- 1Mechanical and Aerospace Engineering Department, University of California San Diego, San Diego, CA, United States
- 2Mechanical Engineering Department, San Diego State University, San Diego, CA, United States
A complementary computational and experimental study is carried out on the formation of ultrafine particulate matter in premixed laminar methane air flames. Specifically, soot formation is examined in premixed stretch-stabilized flames to observe soot inception and growth at relatively high flame temperatures common to oxygen enriched applications. Particle size distribution functions (PSDF) measured by mobility sizing show clear trends as the equivalence ratio increases from Φ = 2.2 to Φ = 2.4. For a given equivalence ratio, the measured distribution decreases in median mobility particle size as the maximum flame temperature increases from approximately 1,950–2,050 K. The median mobility particle size is 20 nm or less for all flame conditions studied. The volume fraction decreases with increasing flame temperature for all equivalence ratio conditions. The Φ = 2.2 condition is close to the soot inception limit and both number density and volume fraction decrease monotonically with increasing flame temperature. The higher equivalence ratio conditions show a peak in number density at 2,000 K which may indicate competing soot inception processes are optimized at this temperature. Flame structure computations are carried out using detailed gas-phase combustion chemistry of the Appel, Bockhorn, Frenklach (ABF) model to examine the connection of the observed PSDF to soot precursor chemistry. Agreement between measured and computed flame standoff distances indicates that the ABF model could provide a reasonable prediction of the flame temperature and soot precursor formation for the flames currently studied. To the first order, the trends observed in the measured PSDF could be understood in terms of computed trends for the formation of benzene, naphthalene and other soot precursors. Results of the current study inform particulate matter behavior for methane and natural gas combustion applications at elevated temperature and oxygen enriched conditions.
Introduction
Particulate matter formation is a crucial factor for combustion applications affecting performance (Heywood, 2018), emissions regulations (Jacobson, 2001) and public health (Jacobson et al., 2000). The most prominent particle produced in conventional combustion engines is soot originating from fuel-rich pockets. As such, a major thrust of combustion research is to develop fundamental knowledge of soot formation processes to minimize negative impacts. Natural gas is an important fuel for transportation, heating and process applications with recent boost in the United States from hydraulic fracturing resources (Montgomery and Smith, 2010). Oxygen enriched conditions are often used with natural gas to optimize heat transfer and emissions performance (Shaddix and Williams, 2017). The current study examines soot formation in premixed methane—enriched air flames using modeling and experimental approaches. Insights into soot inception and growth are gained for these conditions by measuring particle size distributions in the smallest particle size range. Studies on flame structure effects (Xu et al., 1997; Alfè et al., 2010), parent fuel effects (Slavinskaya and Frank, 2009; Sirignano et al., 2011; Russo et al., 2013), and soot inception (Desgroux et al., 2017; Mouton et al., 2013; D’Anna et al., 2008) have been reported for premixed methane flames but the current study focuses on ultrafine (<100 nm) particle formation at elevated flame temperatures under oxygen enrichment. Maricq introduced probe sampling methods and addressed challenges for analysis of mobility size distributions in premixed flames (Maricq et al., 2003; Maricq, 2004; Maricq, 2006). Ever since several investigators have provided useful particle size experimental observations including extensions into particle sizes approaching 1 nm (Wang et al., 2014; Carbone et al., 2016; Carbone et al., 2017a; Carbone et al., 2017b; Tang et al., 2017; Larriba-Andaluz and Carbone, 2021). The current work uses the latest TSI mobility sizing system to examine mobility size down to 1 nm.
Premixed stretch-stabilized flames are established by an aerodynamic balance rather than anchoring by heat loss (Law, 2006) which enables temperatures approaching the adiabatic flame temperature. This configuration is applied in the current study to examine soot formation at elevated temperatures common under oxygen enrichment. Stretch-stabilized stagnation flames also enable systematic probe sampling under well-defined boundary conditions (Camacho et al., 2017; Bonpua et al., 2019; Dasappa and Camacho, 2021a; Dasappa and Camacho, 2021b). Flow perturbations at the sampling orifice are unavoidable but the stagnation surface is an explicit boundary that facilitates complementary modeling to account for probe effects on measured properties (Camacho et al., 2015a; Saggese et al., 2016; Brunnenmeyer and Camacho, 2019). The time for particle growth could be reduced by up to 20% in these flames depending on the orifice and applied pressure drop (Dasappa and Camacho, 2021c). With this in mind, flame structure modeling is carried out to interpret the observed soot formation behavior in terms of flame structure effects and soot precursor chemistry.
Materials and Methods
Experimental Methods
A series of methane-air flames with oxygen enrichment is designed to systematically observe the effects of flame temperature and equivalence ratio on soot formation in premixed laminar flames. Flame conditions spanning 2.2 < Φ < 2.4 and 1,965 K < Tf,max < 2,095 K are summarized in Table 1. The nozzle-to-stagnation surface separation distance is L = 1.5 cm for all flames studied and the computed particle time (tp ∼ 15 ms) is also comparable for all flames. The particle time can be considered to be the residence time for a particle nucleated at the flame zone and sampled at the stagnation surface. For a given equivalence ratio, the flame temperature is adjusted by independently adjusting the flow rate of diluent nitrogen gas fed to the flame. The experimental setup, summarized in Figure 1, centers upon an aerodynamic nozzle (Dnozzle = 1.43 cm) which issues the premixed fuel/air flow. The nozzle takes contours defined by Bergthorson (Bergthorson, 2005) to induce a plug flow at the nozzle boundary. A concentric flow of nitrogen surrounds the flame to reduce perturbation from the surrounding environment. The temperature at the nozzle and stagnation surface boundary are monitored by Type K thermocouples with temperatures maintained at Tnozzle = 330 ± 20 K and Tstagnation = 473 ± 20 K. Calibrated critical orifices are used to control all gas flow rates with oxygen enrichment established by using independent oxygen and nitrogen gas supply.
