- 1Department of Chemistry and Chemical Engineering, Malek-Ashtar University of Technology, Tehran, Iran
- 2Department of Cell and Molecular Biology, Faculty of Biological Sciences, Kharazmi University, Tehran, Iran
- 3Young Researchers and Elite Club, Roudehen Branch, Islamic Azad University, Roudehen, Iran
Here, a low-cost glucose/O2 Y-shaped microfluidic biofuel cell was developed using a printed circuit board for microelectrode construction. A double-side tape based on the pressure-sensitive adhesive was used for microchannel fabrication. A nanocomposite that consisted of reduced graphene oxide gold nanoparticles (AuNPs), and poly neutral red connected to enzymes was applied on the copper electrode surface. The Aspergillus niger glucose oxidase enzyme and Mytheliophthora thermophile laccase were used to prepare the modified anodic and cathodic electrodes. Different procedures such as cyclic voltammetry scanning electron microscope coupled with energy-dispersive x-ray spectroscopy (EDX), and atomic force microscopy were used to scan the modified electrodes. SEM/EDX microanalysis displayed the structural and morphological properties of the proposed nanocomposite. The biofuel cell performance demonstrated a maximum power density of 36 μW cm−2, an open-circuit voltage of 0.5 V, and a flow rate of 50 μl min−1. The proposed rapid technique with RGO/AuNPs/PNR bioelectrodes is a good approach for finding low-cost microfluidic biofuel cells.
Introduction
Nowadays, there is an increasing trend towards miniature biofuel cells over conventional fuel cells in portable devices due to their many benefits like being lightweight, relatively cheap rate, biocompatible with a number of biosystems, and able to operate under moderate temperature and pH conditions to produce green energy (Yang et al., 2018; Lee and Kjeang, 2010; Zebda et al., 2011b). The enzymatic microfluidic fuel cell is a type of miniature biofuel cell based on laminar flows in a microchannel that uses enzymes to generate electrical energy (Heller, 2004; Cooney et al., 2008; Beneyton et al., 2013). Microfluidic fuel cells have been developed to supply energy for portable electronics or implant devices (Zebda et al., 2011a; Aghahosseini et al., 2016; Brocato et al., 2012). In these biofuel cell devices, the soluble fuels and oxidants flow within the channels without a separator (Rewatkar et al., 2019b). Mostly, the enzyme-based biofuel cells are developed by different substrates as fuel e.g., glucose and oxidant, or O2. This is because glucose and O2 are widespread in living organisms so that glucose/O2 devices may be able to power bioelectronics systems in vivo (Rewatkar et al., 2019b; Kerzenmacher et al., 2011; Christwardana et al., 2016). In this way, biofuel cell miniaturization in the development of bioenergy devices for further biological applications has great potential (Wen et al., 2014; Campbell et al., 2015; Ortiz-Ortega et al., 2015). The lack of appropriate enzyme immobilization techniques and the incompatibility of enzymes with lithography processes cause limitations in research about the microfluidic systems. Therefore, future studies play an important role in discovering new uses (Rajasekhar et al., 2013).
In biofuel cells, glucose as a fuel is converted to gluconolactone using enzymes like glucose oxidase (GOx) through oxidation at the anode. Also, on a cathode electrode, oxygen (O2) is converted to water through reduction by different enzymes such as laccase (LAC) or bilirubin oxidase (BOx) (Zebda et al., 2009; Sané et al., 2013; Zhang et al., 2014). Moore et al. (2005), for the first time, have proposed a microfluidic enzymatic biofuel cell by NAD+-based alcohol dehydrogenase enzyme on bioanode and platinum cathode, soft lithography, and polydimethylsiloxane (PDMS) techniques (Moore et al., 2005). Another microfluidic system using photolithography and PDMS using vitamin K3-mediated glucose dehydrogenase (GDH) on bioanode and a Pt cathode was developed by Togo et al. (2007). In another report, Togo et al. (2008) replaced the Pt cathode of a biofuel cell with a BOx-adsorbed biocathode (Togo et al., 2008). A microfluidic biofuel cell consists of soft-lithography and PDMS techniques. A composite of azinobis (ethylbenzothiazoline-6-sulfonate) diammonium salt (ABTS) with LAC enzyme at the cathode and ABTS at the anode was created by Lim and Palmore (2007). Another microfluidic biofuel cell has been described using GDH immobilized on the anode and Box on the cathode (Togo et al., 2007; Zebda et al., 2009). Also, recent research has shown microfluidic enzymatic biofuel cells based on GOx/LAC enzymes coupled with nanomaterials like multi-walled carbon nanotube (MWCNT) or single-walled carbon nanotube (SWCNT). For example, González-Guerrero et al. (2013) made one microfluidic biofuel cell using rapid prototyping technique that consisted of a cathode by anthracene-modified MWCNT/LAC/Tetrabutylammonium bromide (TBAB) Nafion and a GOx/Ferrocenium-based polyethyleneimine polymer (Fc-C6-LPEI) bioanode (González-Guerrero et al., 2013). Also, a glucose microfluidic enzyme biofuel cell developed by Beneyton et al. (2013) used lithography and PDMS based on LAC and GOx covalently bound onto SWCNT electrodes. This research showed the maximum power density (1.65 mW cm−2 at 0.23 V) of an enzymatic fuel cell at the flow rate of 1000 μl h−1 (Beneyton et al., 2013). The former fabrication methods used for microfluidic biofuel cell technology are costly, time-consuming, and require complicated facilities and hefty skills. The search for new materials and procedures can help to improve the power output, action potential, and longevity of the microfluidic fuel cell.
