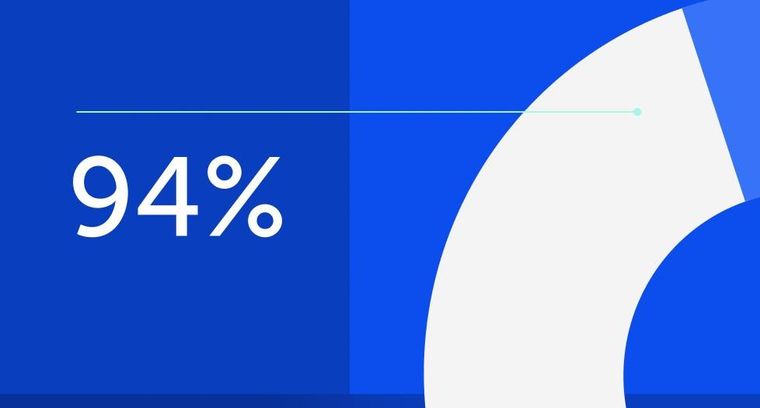
94% of researchers rate our articles as excellent or good
Learn more about the work of our research integrity team to safeguard the quality of each article we publish.
Find out more
MINI REVIEW article
Front. Mech. Eng., 05 May 2021
Sec. Thermal and Mass Transport
Volume 7 - 2021 | https://doi.org/10.3389/fmech.2021.505923
This article is part of the Research TopicMILD Combustion: Modelling Challenges, Experimental Configurations and Diagnostic ToolsView all 14 articles
MILD combustion has a wide potential in enhancing thermal efficiency with nearly zero emissions. It has no visible flame since the radiation from the reacting zones is attenuated due to both the intermediate species at reduced temperatures, induced by intensely burned gas recirculation, and the absence of particulate emitters. Beyond these main features, there are other characteristics such as temperature uniformity and distributed ignition that have to be addressed and analyzed looking at the peculiar role of the heat transfer for such reactors. First, the category of combustion systems object of the study is described. Afterwards an analysis on the heat transfer mechanisms under MILD combustion of gaseous fuels is carried out. Therefore, in this Mini-Review, several literature findings highlighting the role of the heat transfer on the combustion peculiarities of MILD reactors (i.e., temperature uniformity, distributed ignition, low pollutant emissions) are reported and discussed. Heat exchange modes, in fact, contribute to providing MILD macroscopic characteristics by means of the strong interplay between wall and gas heat transfer, instead of the reactive structure. In particular, the thermal behavior of these systems is analyzed in order to stress the distinctive role of the heat loss and the relative contributions of the convective and radiative terms. Heat transfer mechanisms between gas and walls and their interactions, in fact, favor the wide temperature distribution within the chamber. In order to better understand the different effects of the heat transfer under MILD regime, the mechanisms regarding walls and recirculating gas are separately investigated.
Moderate or Intense Low-oxygen Dilution (MILD) Combustion (Cavaliere and de Joannon, 2004) is characterized by low-oxygen concentrations and high inlet temperatures. Important characteristics of this oxidation process are homogeneous temperatures, distributed ignition (Khalil and Gupta, 2017), absence of visible flames (Karyeyen et al., 2019) and low CO, NOx and soot emissions (de Joannon et al., 2012). It is a very good candidate for low-calorific-value (Huang et al., 2014; Sabia et al., 2019), hydrogen, nitrogen-based (Sabia et al., 2019) fuels and industrial ones (Parente et al., 2008). The process occurs under conditions different from conventional ones because of the strong interplay between mixing, chemistry and heat transfer (Lamouroux et al., 2014; Chen et al., 2018).
Several facilities such as the Jet-in-Hot-Coflows (Medwell et al., 2008; Oldenhof et al., 2010), and the Cabra flame (Cabra et al., 2005), are used to reproduce highly diluted and preheated conditions, on the basis of kinetic time-scales modifications. In all these cases the combustion process is unconfined and occurs far from the walls, under adiabatic conditions (Kim et al., 2005; De and Dongre, 2015).
MILD is realized by using exhaust gas recirculation (Cavigiolo et al., 2003; Minamoto et al., 2013). The internal EGR is obtained through proper chamber designs (Veríssimo et al., 2013), by realizing long residence times (Li et al., 2014) due to convoluted and confined flow-fields (Sorrentino et al., 2018). Gas entrainment (de Joannon et al., 2017) lead to chemical time-scales comparable to mixing ones (Özdemir and Peters, 2001) and reflect the importance of impinging wall-jets (de Joannon et al., 2017) or cavity flows (Chinnici et al., 2017).
These systems, identified hereafter as “MILD reactors,” are classified with respect to nominal MILD requisites. Specifically, the inlet-feeding temperature (Tin), should be higher than the spontaneous-ignition one (Tign), and the differential temperature increase between products and reactants (ΔTproduct), should be lower than Tign. In the current literature, three main configurations of MILD reactors can be identified with respect to the strategy they use to fulfill the above-mentioned conditions (Perpignan et al., 2018). In the following, a review of these configurations is given.