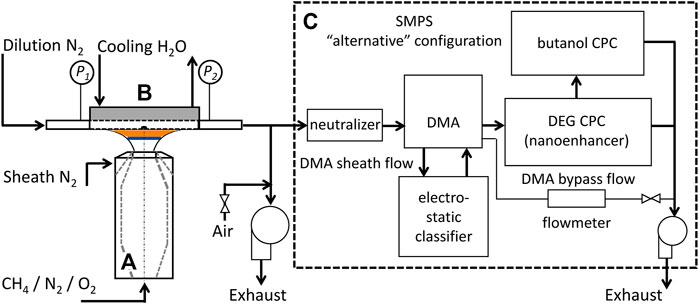
FIGURE 1. Experimental setup including an aerodynamic nozzle for premixed flames (A), a stagnation surface/sampling probe assembly (B), and a TSI SMPS system in the compact configuration (C).
A sample probe with orifice diameter of 130 micron is embedded into the stagnation surface with sampling procedures established to minimize particle diffusion losses and artificial coagulation (Abid et al., 2009a; Cain et al., 2011; Camacho et al., 2013; Puduppakkam et al., 2014; Camacho et al., 2015b; Saggese et al., 2019; Dasappa and Camacho, 2021d). Particle size is measured by mobility sizing using a TSI 1 nm Scanning Mobility Particle Sizer (TSI 3838E77, SMPS) in the “compact” configuration to minimize diffusion loss of the smallest particles. This SMPS system contains a dual voltage classifier (TSI 3082), a Kr-85 bi-polar diffusion charger (Neutralizer TSI 3077A), 1 nm differential mobility analyzer (DMA) (TSI 3086), a diethylene glycol-based (DEG) condensation particle counter (CPC) (so-called Nanoenhancer, TSI 3777) and a butanol-based CPC (TSI 3772). TSI Aerosol Instrument Manager Software (version 10.2) is used to collect and export the measured PSDFs. An insert supplied by the vendor is now mounted onto the inlet of the neutralizer to minimize flow recirculation for more predictable attainment of the equilibrium charge distribution. TSI also measured the penetration of ultra-fine particles through the system flow path recently and a new diffusion loss correction (TSI, 2019) is applied to the measured particle size distribution function (PSDF). The mobility size is corrected to properly account for the transition in gas-particle collision regimes for ultra-fine soot particles (Li and Wang, 2003). The dilution ratio is calibrated using independent flow-meter and CO2 detector measurements. As discussed previously (Zhao et al., 2003; Abid et al., 2009b; Camacho et al., 2015a; Gu et al., 2016; Lin et al., 2016), the dilution ratio is correlated to indicated pressure drop at the sample probe dilution flow inlet and outlet. The dilution ratio applied to all flames is 2,100 for the current study. The flame position is determined experimentally by analysis of flame projection images obtained from a Nikon D5300 DSLR camera.
Computational Methods
Premixed stretch-stabilized flames are a relatively simple axisymmetric flow field that can be solved using a similarity solution (Von Karman, 1921; Seshadri and Williams, 1978; Smooke et al., 1988; Kee et al., 1989a). Previous studies have indicated that the similarity solution is reasonably accurate for stretch-stabilized flames with a wide range of nozzle-to-stagnation surface separations (Saggese et al., 2015; Yapp et al., 2015; Liu et al., 2018; Dasappa and Camacho, 2020). The OPPDIF flame solver (Lutz et al., 1997) is used in the current study to compute the flame structure with a similarity solution in the Chemkin framework (Kee et al., 1989b). Detailed gas-phase combustion chemistry and transport is modeled with the Appel, Bockhorn, Frenklach (ABF) model (Appel et al., 2000) to examine flame structure and the effect of flame structure on production of PAH soot precursors. The current computations do not consider soot formation processes because extensive development of chemical reversibility, graphitization and other processes at elevated flame temperatures will be left to future work.
Results and Discussion
Images of the series of flames currently studied are shown in Figure 2. The image is a projection of the axisymmetric stagnation flow with a steady flame position indicated by the thin blue disc. For flames sitting close to the nozzle boundary, non-ideal deviations from the flat disc shape are observed due to the tendency of the flame to anchor. The intensity of the orange luminosity in the post-flame region is an indication of the amount of soot produced in the flame. As expected, the most intense luminosity is observed for the most fuel rich flames.
Measured PSDF for the series of flames currently studied is shown in Figure 3. For a given equivalence ratio, the median mobility particle size decreases as the flame temperature increases. This indicates that soot formation processes are hindered at elevated temperatures due to reversibility in precursor formation and soot growth (Alfè et al., 2010; Abid et al., 2008), increase in OH oxidation (Haynes and Wagner, 1981) and a reduction in coagulation efficiency (D’Alessio et al., 2005; Sirignano and D’Anna, 2013; Pejpichestakul et al., 2018; Raj et al., 2010). For comparable flame temperature, the median mobility diameter increases over a factor of two from
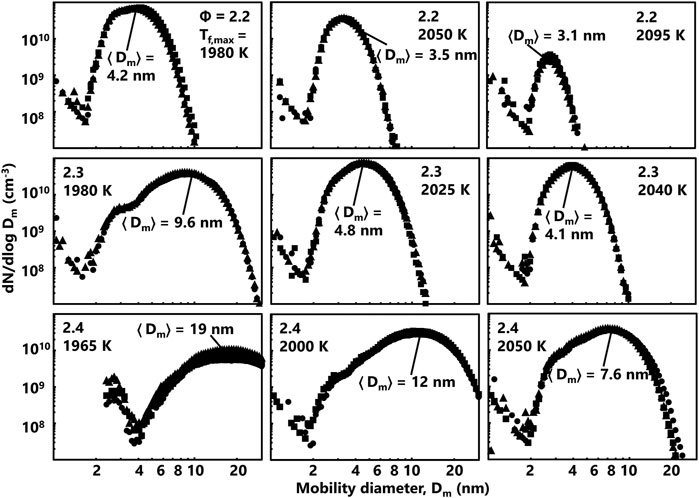
FIGURE 3. Measured PSDF for the series of flames currently studied. The PSDF measurement is repeated three times and different symbols are used to highlight repeated measurements. The median diameter, <Dm>, is labeled for each flame condition as well.