In this paper, we described an enzyme immobilization coupled with a low-cost and rapid fabrication method related to previous work by our group (Mazar et al., 2020). As we explained before, the proposed method could easily be adapted by biological process and did not need the lithography process. In this method, the commercial IC layout software, Corel DRAW tool, and CO2 Laser cutter were used to prepare the device. PCB coupled with copper electrodes were used as a substrate. The Y-shaped microfluidic channel was prepared on a double-sided adhesive layer. The PET layer was applied to inhibit any air bubbles becoming trapped, and the entrances of the buffers to channels were created with PMMA block. O-rings accompanied the connection of PMMA entrance to the microfluidics system. A nanocomposite of reduced graphene oxide (RGO), gold nanoparticles (AuNPs), and poly neutral red (PNR) were used to modify the electrode surface. RGO-AuNPs hybrid structures offer remarkable advantages on physicochemical properties and functions of the biosensor and bio-imaging systems (Khalil et al., 2008). PNR as a conductive polymer showed advantages like simple fabrication, rapid response, and excellent potential for miniaturization of electrochemical devices based on the enzyme (Barsan et al., 2008; Broncová et al., 2008). To prepare a Y-shaped membraneless microfluidic biofuel cell based on an enzyme, modified coating copper (Cu) was used as the electrodes. Mytheliophthora thermophile LAC enzyme was used as the cathodic compartment of this enzymatic fuel cell. Also, the GOx enzyme from Aspergillus niger was used in the anodic chamber. The schematic mechanism of the enzymatic biofuel cell performance is described in Scheme 1. The proposed nanocomposition (RGO-AuNPs/PNR) was assayed using cyclic voltammetry (CV), a scanning electron microscopy accompanied with energy dispersive x-ray spectroscopy study (SEM-EDX), and atomic force microscopy (AFM) procedures. This work was developed to report a simple, inexpensive, and rapid microfabrication process by creating a Y-shaped enzymatic microfluidic biofuel cell based on new nanocomposite materials to harvest power from physiological fluids.
Experiment
Chemicals and Equipment
Glucose oxidase (GOx from Aspergillus niger), Gold nanoparticles (AuNPs), and Neutral red (NR) were prepared from Sigma-Aldrich (United States). Commercial Laccase (LAC from Mytheliophthora thermophile) was prepared from Novozymes Company (Portugal). D-(+)-glucose,
Electrode Characterization
The modified electrodes were characterized using a scanning electron microscope together with the EDX detector (SEM/EDX). Evaluation of the RGO-AuNPs composite morphology, and also RGO-AuNPs/PNR morphology characterization, was done with SEM. The elemental analysis of RGO-AuNPs was identified by the EDX. The AFM image was prepared using the tapping-mode of AFM. The samples were stored air-dry before AFM analysis.