The first configuration regards reactors where Tin >Tign and ΔTproduct <Tign are satisfied through external feeding. This is the case of post-combustion systems. Fuel-rich (Giménez-López et al., 2011) or fuel-lean re-burning processes (Miller et al., 1998; Kim et al., 2012) are examples of in-furnace and/or post-furnace NOx abatement systems. Fuel is injected in exhaust/oxygen mixtures for jet-engine systems (Fureby, 2000) or waste incineration (Parr et al., 1996).
Another group of MILD reactors refers to the case Tin >Tign, obtained by external feeding while ΔTproduct <Tign, through intra-reactor phenomena. Such a condition is typically obtained in recuperative/regenerative furnace systems (Wünning and Wünning, 1997; Katsuki and Hasegawa, 1998; Özdemir and Peters, 2001; Rafidi and Blasiak, 2006; Li et al., 2014) in which part of the combustion heat is subtracted by walls through exhausts reverse flow (Nemitallah et al., 2018).
The third MILD reactors category involves those systems where both requirements are fulfilled by internal recirculation and heat exchange strategies. Also, in this case, many different fluid-dynamic arrangements can be used. In particular, parallel jets (Abtahizadeh et al., 2012; Huang et al., 2014; Cheong et al., 2019) and cyclonic flow configurations (de Joannon et al., 2017). The former has also been used for burners in regenerative conditions (Wünning and Wünning, 1997; Katsuki and Hasegawa, 1998; Rafidi and Blasiak, 2006; Li et al., 2014). Regarding the cyclonic systems, LUCY burner (Sorrentino et al., 2016) is a valuable example of MILD reactor with a tailored flow-field (Sorrentino et al., 2017).
In the second and third categories, the condition ΔTproduct <Tign, is influenced by heat exchange at walls, and the residence time poses severe design restrictions (Kruse et al., 2015). Indeed, heat loss plays a key role in reducing the reacting mixture temperature (Szegö et al., 2009). On the other hand, a sufficiently high temperature must be guaranteed inside the reactor itself. In other words, the walls are responsible for both the heat loss by products and the heat gain by reactants (Danon et al., 2011).
Energy equation includes contributions of the three heat transfer modes: conduction, convection and thermal radiation, where the latter is included through the radiative flux divergence (Özışık, 1973; Viskanta, 2005; Dombrovsky and Baillis, 2010; Modest, 2013). In the following sections, the contributions of each heat transfer mechanism under MILD conditions are analyzed and discussed.
The overall heat transfer is conceptually divided into three phenomena: gas-to-gas, gas-to-wall and wall-to-wall.
EGR is essential in MILD to mix fresh reactants with exhausts in order to lower the reaction-rate and sustain the oxidation for diluted conditions (Tu et al., 2015). Thus, the reactor design (Liu et al., 2015) must ensure the required convection and radiation levels. High concentrations of absorbing and emitting H2O/CO2 mixtures inside the combustion chamber (Dorigon et al., 2013) lead to enhanced radiative re-absorptions. This effect is not negligible and contributes to the system thermal homogeneity, as highlighted in several literature papers (Zhang et al., 2019; Ceriello et al., 2020).
The specific aerodynamic requirements of MILD Combustion (enhanced jet mixing through high inlet jets momentum and large scale recirculation of flue gases) influence the contribution of convective heat transfer mode. In particular the share of convective heat transfer in the MILD mode is enhanced by the higher velocity and momentum of air at the inlet when compared to the conventional combustion mode (Riahi et al., 2013). On the other hand the increased internal recirculation enhances the convective heat transfer process and this effect is more marked in MILD oxyfuel combustion processes (Chen et al., 2012).
In Figure 1, emissivity of CO2 and H2O as functions of the temperature at different products between total pressure and length of the enclosure (Hottel and Cohen, 1958), and modified by Alberti et al. (2015), are reported. Those trends show that, for both species, when the temperature of the mixture gets lower, the emissivity increases. For MILD systems, operating in the range 1,000< T <1,400 K, the standard gas emissivity of burned products results higher with respect to conventional combustion (where T >1,800 K). This is due to both the effect of lower system temperatures and CO2/H2O higher partial pressures. Therefore, the effect of the re-absorption is noticeable in MILD, even for a small-scale apparatus (Sorrentino et al., 2018; Zhang et al., 2019).
Figure 1. Total emissivity of pure carbon dioxide (left) and pure water vapor (right) as a function of temperature (Hottel and Cohen, 1958) and modified by Alberti et al. (2015).
To model radiative re-absorption, the spectral dependence of the radiative properties of the exhausts must be known. The most accurate method to calculate the radiative properties of the combustion products is the line-by-line integration (Chu et al., 2011; Zeng et al., 2020) which uses spectroscopic databases (Rothman et al., 2010; Gordon et al., 2017; Pannier and Laux, 2019). However, LBL calculation is not feasible for the CFD of complex combustion systems. Very few works showed LBL with Monte Carlo methods coupled to CFD for combustion applications (Zhao et al., 2013; Ren et al., 2018).