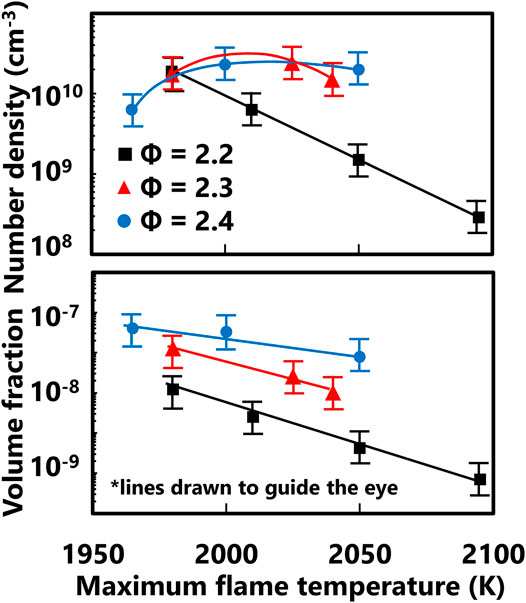
FIGURE 4. Global particle distribution properties derived from the measured PSDF. Lines are drawn to guide the eye.
Complementary flame structure calculations are carried out for the series of flames currently studied. The flame standoff distance is considered to be the distance from the blue flame disc to the stagnation surface. This is measured in the current study from analysis of pixel intensities in the flame projection images. The computed flame standoff distance is considered to be the location of the peak CH* concentration in the centerline axial profile. A comparison between measured and computed values is shown in Figure 5. Reasonable agreement is observed as long as the flame does not approach the vicinity of the nozzle (high standoff distance). As discussed above, the flame disc distorts significantly for flame position close to the nozzle due to the tendency to anchor onto any solid surface. This effect causes a significant disagreement between the measured flame standoff distance for the Φ = 2.2, Tf,max = 2,095 K condition. Reasonable agreement for the other conditions indicates that the ABF combustion chemistry model reasonably predicts the fuel burn rate and flame temperature. The delicate kinematic balance between opposing burning and flow velocity determines the flame position and the ABF model is able to capture the experimental behavior.
With the performance of the ABF model established for the current flames, the flame structure calculations could provide insight into temperature and flame chemistry effects. The computed flame structure for the Φ = 2.2, Tf,max = 1,980 K case is shown in Figure 6 to demonstrate the profiles for a typical premixed stretch-stabilized flame. The axial temperature profile shows the sharp temperature increase at the flame reaction zone significantly downstream of the nozzle exit. The axial convective velocity also increases at the flame and falls down to zero at the stagnation surface. The thermophoretic velocity of the soot particles is calculated based on the assumption that the particles follow the gas streamlines and the hard sphere approximation applies. Assuming inception of the first particles occurs at the flame reaction zone, the time for the particle to traverse the flame zone to the stagnation surface is comparable across all flames (see Table 1). The computed major species for this fuel rich flame show CO production is much higher than CO2 but H2 and H2O are produced at comparable levels. Computed profiles for light and heavy soot precursors are shown in Figure 7 for the series of flames currently studied. As Table 1 shows, the computed particle residence times are all within 13% which effectively minimizes growth time effects on the measured PSDF and computed species profiles. Interestingly, the predicted concentrations of acetylene and propargyl radical are not substantially different across the current range of equivalence ratio and flame temperature. In contrast, the computed profiles for benzene and PAH predict concentrations show some sensitivity to the flame temperature. The measured volume fraction decreases with increasing flame temperature for all equivalence ratio series but the trends for computed PAH are not strong in all cases. For the Φ = 2.2 case, the computed A1-A4 profiles show a clear decreasing trend with increasing flame temperature. This downward trend is generally true for the Φ = 2.3 case but narrower range in flame temperature results in a narrower spread in predicted concentrations. The highest equivalence ratio conditions show a relatively weak sensitivity of the computed A1-A4 mole fractions even though the predicted range of temperatures is wider.
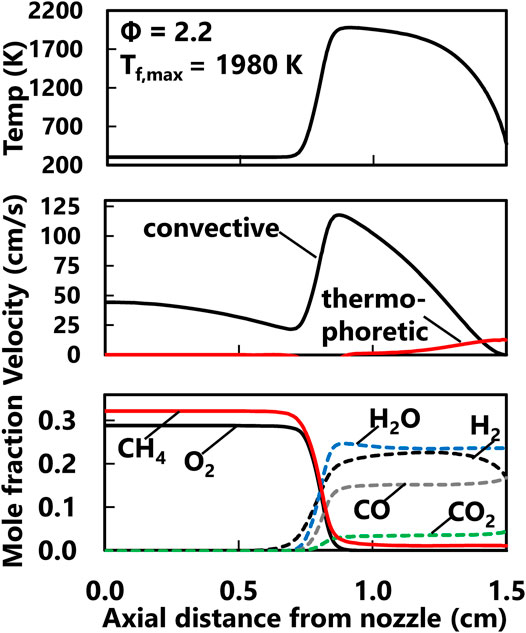
FIGURE 6. Computed axial temperature profile (top), axial velocity profiles (middle) and major products (bottom) at the centerline for the Φ = 2.2, Tf,max = 1,980 K flame.