Bioelectrode Preparation and Measurement
The bioelectrode preparation was done using a glassy carbon electrode (GCE) based on RGO-AuNPs/PNR nanocomposite, as described before (Mazar et al., 2017). The electrode surface was polished by alumina polishing powder a few times to prepare for modification. A composite of RGO-AuNPs (1:1.5) was deposited onto the GCE surface through drop-casting method, and NR was electropolymerized onto RGO-AuNPs modified electrode. The RGO was synthesized using the modified Hummers’ method (Marcano et al., 2010). A PNR film was polymerized on the RGO-AuNPs nanocomposite surface by cyclic voltammetry. PNR was made with 100 mM NR monomer solution in buffer pH 6, at 25°C for 20 cycles. The potential scanning was carried out between −1 V and +0.6 V at the scan rate of 50 mV s−1 (Schlereth and Karyakin, 1995). To prepare the bioanode, 4 µL of the 5 mg ml−1 GOx enzyme solution was drop cast on RGO-AuNPs/PNR. 4 µl of the 5 mg ml−1 LAC enzyme solution was pipetted onto RGO-AuNPs/PNR modified electrode for the biocathode preparation. All the electrochemical redox reactions of the anodic electrode were done in 100 mM phosphate buffer solution (PBS, pH 7) with 100 mM glucose. The acetate buffer solution (100 mM, pH 4.6) saturated by O2 was used for cathodic electrode experiments. The electrochemical properties of the experimental electrodes after modifications were characterized using a Potentiostat Galvanostat (Drop Sens model DRP-STAT 200) at room temperature with the glucose and O2 substrates. Also, the electrochemical measurements were obtained with cyclic voltammetry through the potential range of −0.9 to 0.0 V for bioanode, 0.2–1.1 V for biocathode, and the fixed potential scan rate = 100 mV s−1.
Microfluidic Biofuel Cell Device Design and Working Principles
The design of a microfluidic enzymatic biofuel cell device was according to the previous work of our group. It consists of five different sections (A) Cu electrodes on PCB as a substrate, (B) Double-sided fabric adhesive Tape, (C) Transparent polymer PET, (D) Two O-ring, and (E) PMMA block. The detail of the fabrication method is mentioned in reference (Mazar et al., 2020). Scheme 2 A and B shows the schematic and actual photograph of this microfluidic biofuel cell. The most fundamental principle concepts for the development of miniaturized microfluidic devices are the surface and interfacial tension, laminar flow, and capillary force. The Reynolds number (Re = νlρ·μ−1) predicts different fluid flow situations like turbulence or laminar. ν shows the fluid velocity (m s−1), l shows capillary diameter (m), ρ shows the fluid density (kg m−3), and μ shows the liquid viscosity (kg m−1 s). Re has different values based on the channel geometry, and low Re allows laminar fluid flow rather than turbulence. In microfluidic devices, two streams move in parallel together at laminar flow without turbulent mixing at the interface. Laminar flow causes fractioning of the samples like ions through diffusion between a stream and an adjacent stream (Kenis et al., 1999; Kenis et al., 2000; Rewatkar et al., 2019a).
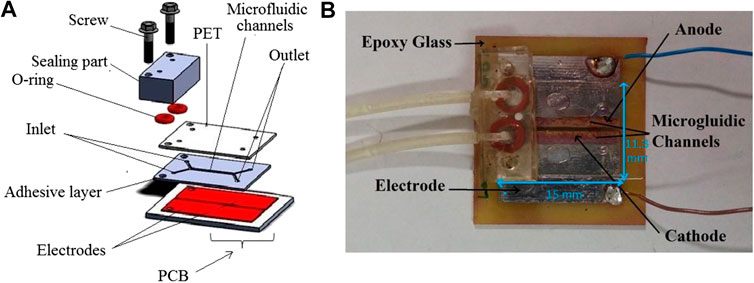
SCHEME 2. (A) Schematic illustration of the glucose biofuel cell segments. (B) Photo of the miniature biofuel cell (Mazar et al., 2020).
Microfluidic Biofuel Cell Assembly and Function
To assemble a microfluidic biofuel cell, the RGO-AuNPs/PNR/enzyme was coated on the Cu bare electrodes to perform the anode and cathode electrodes. The bioanode and biocathode decorations were prepared for consideration using drop 10 µL of GOx and LAC enzyme solutions containing 5 mg ml−1 onto RGO-AuNPs/PNR modified electrode, respectively. The whole body of the microfluidic biofuel cell is attached to the modified electrode surfaces strictly. The 100 mM glucose solved in 100 mM PBS with pH 7 was used as a fuel solution or anolyte of biofuel cell. Moreover, the oxidant solution or catholyte included an 100 mM air-saturated acetate buffer with pH 4.6. A syringe pump was used to distribute the flows of anolyte and catholyte to the microfluidic channel. The assembled biofuel cell performance, such as the current and power density, was tested by a multimeter using external resistance 10–500 kΩ. The electrolytes containing biofuels were loaded into the modified electrodes of the biofuel cell. The 1 × 15 mm2 surfaces of the Cu modified electrodes were in contact with the solutions. The streams of reactive species caused the oxidation-reduction reactions on both anodic and cathodic electrodes. The RGO-AuNPs/PNR/GOx bioanode oxidized the glucose substrate and released the electron. After catalytic oxidation of glucose on the bioanodic electrode, the electrons moved from the bioanode to the biocathode surface through external circuitry. The RGO-AuNPs/PNR/LAC cathode catalyzed oxygen reduction. Electrons and oxygen molecules were incorporated and then oxygen reduction occured. Three different samples of this biofuel cell were tested for the performance measurements of the device. Each experiment was repeated two times and had the same performance.
