chem. Soc. Rev. 46, 5805–5863. doi:10.1039/c6cs00066e
Snoeckx, R., Wang, W., Zhang, X., Cha, M. S., and Bogaerts, A. (2018). Plasma-based Multi-Reforming for Gas-To-Liquid: Tuning the Plasma Chemistry towards Methanol. Sci. Rep. 8, 15929. doi:10.1038/s41598-018-34359-x
Starikovskiy, A., and Aleksandrov, N. (2013). Plasma-assisted Ignition and Combustion. Prog. Energy Combust. Sci. 39, 61–110. doi:10.1016/j.pecs.2012.05.003
Tao, X., Bai, M., Li, X., Long, H., Shang, S., Yin, Y., et al. (2011). CH4-CO2 Reforming by Plasma - Challenges and Opportunities. Prog. Energy Combust. Sci. 37, 113–124. doi:10.1016/j.pecs.2010.05.001
Tendero, C., Tixier, C., Tristant, P., Desmaison, J., and Leprince, P. (2006). Atmospheric Pressure Plasmas: a Review. Spectrochim. Acta Part B At. Spectrosc. 61, 2–30. doi:10.1016/j.sab.2005.10.003
Tiwari, S., Caiola, A., Bai, X., Lalsare, A., and Hu, J. (2020). Microwave Plasma-Enhanced and Microwave Heated Chemical Reactions. Plasma Chem. Plasma Process 40, 1–23. doi:10.1007/s11090-019-10040-7
Wang, D., and Namihira, T. (2020). Nanosecond Pulsed Streamer Discharges: II. Physics, Discharge Characterization and Plasma Processing. Plasma Sources Sci. Technol. 29, 023001. doi:10.1088/1361-6595/ab5bf6
Wang, W., Snoeckx, R., Zhang, X., Cha, M. S., and Bogaerts, A. (2018). Modeling Plasma-Based CO2 and CH4 Conversion in Mixtures with N2, O2, and H2O: The Bigger Plasma Chemistry Picture. J. Phys. Chem. C 122, 8704–8723. doi:10.1021/acs.jpcc.7b10619
Whitehead, J. C. (2016). Plasma-catalysis: the Known Knowns, the Known Unknowns and the Unknown Unknowns. J. Phys. D. Appl. Phys. 49, 243001. doi:10.1088/0022-3727/49/24/243001
Winter, L. R., and Chen, J. G. (2021). N2 Fixation by Plasma-Activated Processes. Joule 5, 300–315. doi:10.1016/j.joule.2020.11.009
Keywords: plasma chemistry, thermal plasma, nonthermal plasma, combustion, reacting flow
Citation: Cha MS and Snoeckx R (2022) Plasma Technology–Preparing for the Electrified Future. Front. Mech. Eng 8:903379. doi: 10.3389/fmech.2022.903379
Received: 24 March 2022; Accepted: 28 April 2022;
Published: 12 May 2022.
Edited by:
Joseph K. Lefkowitz, Technion Israel Institute of Technology, IsraelReviewed by:
Mariana Amorim Fraga, Federal University of São Paulo, BrazilCopyright © 2022 Cha and Snoeckx. This is an open-access article distributed under the terms of the Creative Commons Attribution License (CC BY). The use, distribution or reproduction in other forums is permitted, provided the original author(s) and the copyright owner(s) are credited and that the original publication in this journal is cited, in accordance with accepted academic practice. No use, distribution or reproduction is permitted which does not comply with these terms.
*Correspondence: Min Suk Cha, bWluc3VrLmNoYUBrYXVzdC5lZHUuc2E=
Disclaimer: All claims expressed in this article are solely those of the authors and do not necessarily represent those of their affiliated organizations, or those of the publisher, the editors and the reviewers. Any product that may be evaluated in this article or claim that may be made by its manufacturer is not guaranteed or endorsed by the publisher.
Research integrity at Frontiers
Learn more about the work of our research integrity team to safeguard the quality of each article we publish.