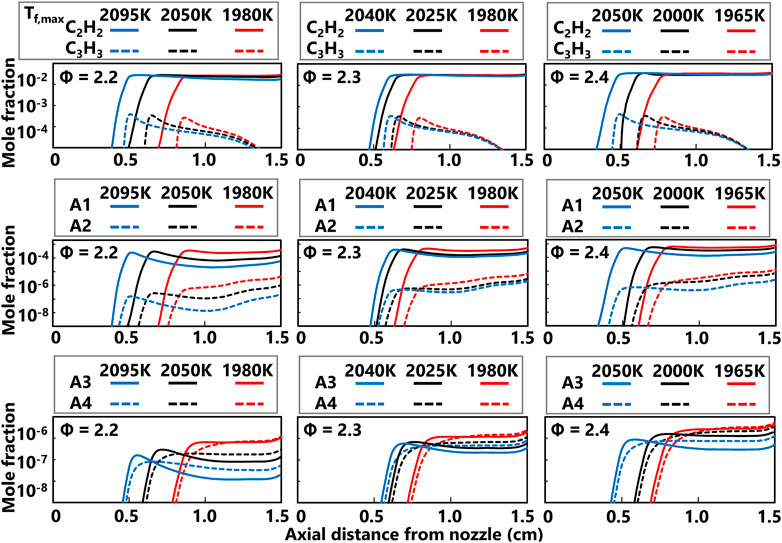
FIGURE 7. Computed centerline axial profiles of soot precursors for the series of flames currently studied. The top row shows the production of acetylene (C2H2) and propargyl radical (C3H3), the middle row shows the production of benzene (A1) and naphthalene (A2), and the bottom row shows the production of phenanthrene (A3) and pyrene (A4).
Reaction rates predicted by the ABF model are also shown here to provide insight into soot precursor formation pathways. Computed profiles for rates of propargyl recombination (2C3H3• = A1) and acetylene + butadienyl (n-C4H5• + C2H2 = A1 + H•) is shown in Figure 8 for four flames spanning the range of equivalence ratios and flame temperatures currently studied. The most significant reaction pathway for benzene production is propargyl recombination for the current series of methane flames. As shown in Figure 8, the C2 pathway is not favored as the reverse reaction consumes a small fraction of benzene in the flame. Predicted production rates for naphthalene based on the A1• + C4H4 = A2 + H• pathway and the A2• + H• = A2 pathway are shown in Figure 9. These two pathways are the most significant for the series currently studied based on the rates predicted by the ABF model. The phenyl radical pathway is slightly slower than the A2 radical pathway but this channel is a culmination of multiple pathways to form the A2 radical. The selected reaction rate profiles for benzene and naphthalene show a modest sensitivity to flame temperature and equivalence ratio.
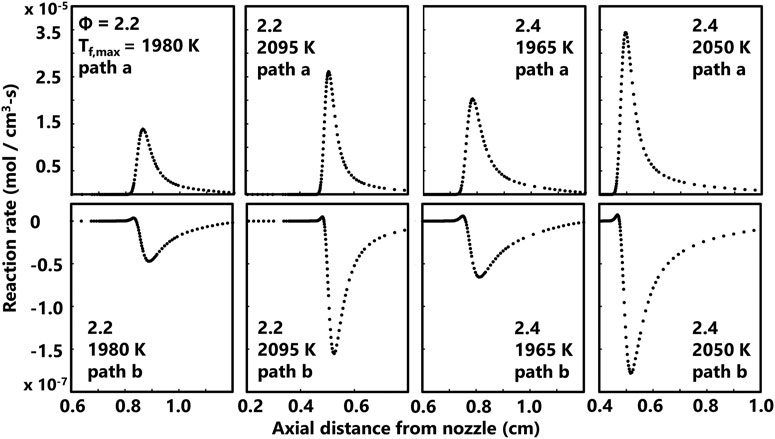
FIGURE 8. Computed rates of two common benzene (A1) production reactions for four flames spanning the range of equivalence ratios and flame temperatures currently studied. The top row is the axial profile for the net rate of 2C3H3• = A1 (path a) and the bottom row is the axial profile for the net rate of n-C4H5• + C2H2 = A1 + H• (path b).
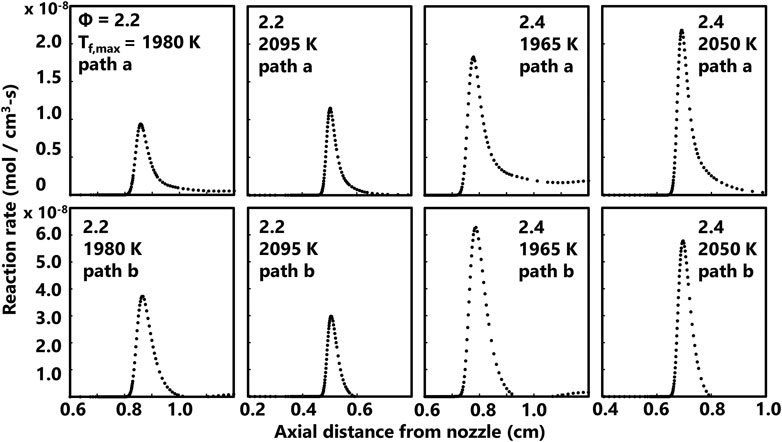
FIGURE 9. Computed rates of two common naphthalene (A2) production reactions for four flames spanning the range of equivalence ratios and flame temperatures currently studied. The top row is the axial profile for the net rate of A1• + C4H4 = A2 + H• (path a) and the bottom row is the axial profile for the net rate of A2• + H• = A2 (path b).
A common soot inception model is to consider pyrene dimerization as the effective onset of soot particles (Frenklach, 2002; Wang, 2011; Totton et al., 2012; Zhang et al., 2014). The ABF model considers four major pathways, summarized in Figure 10, to form pyrene all stemming from phenanthrene species. Phenanthrene radical (A3-4) is postulated to combine with acetylene to either form pyrene directly or to form radicals with unclosed rings. Phenanthrene can also react with C2H radical to form an unclosed ring. For the current series of flames, the ABF model predicts that the direct formation of pyrene from the phenanthrene radical is an order of magnitude faster than the other pathways. Increasing flame temperature also results in somewhat lower rates of pyrene production. The species and reaction rate profiles provide insight into the underlying soot precursor flame chemistry. The experimental PSDF provide a guide for future soot formation modeling in these premixed, enriched air methane flames.