Results and Discussion
The Modified Electrode Characterization
The physical characterization of RGO-AuNPs as SEM image shows AuNPs with around a 30 nm diameter with most of them attached to the sheets of RGO (Figure 1A). Figure 1B indicates the SEM photomicrograph of the RGO-AuNPs/PNR. The elemental information of RGO-AuNPs composite was confirmed using the elemental analysis. Figure 1C displays the RGO-AuNPs nanocomposite characterization with EDX spectrum. The unique peaks of Au and oxygen related to RGO-AuNPs showing in the EDX spectrum confirmed that the nanocomposite consists of Au and oxygen. Figure 1D demonstrates the morphological and structural properties of the characterized RGO-AuNPs composite by AFM. It can be found that some AuNPs retain their spherical shapes and are attached to the RGO surface (Mazar et al., 2017).
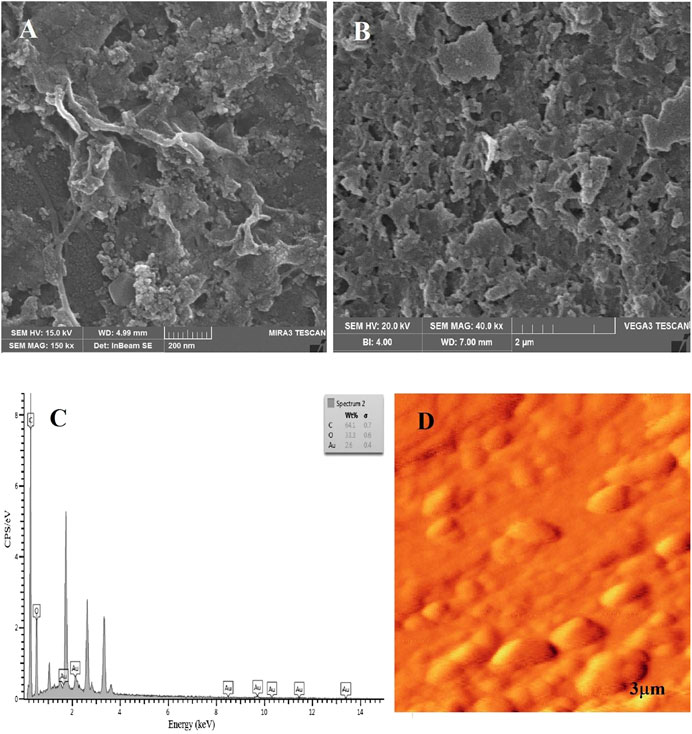
FIGURE 1. (A) SEM images of AuNPs-RGO. (B) SEM image of AuNPs-RGO/PNR composite. (C) Energy-dispersive X-ray microanalysis (EDX) confirming the Au and Oxygen elemental presence in the nanocomposite (D) AFM topography of gold nanoparticles by RGO (Mazar et al., 2017).
Electrochemical Properties of the Modified Bioelectrode Surface
Figure 2 describes two obtained cyclic voltammograms of the modified GC bioelectrodes. Figure 2A presents the RGO-AuNPs/PNR/LAC cyclic voltammograms of LAC cathode in the presence of oxygen. This voltammogram demonstrates a potential of around 0.67 V with a current of 0.33 μA. The O2 reduction potential of this biocathode is close to 0.65 V with a catalytic current of −0.22 μA. Figure 2B shows the cyclic voltammogram of modified bioanode prepared by RGO-AuNPs/PNR/GOx onto GCE surface in 100 Mm glucose presence. The reversible voltametric wave exhibits the catalytic activity of GOx at the potential of 0.37 V and a current of 4.6 μA. These data showed these anodic and cathodic electrodes should be appropriate for a biofuel cell.