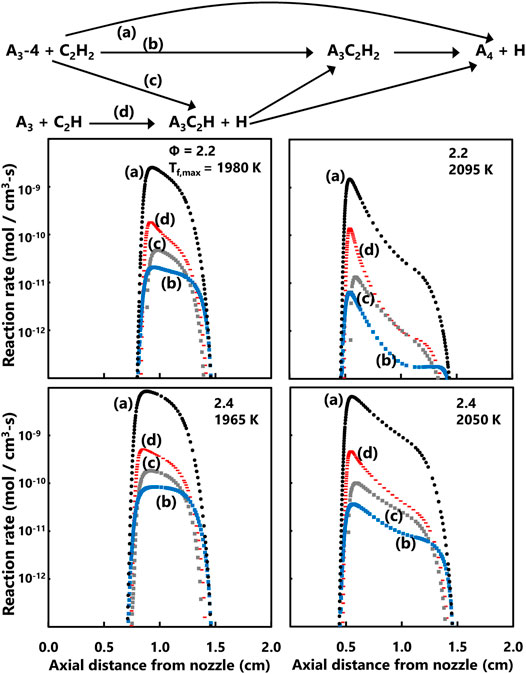
FIGURE 10. Reaction pathways for production of pyrene (A4) considered by the ABF model (top) and axial profiles of computed reaction rates for four main pyrene production pathways (bottom).
Conclusion
Measured PSDF of ultrafine particulate matter formed in premixed methane – air flames are examined with complementary flame structure computations. Relatively high flame temperatures are investigated by using oxygen enrichment and premixed stretch-stabilized flames. For a given equivalence ratio, the measured distribution decreases in median mobility particle size as the maximum flame temperature increases from approximately 1,950–2,050 K. The lowest equivalence ratio, highest temperature flame condition corresponds to a PSDF having a median mobility diameter of 3 nm while the highest equivalence ratio, lowest flame temperature condition corresponds to 20 nm. The soot number density shows a broad peak on the order of 1010 cm−3 for the higher equivalence ratio conditions and decreases to 108 for the lowest equivalence ratio. The measured soot volume fraction shows a monotonic decrease with increasing flame temperature from 10−7.
Flame structure computations are carried out using detailed gas-phase combustion chemistry of the Appel, Bockhorn, Frenklach (ABF) model to examine the connection of the observed PSDF to soot precursor chemistry. Agreement between measured and computed flame standoff distances indicates that the ABF model could provide a reasonable prediction of the flame temperature and soot precursor formation for the flames currently studied. To the first order, the trends observed in the measured PSDF could be understood in terms of the computed trends for the formation of benzene, naphthalene and other soot precursors. Propargyl recombination is the dominant pathway to benzene formation for the current methane flames. According to the ABF model, naphthalene formation occurs by combination of phenyl radical + C4H4 and other pathways leading to naphthalene radical formation. Pyrene formation is predicted to be fastest through direct formation after combination of phenanthrene radical and acetylene. Results of the current study inform particulate matter behavior for methane and natural gas combustion applications at elevated temperature and oxygen enriched conditions.
Data Availability Statement
The raw data supporting the conclusions of this article will be made available by the authors, without undue reservation.
Author Contributions
SD: Conceptualization, methodology, software, investigation, writing—original draft, writing—review and editing, visualization, funding acquisition. JC: Conceptualization, methodology, investigation, writing—original draft, writing—review and editing, visualization, funding acquisition.
Funding
The work was supported by NSF Combustion and Fire Systems program under Award 1841357.
Conflict of Interest
The authors declare that the research was conducted in the absence of any commercial or financial relationships that could be construed as a potential conflict of interest.
Publisher’s Note
All claims expressed in this article are solely those of the authors and do not necessarily represent those of their affiliated organizations, or those of the publisher, the editors and the reviewers. Any product that may be evaluated in this article, or claim that may be made by its manufacturer, is not guaranteed or endorsed by the publisher.
Acknowledgments
Kaylin Dones Sabado aided in the flame collection of the flame images.
References
Abid, A. D., Camacho, J., Sheen, D. a., and Wang, H. (2009). Evolution of Soot Particle Size Distribution Function in Burner-Stabilized Stagnation n-Dodecane−Oxygen−Argon Flames. Energy Fuels 23, 4286–4294. doi:10.1021/ef900324e
Abid, A. D., Camacho, J., Sheen, D. A., and Wang, H. (2009). Quantitative measurement of soot particle size distribution in premixed flames - The burner-stabilized stagnation flame approach. Combustion and Flame 156, 1862–1870. doi:10.1016/j.combustflame.2009.05.010
Abid, A. D., Heinz, N., Tolmachoff, E. D., Phares, D. J., Campbell, C. S., and Wang, H. (2008). On evolution of particle size distribution functions of incipient soot in premixed ethylene-oxygen-argon flames. Combustion and Flame 154, 775–788. doi:10.1016/j.combustflame.2008.06.009
Alfè, M., Apicella, B., Rouzaud, J.-N., Tregrossi, A., and Ciajolo, A. (2010). The effect of temperature on soot properties in premixed methane flames. Combustion and Flame 157, 1959–1965. doi:10.1016/j.combustflame.2010.02.007
Appel, J., Bockhorn, H., and Frenklach, M. (2000). Kinetic modeling of soot formation with detailed chemistry and physics: laminar premixed flames of C2 hydrocarbons. Combustion and Flame 121, 122–136. doi:10.1016/S0010-2180(99)00135-2
Bartos, D., Sirignano, M., Dunn, M. J., D'Anna, A., and Masri, A. R. (2019). Soot inception in laminar coflow diffusion flames. Combustion and Flame 205, 180–192. doi:10.1016/j.combustflame.2019.03.026
Bergthorson, J. M. (2005). Experiments and Modeling of Impinging Jets and Premixed Hydrocarbon Stagnation Flames. California: California Institute of Technology.
Böhm, H., Hesse, D., Jander, H., Lüers, B., Pietscher, J., Wagner, H. G. G., et al. (1989). The influence of pressure and temperature on soot formation in premixed flames. Symp. (International) Combustion 22, 403–411. doi:10.1016/S0082-0784(89)80047-5
Bonpua, J., Yagües, Y., Aleshin, A., Dasappa, S., and Camacho, J. (2019). Flame temperature effect on sp2 bonds on nascent carbon nanoparticles formed in premixed flames (T>2100 K): A Raman spectroscopy and particle mobility sizing study, Proc. Combust. Inst. 37, 943–951. doi:10.1016/j.proci.2018.06.124
Bowal, K., Martin, J. W., Misquitta, A. J., and Kraft, M. (2019). Ion-Induced Soot Nucleation Using a New Potential for Curved Aromatics. Combustion Sci. Tech. 191, 747–765. doi:10.1080/00102202.2019.1565496
Cain, J. P., Camacho, J., Phares, D. J., Wang, H., and Laskin, A. (2011). Evidence of aliphatics in nascent soot particles in premixed ethylene flames. Proc. Combustion Inst. 33, 533–540. doi:10.1016/j.proci.2010.06.164
Camacho, J., Lieb, S., and Wang, H. (2013). Evolution of size distribution of nascent soot in n- and i-butanol flames. Proc. Combustion Inst. 34, 1853–1860. doi:10.1016/j.proci.2012.05.100
Camacho, J., Liu, C., Gu, C., Lin, H., Huang, Z., Tang, Q., et al. (2015). Mobility size and mass of nascent soot particles in a benchmark premixed ethylene flame. Combustion and Flame 162, 3810–3822. doi:10.1016/j.combustflame.2015.07.018
Camacho, J., Singh, A. V., Wang, W., Shan, R., Yapp, E. K. Y., Chen, D., et al. (2017). Soot particle size distributions in premixed stretch-stabilized flat ethylene-oxygen-argon flames. Proc. Combustion Inst. 36, 1001–1009. doi:10.1016/j.proci.2016.06.170
Camacho, J., Tao, Y., and Wang, H. (2015). Kinetics of nascent soot oxidation by molecular oxygen in a flow reactor. Proc. Combustion Inst. 35, 1887–1894. doi:10.1016/j.proci.2014.05.095
Carbone, F., Attoui, M., and Gomez, A. (2016). Challenges of measuring nascent soot in flames as evidenced by high-resolution differential mobility analysis. Aerosol Sci. Tech. 50, 740–757. doi:10.1080/02786826.2016.1179715
Carbone, F., Gleason, K., and Gomez, A. (2017). Probing gas-to-particle transition in a moderately sooting atmospheric pressure ethylene/air laminar premixed flame. Part I: gas phase and soot ensemble characterization. Combustion and Flame 181, 315–328. doi:10.1016/j.combustflame.2017.01.029
Carbone, F., Moslih, S., and Gomez, A. (2017). Probing gas-to-particle transition in a moderately sooting atmospheric pressure ethylene/air laminar premixed flame. Part II: Molecular clusters and nascent soot particle size distributions. Combustion and Flame 181, 329–341. doi:10.1016/j.combustflame.2017.02.021
Commodo, M., Tessitore, G., De Falco, G., Bruno, A., Minutolo, P., and D’Anna, A. (2015). Further details on particle inception and growth in premixed flames. Proc. Combustion Inst. 35, 1795–1802. doi:10.1016/j.proci.2014.06.004
D’Alessio, A., Barone, A. C., Cau, R., D’Anna, A., and Minutolo, P. (2005). Surface deposition and coagulation efficiency of combustion generated nanoparticles in the size range from 1 to 10nm. Proc. Combust. Inst. 30, 2595–2603. doi:10.1016/j.proci.2004.08.267
D’Anna, A., Sirignano, M., Commodo, M., Pagliara, R., and Minutolo, P. (2008). An Experimental and Modelling Study of Particulate Formation in Premixed Flames Burning Methane. Combust. Sci. Technol. 180, 950–958. doi:10.1080/00102200801894448
Dasappa, S., and Camacho, J. (2021). Evolution in size and structural order for incipient soot formed at flame temperatures greater than 2100 Kelvin. Fuel 291, 120196. doi:10.1016/j.fuel.2021.120196
Dasappa, S., and Camacho, J. (2020). Formation of nanocrystalline manganese oxide in flames: oxide phase governed by classical nucleation and size-dependent equilibria. CrystEngComm 22, 5509–5521. doi:10.1039/D0CE00734J
Dasappa, S., and Camacho, J. (2021). Fuel molecular structure effect on soot mobility size in premixed C6 hydrocarbon flames. Fuel 300, 120973. doi:10.1016/j.fuel.2021.120973
Dasappa, S., and Camacho, J. (2021). Raman spectroscopy, mobility size and radiative emissions data for soot formed at increasing temperature and equivalence ratio in flames hotter than conventional combustion applications. Data in Brief 36, 107064. doi:10.1016/j.dib.2021.107064
Dasappa, S., and Camacho, J. (2021). Thermodynamic Barrier to Nucleation for Manganese Oxide Nanoparticles Synthesized by High-Temperature Gas-to-Particle Conversion. Energy Fuels 35, 1874–1884. doi:10.1021/acs.energyfuels.0c03662
Desgroux, P., Faccinetto, A., Mercier, X., Mouton, T., Aubagnac Karkar, D., and El Bakali, A. (2017). Comparative study of the soot formation process in a “nucleation” and a “sooting” low pressure premixed methane flame. Combustion and Flame 184, 153–166. doi:10.1016/j.combustflame.2017.05.034
Frenklach, M., Clary, D. W., Gardiner, W. C., and Stein, S. E. (1985). Detailed kinetic modeling of soot formation in shock-tube pyrolysis of acetylene. Symp. (International) Combustion 20, 887–901. doi:10.1016/S0082-0784(85)80578-6