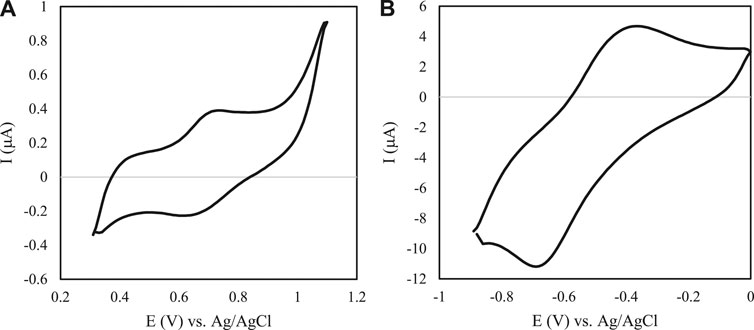
FIGURE 2. Cyclic voltammogram of modified GC electrodes. (A) Cyclic voltammogram of a LAC-modified RGO/AuNPs/PNR in 100 mM acetate buffer with pH 4.6 and O2 saturated. Scan rate: 100 mV s−1. (B) Cyclic voltammogram behaviors of a GOx-modified RGO/AuNPs/PNR in 100 mM PBS, pH 7 in the presence of 100 mM glucose. Scan rate: 100 mV s−1.
Evaluation of the Enzymatic Microfluidic Fuel Cell
After the characterization of the bioelectrodes, the cell performance was evaluated. The evaluation of the biofuel cell application was offered using the enzyme covalent binding in the microfluidic biofuel cell. Figure 3 describes the measured polarization curves and power outputs of the fuel cells with a buffer. The microfluidic biofuel cell consideration especially demonstrated an open circuit voltage of 0.5 V and a short circuit current of 420 μA cm−2 (Figure 3A). The delivered power density of the biofuel cell was around 36 μW cm−2 at a cell potential close to 0.2 V with 50 μl min−1 flow rate (Figure 3B). Table 1 compares different parameters of published glucose/O2 microfluidic biofuel cell papers.
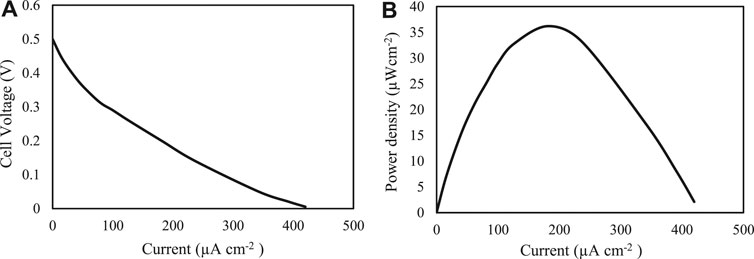
FIGURE 3. (A) Polarization curve and (B) power density curve obtained with a microfluidic biofuel cell consisting of a Cu electrode modified RGO-AuNPs/PNR using LAC/GOx at 25°C and 50 μl min−1 of cathodic: O2 saturated acetate buffer (100 mM; pH 4.6), and anodic: PBS (100 mM; pH 7) containing 100 mM glucose flows.
Proposed PCB/PSA Microfluidic Biofuel Cell Compare With the Traditional Micro-biofuel Cells
A microfluidics biofuel cell conventionally consists of microchannels over a thin metal layer of microelectrodes. A microelectrode is commonly fabricated using a thermal or plasma spray coating process and photolithographic patterning. In terms of the microchannel, the fabrication methods are usually based on the casting of PDMS (Lim and Palmore, 2007; Togo et al., 2007; Zebda et al., 2009; Beneyton et al., 2013; González-Guerrero et al., 2013; Khan et al., 2019; Torrinha et al., 2019). Even though these fabrication methods may have many benefits, they are costly, take a long time, and need interacting equipment and experienced operators. These difficulties confirm the disadvantage and inconvenience of the rapid prototyping processes or laboratories in limited-resource areas. The proposed biofuel cell involves a quick and economical manufacturing approach compared to other techniques. In this proposed approach, the Cu layer of PCB is applied as a microelectrode. The pressure-sensitive adhesive (PSA) tapes with a CO2 laser cutting machine are used for microchannel manufacture. The reusable part was used to join the different parts of the microfluidic system with the macro-world, without the need for the connectors as separators. In addition to these, this fabrication method can be comfortably adapted to biological modifications on the electrode surface.