Frenklach, M. (2002). Reaction mechanism of soot formation in flames. Phys. Chem. Chem. Phys. 4, 2028–2037. doi:10.1039/B110045A
Gleason, K., Carbone, F., Sumner, A. J., Drollette, B. D., Plata, D. L., and Gomez, A. (2021). Small aromatic hydrocarbons control the onset of soot nucleation. Combustion and Flame 223, 398–406. doi:10.1016/j.combustflame.2020.08.029
Gu, C., Lin, H., Camacho, J., Lin, B., Shao, C., Li, R., et al. (2016). Particle size distribution of nascent soot in lightly and heavily sooting premixed ethylene flames. Combustion and Flame 165, 177–187. doi:10.1016/j.combustflame.2015.12.002
Haynes, B. S., and Wagner, H. G. (1981). Soot formation. Prog. Energ. Combustion Sci. 7, 229–273. doi:10.1016/0360-1285(81)90001-0
Jacobson, M. C., Hansson, H.-C., Noone, K. J., and Charlson, R. J. (2000). Organic atmospheric aerosols: Review and state of the science. Rev. Geophys. 38, 267–294. doi:10.1029/1998RG000045
Jacobson, M. Z. (2001). Strong radiative heating due to the mixing state of black carbon in atmospheric aerosols. Nature 409, 695–697. doi:10.1038/35055518
Brunnenmeyer, J., and Camacho, J., Calculation of 2D Flame Structure for Premixed Axisymmetric Stagnation Flames with Elevated Flame Temperature and Equivalence Ratio, in: AIAA Scitech 2019 Forum, American Institute of Aeronautics and Astronautics, USA, (2019). doi:10.2514/6.2019-0781
Johansson, K. O., Head-Gordon, M. P., Schrader, P. E., Wilson, K. R., and Michelsen, H. A. (2018). Resonance-stabilized hydrocarbon-radical chain reactions may explain soot inception and growth. Science 361, 997–1000. doi:10.1126/science.aat3417
Kee, R. J., Miller, J. A., Evans, G. H., and Dixon-Lewis, G. (1989). A computational model of the structure and extinction of strained, opposed flow, premixed methane-air flames. Symp. (International) Combustion 22, 1479–1494. doi:10.1016/S0082-0784(89)80158-4
Kee, R. J., Rupley, F. M., and Miller, J. A. (1989). Chemkin-II: A Fortran chemical kinetics package for the analysis of gas-phase chemical kinetics. United States: OSTI. doi:10.2172/5681118
Larriba-Andaluz, C., and Carbone, F. (2021). The size-mobility relationship of ions, aerosols, and other charged particle matter. J. Aerosol Sci. 151, 105659. doi:10.1016/j.jaerosci.2020.105659
Li, Z., and Wang, H. (2003). Drag force, diffusion coefficient, and electric mobility of small particles. II. Application. Phys. Rev. E. 68, 61207. doi:10.1103/physreve.68.061207
Lin, H., Gu, C., Camacho, J., Lin, B., Shao, C., Li, R., et al. (2016). Mobility size distributions of soot in premixed propene flames. Combustion and Flame 172, 365–373. doi:10.1016/j.combustflame.2016.07.002
Liu, C., Camacho, J., and Wang, H. (2018). Phase Equilibrium of TiO 2 Nanocrystals in Flame‐Assisted Chemical Vapor Deposition. ChemPhysChem 19, 180–186. doi:10.1002/cphc.201700962
Lutz, A. E., Kee, R. J., Grcar, J. F., and Rupley, F. M. (1997). OPPDIF: A Fortan program for computing opposed-flow diffusion flames. United States: OSTI. doi:10.2172/568983
Maricq, M. M. (2006). A comparison of soot size and charge distributions from ethane, ethylene, acetylene, and benzene/ethylene premixed flames. Combustion and Flame 144, 730–743. doi:10.1016/j.combustflame.2005.09.007
Maricq, M. M., Harris, S. J., and Szente, J. J. (2003). Soot size distributions in rich premixed ethylene flames. Combustion and Flame 132, 328–342. doi:10.1016/S0010-2180(02)00502-3
Maricq, M. M. (2004). Size and charge of soot particles in rich premixed ethylene flames. Combustion and Flame 137, 340–350. doi:10.1016/j.combustflame.2004.01.013
Montgomery, C. T., and Smith, M. B. (2010). Hydraulic Fracturing: History of an Enduring Technology. J. Pet. Technol. 62, 26–40. doi:10.2118/1210-0026-JPT
Mouton, T., Mercier, X., Wartel, M., Lamoureux, N., and Desgroux, P. (2013). Laser-induced incandescence technique to identify soot nucleation and very small particles in low-pressure methane flames. Appl. Phys. B 112, 369–379. doi:10.1007/s00340-013-5446-x
Pejpichestakul, W., Frassoldati, A., Parente, A., and Faravelli, T. (2018). Kinetic modeling of soot formation in premixed burner-stabilized stagnation ethylene flames at heavily sooting condition. Fuel 234, 199–206. doi:10.1016/j.fuel.2018.07.022
Puduppakkam, K. V., Modak, A. U., Naik, C. V., Camacho, J., Wang, H., and Meeks, E. (2014). A soot chemistry model that captures fuel effects. Proc. ASME Turbo Expo 4B, 11. doi:10.1115/GT2014-27123
Raj, A., Sander, M., Janardhanan, V., and Kraft, M. (2010). A study on the coagulation of polycyclic aromatic hydrocarbon clusters to determine their collision efficiency. Combustion and Flame 157, 523–534. doi:10.1016/j.combustflame.2009.10.003
Russo, C., Alfè, M., Rouzaud, J.-N., Stanzione, F., Tregrossi, A., and Ciajolo, A. (2013). Probing structures of soot formed in premixed flames of methane, ethylene and benzene. Proc. Combustion Inst. 34, 1885–1892. doi:10.1016/j.proci.2012.06.127
Saggese, C., Cuoci, A., Frassoldati, A., Ferrario, S., Camacho, J., Wang, H., et al. (2016). Probe effects in soot sampling from a burner-stabilized stagnation flame. Combustion and Flame 167, 184–197. doi:10.1016/j.combustflame.2016.02.013
Saggese, C., Ferrario, S., Camacho, J., Cuoci, A., Frassoldati, A., Ranzi, E., et al. (2015). Kinetic modeling of particle size distribution of soot in a premixed burner-stabilized stagnation ethylene flame. Combustion and Flame 162, 3356–3369. doi:10.1016/j.combustflame.2015.06.002
Saggese, C., Singh, A. V., Xue, X., Chu, C., Kholghy, M. R., Zhang, T., et al. (2019). The distillation curve and sooting propensity of a typical jet fuel. Fuel 235, 350–362. doi:10.1016/j.fuel.2018.07.099
Schenk, M., Lieb, S., Vieker, H., Beyer, A., Gölzhäuser, A., Wang, H., et al. (2013). Imaging Nanocarbon Materials: Soot Particles in Flames are Not Structurally Homogeneous. ChemPhysChem 14, 3248–3254. doi:10.1002/cphc.201300581
Seshadri, K., and Williams, F. A. (1978). Laminar flow between parallel plates with injection of a reactant at high reynolds number. Int. J. Heat Mass Transfer 21, 251–253. doi:10.1016/0017-9310(78)90230-2
Shaddix, C. R., and Williams, T. C. (2017). The effect of oxygen enrichment on soot formation and thermal radiation in turbulent, non-premixed methane flames. Proc. Combustion Inst. 36, 4051–4059. doi:10.1016/j.proci.2016.06.106
Sirignano, M., Alfè, M., Tregrossi, A., Ciajolo, A., and D'Anna, A. (2011). Experimental and modeling study on the molecular weight distribution and properties of carbon particles in premixed sooting flames. Proc. Combustion Inst. 33, 633–640. doi:10.1016/j.proci.2010.07.065
Sirignano, M., and D’Anna, A. (2013). Coagulation of combustion generated nanoparticles in low and intermediate temperature regimes: An experimental study. Proc. Combustion Inst. 34, 1877–1884. doi:10.1016/j.proci.2012.06.119
Slavinskaya, N. A., and Frank, P. (2009). A modelling study of aromatic soot precursors formation in laminar methane and ethene flames. Combustion and Flame 156, 1705–1722. doi:10.1016/j.combustflame.2009.04.013
Smooke, M. D., Puri, I. K., and Seshadri, K. (1988). A comparison between numerical calculations and experimental measurements of the structure of a counterflow diffusion flame burning diluted methane in diluted air. Symp. (International) Combustion 21, 1783–1792. doi:10.1016/S0082-0784(88)80412-0
Tang, Q., Cai, R., You, X., and Jiang, J. (2017). Nascent soot particle size distributions down to 1 nm from a laminar premixed burner-stabilized stagnation ethylene flame. Proc. Combustion Inst. 36, 993–1000. doi:10.1016/j.proci.2016.08.085
Totton, T. S., Misquitta, A. J., and Kraft, M. (2012). A quantitative study of the clustering of polycyclic aromatic hydrocarbons at high temperatures. Phys. Chem. Chem. Phys. 14, 4081–4094. doi:10.1039/C2CP23008A
TSI (2019). Diffusion correction using TSI’s 1 nm SMPS SYSTEM Model 3938, Application note SMPS-010. US: TSI.
Wang, H. (2011). Formation of nascent soot and other condensed-phase materials in flames. Proc. Combustion Inst. 33, 41–67. doi:10.1016/j.proci.2010.09.009
Wang, Y., Fang, J., Attoui, M., Chadha, T. S., Wang, W.-N., and Biswas, P. (2014). Application of Half Mini DMA for sub 2nm particle size distribution measurement in an electrospray and a flame aerosol reactor. J. Aerosol Sci. 71, 52–64. doi:10.1016/j.jaerosci.2014.01.007
Xu, F., Sunderland, P. B., and Faeth, G. M. (1997). Soot formation in laminar premixed ethylene/air flames at atmospheric pressure. Combustion and Flame 108, 471–493. doi:10.1016/S0010-2180(96)00200-3
Yapp, E. K. Y., Chen, D., Akroyd, J., Mosbach, S., Kraft, M., Camacho, J., et al. (2015). Numerical simulation and parametric sensitivity study of particle size distributions in a burner-stabilised stagnation flame. Combustion and Flame 162, 2569–2581. doi:10.1016/j.combustflame.2015.03.006
Zhang, H.-B., You, X., Wang, H., and Law, C. K. (2014). Dimerization of Polycyclic Aromatic Hydrocarbons in Soot Nucleation. J. Phys. Chem. A. 118, 1287–1292. doi:10.1021/jp411806q
Keywords: soot, carbon, methane, particulate matter, particle size distibution, enriched air
Citation: Dasappa S and Camacho J (2021) Ultrafine Particulate Matter in Methane-Air Premixed Flames With Oxygen Enrichment. Front. Mech. Eng 7:739914. doi: 10.3389/fmech.2021.739914
Received: 12 July 2021; Accepted: 03 August 2021;
Published: 12 August 2021.
Edited by:
Chiara Saggese, United States Department of Energy (DOE), United StatesReviewed by:
Carson Chu, University of Toronto, CanadaGianluigi De Falco, DICMAPI - University of Naples Federico II, Italy
Francesco Carbone, University of Connecticut, United States
Copyright © 2021 Dasappa and Camacho. This is an open-access article distributed under the terms of the Creative Commons Attribution License (CC BY). The use, distribution or reproduction in other forums is permitted, provided the original author(s) and the copyright owner(s) are credited and that the original publication in this journal is cited, in accordance with accepted academic practice. No use, distribution or reproduction is permitted which does not comply with these terms.
*Correspondence: Joaquin Camacho, amNhbWFjaG9Ac2RzdS5lZHU=