Conclusions
Here, a rapid microfluidic glucose biofuel cell with inexpensive materials and fast methods for delivering electrical power is evaluated. Bioelectrodes are based on AuNPs/RGO/PNR/enzyme on a PCB as a microelectrode and a PSA tape as a structure material for Y-shaped microchannel. The anolyte and catholyte streams using a syringe pump flowed laminar at Cu modified electrode surfaces. The maximum power delivered from the device was 36 μW cm−2 at 0.2 V at the flow rate of 50 μL min−1 with 100 mM glucose with O2 saturated at room temperature. Finally, the presented microfluidic biofuel cell with one simple and inexpensive method can overcome many challenges in fuel cell technology, like low-cost and high-power-density systems. The used strategy process is only limited to lab testing and needs more amenable experiments until it can be used in mass production. Also, searching for new materials and enzymes should be a necessary matter to produce practical application powers.
Data Availability Statement
The raw data supporting the conclusion of this article will be made available by the authors, without undue reservation.
Author Contributions
All authors listed have made a substantial, direct, and intellectual contribution to the work and approved it for publication.
Conflict of Interest
The authors declare that the research was conducted in the absence of any commercial or financial relationships that could be construed as a potential conflict of interest.
References
Aghahosseini, H., Ramazani, A., Azimzadeh Asiabi, P., Gouranlou, F., Hosseini, F., Rezaei, A., et al. (2016). Glucose-based biofuel cells: nanotechnology as a vital science in biofuel cells performance. Nanochem. Res. 1, 42. doi:10.7508/ncr.2016.02.006
Barsan, M. M., Pinto, E. M., and Brett, C. M. A. (2008). Electrosynthesis and electrochemical characterisation of phenazine polymers for application in biosensors. Electrochim. Acta 53, 3973–3982. doi:10.1016/j.electacta.2007.10.012
Beneyton, T., Wijaya, I. P., Salem, C. B., Griffiths, A., and Taly, V. (2013). Membraneless glucose/O2 microfluidic biofuel cells using covalently bound enzymes. Chem. Commun. 49, 1094–1096. doi:10.1039/c2cc37906f
Brocato, S., Lau, C., and Atanassov, P. (2012). Mechanistic study of direct electron transfer in bilirubin oxidase. Electrochim. Acta 61, 44–49. doi:10.1016/j.electacta.2011.11.074
Broncová, G., Shishkanova, T. V., Krondak, M., Volf, R., and Král, V. (2008). Optimalization of poly(neutral red) coated-wire electrode for determination of citrate in soft drinks. Sensors 8, 594–606. doi:10.3390/s8020594
Campbell, A. S., Jeong, Y. J., Geier, S. M., Koepsel, R. R., Russell, A. J., and Islam, M. F. (2015). Membrane/mediator-free rechargeable enzymatic biofuel cell utilizing graphene/single-wall carbon nanotube cogel electrodes. ACS Appl. Mater. Inter. 7, 4056–4065. doi:10.1021/am507801x
Christwardana, M., Kim, K. J., and Kwon, Y. (2016). Fabrication of mediatorless/membraneless glucose/oxygen based biofuel cell using biocatalysts including glucose oxidase and laccase enzymes. Sci. Rep. 6, 30128. doi:10.1038/srep30128
Cooney, M. J., Svoboda, V., Lau, C., Martin, G., and Minteer, S. D. (2008). Enzyme catalysed biofuel cells. Energy Environ. Sci. 1, 320–337. doi:10.1039/B809009B
Escalona-Villalpando, R. A., Dector, A., Dector, D., Moreno-Zuria, A., Durón-Torres, S. M., Galván-Valencia, M., et al. (2016). Glucose microfluidic fuel cell using air as oxidant. Int. J. Hydrogen Energ. 41, 23394–23400. doi:10.1016/j.ijhydene.2016.04.238
Galindo, R., Dector, A., Arriaga, L. G., Gutiérrez, S., and Herrasti, P. (2012). Maghemite as a catalyst for glucose oxidation in a microfluidic fuel cell. J. Electroanal. Chem. 671, 38–43. doi:10.1016/j.jelechem.2012.02.020
González-Guerrero, M. J., Esquivel, J. P., Sánchez-Molas, D., Godignon, P., Muñoz, F. X., del Campo, F. J., et al. (2013). Membraneless glucose/O2 microfluidic enzymatic biofuel cell using pyrolyzed photoresist film electrodes. Lab. Chip 13, 2972–2979. doi:10.1039/c3lc50319d
Heller, A. (2004). Miniature biofuel cells. Phys. Chem. Chem. Phys. 6, 209–216. doi:10.1039/b313149a
Jayapiriya, U., Rewatkar, P., and Goel, S. (2021). Miniaturized polymeric enzymatic biofuel cell with integrated microfluidic device and enhanced laser ablated bioelectrodes. Int. J. Hydrogen Energ. 46, 3183–3192. doi:10.1016/j.ijhydene.2020.06.133
Kenis, P. J., Ismagilov, R. F., Takayama, S., Whitesides, G. M., Li, S., and White, H. S. (2000). Fabrication inside microchannels using fluid flow. Acc. Chem. Res. 33, 841–847. doi:10.1021/ar000062u
Kenis, P. J., Ismagilov, R. F., and Whitesides, G. M. (1999). Microfabrication inside capillaries using multiphase laminar flow patterning. Science 285, 83–85. doi:10.1126/science.285.5424.83
Kerzenmacher, S., Kräling, U., Metz, T., Zengerle, R., and Von Stetten, F. (2011). A potentially implantable glucose fuel cell with Raney-platinum film electrodes for improved hydrolytic and oxidative stability. J. Power Sourc. 196, 1264–1272. doi:10.1016/j.jpowsour.2010.08.019
Khalil, I., Julkapli, N. M., Yehye, W. A., Basirun, W. J., and Bhargava, S. K. (2008). Graphene-gold nanoparticles hybrid-synthesis, functionalization, and application in a electrochemical and surface-enhanced raman scattering biosensor. Materials 9, 406. doi:10.3390/ma9060406
Khan, H., Kim, C. M., Kim, S. Y., Goel, S., Dwivedi, P. K., Sharma, A., et al. (2019). Fabrication of enzymatic biofuel cell with electrodes on both sides of microfluidic channel. Int. J. Precis. Eng. Manuf. Green Technol. 6, 511–520. doi:10.1007/s40684-019-00056-x
Lee, J. W., and Kjeang, E. (2010). A perspective on microfluidic biofuel cells. Biomicrofluidics 4, 41301. doi:10.1063/1.3515523
Lim, K. G., and Palmore, G. T. (2007). Microfluidic biofuel cells: the influence of electrode diffusion layer on performance. Biosens. Bioelectron. 22, 941–947. doi:10.1016/j.bios.2006.04.019
Marcano, D. C., Kosynkin, D. V., Berlin, J. M., Sinitskii, A., Sun, Z., Slesarev, A., et al. (2010). Improved synthesis of graphene oxide. ACS Nano 4, 4806–4814. doi:10.1021/nn1006368
Mazar, F. M., Alijanianzadeh, M., Molaeirad, A., and Heydari, P. (2017). Development of novel glucose oxidase immobilization on graphene/gold nanoparticles/poly neutral red modified electrode. Process. Biochem. 56, 71–80. doi:10.1016/j.procbio.2017.02.008
Mazar, F. M., Alijanianzadeh, M., Rad, A. M., and Heydari, P. (2020). Power harvesting from physiological serum in microfluidic enzymatic biofuel cell. Microelectron. Eng. 219, 111159. doi:10.1016/j.mee.2019.111159
Moore, C. M., Minteer, S. D., and Martin, R. S. (2005). Microchip-based ethanol/oxygen biofuel cell. Lab. Chip 5, 218–225. doi:10.1039/b412719f
Ortiz-Ortega, E., Gurrola, M., Arriaga, L., and Ledesma-García, J. (2015). A bendable and compactdevice for low-power application. J. Phy. Conf. Ser. 660, 012054. doi:10.1088/1742-6596/660/1/012054
Rajasekhar, A., Gimi, B., and Hu, W. (2013). Applications of semiconductor fabrication methods to nanomedicine: a review of recent inventions and techniques. Recent Pat Nanomed 3, 9–20. doi:10.2174/1877912311303010003
Rewatkar, P., and Goel, S. (2018). Paper-based membraneless Co-laminar microfluidic glucose biofuel cell with MWCNT-fed bucky paper bioelectrodes. IEEE Trans. Nanobioscience 17, 374–379. doi:10.1109/TNB.2018.2857406
Rewatkar, P., Hitaishi, V. P., Lojou, E., and Goel, S. (2019a). Enzymatic fuel cells in a microfluidic environment: status and opportunities. a mini review. Electrochem. Commun. 107, 106533. doi:10.1016/j.elecom.2019.106533
Rewatkar, P., Kothuru, A., and Goel, S. (2019b). “Laser-induced Flexible Graphene Bioelectrodes for Enzymatic Biofuel Cell,” in IEEE 13th international conference on nano/molecular medicine & engineering (NANOMED), November, 2019, IEEE, Gwangju, Korea (South), 30–34.
Rewatkar, P., Kothuru, A., and Goel, S. (2020). PDMS-based microfluidic glucose biofuel cell integrated with optimized laser-induced flexible graphene bioelectrodes. IEEE Trans. Electron. Devices 67, 1832–1838. doi:10.1109/ted.2020.2971480
Sané, S., Jolivalt, C., Mittler, G., Nielsen, P. J., Rubenwolf, S., Zengerle, R., et al. (2013). Overcoming bottlenecks of enzymatic biofuel cell cathodes: crude fungal culture supernatant can help to extend lifetime and reduce cost. ChemSusChem 6, 1209–1215. doi:10.1002/cssc.201300205
Schlereth, D. D., and Karyakin, A. A. (1995). Electropolymerization of phenothiazine, phenoxazine and phenazine derivatives: characterization of the polymers by UV-visible difference spectroelectrochemistry and Fourier transform IR spectroscopy. J. Electroanal. Chem. 395, 221–232. doi:10.1016/0022-0728(95)04127-a
Togo, M., Takamura, A., Asai, T., Kaji, H., and Nishizawa, M. (2007). An enzyme-based microfluidic biofuel cell using vitamin K3-mediated glucose oxidation. Electrochim. Acta 52, 4669–4674. doi:10.1016/j.electacta.2007.01.067
Togo, M., Takamura, A., Asai, T., Kaji, H., and Nishizawa, M. (2008). Structural studies of enzyme-based microfluidic biofuel cells. J. Power Source 178, 53–58. doi:10.1016/j.jpowsour.2007.12.052
Torrinha, Á., Montenegro, M. C. B. S. M., and Araújo, A. N. (2019). Conjugation of glucose oxidase and bilirubin oxidase bioelectrodes as biofuel cell in a finger-powered microfluidic platform. Electrochim. Acta 318, 922–930. doi:10.1016/j.electacta.2019.06.140
Wen, D., Liu, W., Herrmann, A. K., and Eychmüller, A. (2014). A membraneless glucose/O(2) biofuel cell based on Pd aerogels. Chemistry 20, 4380–4385. doi:10.1002/chem.201304635
Yang, Y., Liu, T., Tao, K., and Chang, H. (2018). Generating electricity on chips: microfluidic biofuel cells in perspective. Ind. Eng. Chem. Res. 57, 2746–2758. doi:10.1021/acs.iecr.8b00037
Zebda, A., Gondran, C., Le Goff, A., Holzinger, M., Cinquin, P., and Cosnier, S. (2011a). Mediatorless high-power glucose biofuel cells based on compressed carbon nanotube-enzyme electrodes. Nat. Commun. 2, 370. doi:10.1038/ncomms1365
Zebda, A., Innocent, C., Renaud, L., Cretin, M., Pichot, F., Ferrigno, R., and Tingry, S. (2011b). “Enzyme-based microfluidic biofuel cell to generate micropower,” in Biofuel's engineering process technology, Rijeka, Croatia: IntechOpen.
Zebda, A., Renaud, L., Cretin, M., Innocent, C., Pichot, F., Ferrigno, R., et al. (2009). Electrochemical performance of a glucose/oxygen microfluidic biofuel cell. J. Power Sourc. 193, 602–606. doi:10.1016/j.jpowsour.2009.04.066
Keywords: enzyme-based biofuel cell, glucose oxidase, laccase, microfluidics, RGO/AuNPs/PNR
Citation: Mashayekhi Mazar F, Alijanianzadeh M, Molaei Rad A and Heydari P (2021) Microfluidic Rapid Prototype Enzymatic Biofuel Cell Based on a Nanocomposite. Front. Mech. Eng 7:599414. doi: 10.3389/fmech.2021.599414
Received: 27 August 2020; Accepted: 08 February 2021;
Published: 07 April 2021.
Edited by:
Hao Ren, ShanghaiTech University, ChinaReviewed by:
Mariana Amorim Fraga, Federal University of São Paulo, BrazilMarwan Nafea, University of Nottingham Malaysia Campus, Malaysia
Copyright © 2021 Mashayekhi Mazar, Alijanianzadeh, Molaei Rad and Heydari. This is an open-access article distributed under the terms of the Creative Commons Attribution License (CC BY). The use, distribution or reproduction in other forums is permitted, provided the original author(s) and the copyright owner(s) are credited and that the original publication in this journal is cited, in accordance with accepted academic practice. No use, distribution or reproduction is permitted which does not comply with these terms.
*Correspondence: Mahdi Alijanianzadeh, alijanianzadeh_m@yahoo.com