Intermediate between LBL and global models, narrow band models such as the correlated-k approach has also been retained in some works by solving the radiative transfer equation in the CFD using a Monte-Carlo based on the emission-reciprocity method (ERM) (Koren et al., 2018; Rodrigues et al., 2019).
Global models such as the full spectrum correlated-K method (FSK) and the spectral line based weighted sum of gray gas method (SLW) have received a lot of attention because of their accuracy and low computational costs. Despite that, their implementation is not straightforward since the correlation of FSK and SLW are usually offered for single gas species.
A simple solution that is commonly used in commercial CFD codes, due to its computational efficiency, relies on the resolution of only one radiative transfer equation to obtain the total radiation intensity field by replacing the spectral absorption coefficient with the mean gray absorption coefficient (Galletti et al., 2007; Pallarés et al., 2007; Yang et al., 2007). In such cases simplified radiation models optimized for combustion systems can be developed by using the detailed information given by the spectral databases (Paul et al., 2019).
Usually, in numerical modeling of MILD/Flameless systems the Weighted-Sum-of-Gray-Gases Model, WSGGM (Modest, 1991), is adopted to model the spectrally dependent properties of the combustion gases, making use of updated spectral databases. A possible choice is to use the coefficient proposed by Smith et al. (1982). Modified WSGG (Bordbar et al., 2014; Cassol et al., 2014) were recently developed. In particular, the coefficients proposed in this models were used to account for various temperature and different concentrations of H2O/CO2 in each computational cell. According to this radiative database, both the absorption coefficients and the black body weights of the gray gases depend on molar ratio, while only the weights depend on temperature. Modified WSGG models were applied for simulating MILD condition in several works (Galletti et al., 2007; Sorrentino et al., 2018).
An interesting modification of the WSGG model is represented by the SLW (Webb et al., 2018) where detailed gas spectroscopic data are captured by a designed distribution function (Denison and Webb, 1993). It gives some improvements with respect to WSGG (Krishnamoorthy et al., 2010; Webb et al., 2018). However, since the MILD does not have strong gradients of temperature and concentration, the improvements of SLW with respect to WSGG do not justify the increase of computational costs. Therefore, the flameless nature of the MILD process supports the use of a modified WSGG to obtain reliable results for such systems.
Regarding the solution method of the radiative-transfer-equation (RTE), considering the intermediate optical thickness of MILD, the Discrete Ordinate (DO) radiation model (Chui and Raithby, 1993) seems an appropriate choice. DO approximation converts the RTE into a series of differential equations along directions (Modest, 2013).
On the other hand, some studies showed that P1 model gave worse prediction than DO for MILD (Frassoldati et al., 2010). Moreover very few works used Monte Carlo methods in MILD burners and results differed by <5% with respect to DO, but the latter model was preferred because of its lower computational cost (Galletti et al., 2007).
Some literature works reported the relative importance of convective and radiative modes in MILD combustion. In particular, heat transfer characteristics from the furnace walls for H2O/CO2 mixtures (Zhang et al., 2019) were analyzed and they showed that the H2O increase enhanced the share of heat radiation. When H2O is substitute for CO2, the influence of heat capacity progressively declines and the effect of heat capacity on strengthening convective heat transfer or weakening radiative heat transfer gradually decreases. Moreover, thermal performance analysis of hybrid solar receiver MILD combustor showed that the ratio of radiative to convective heat transfer rate is found to be dependent on the fuel type (Chinnici et al., 2019).
In this context, engineering global assessments on the importance of radiative heat transfer with respect to convective one can be obtained for MILD systems without solving RTE if average values of fluid and wall temperatures are available and by adopting several approximations.
In a combustion system, the overall heat transfer flux between gas and walls, Q, in W/m2, can be expressed as the sum of a convective, Qc, and a radiative term, Qr (Baukal, 2000), as follows:
with
In such relations, is the average convection heat transfer coefficient over the heat transfer area, in W/m2 K, Tw the wall surface temperature, Tf the fluid temperature, Tg and εg are the exhaust (H2O/CO2 mixture) mean temperature and emissivity, respectively (Kreith et al., 2011). In the expression of the radiative heat flux, Qr, the view factors are considered to have value 1. This assumption for the flux leaving gas and reaching walls is supported by the black body cavity behavior of MILD systems. Moreover, since the combustion process extends to the whole volume under MILD conditions, the view factor can be assumed to be 1 as well.
In the expression of Equation (3), both the black walls and gas are assumed to emits and absorbs heat at all wavelengths. A more accurate expression can be found in the book by Lefebvre and Ballal (2010) where the gas emits only a few narrow bands of wavelengths and absorbs only those wavelengths included in its emission bands.
For a MILD reactor, as reported in several works (Chinnici et al., 2017; Sorrentino et al., 2018; Zhang et al., 2019), the fluid temperature, Tf, can be approximated to the mean gas temperature inside the combustion chamber, Tg, because of the absence of steep gradients and peaks:
This approximation is commonly adopted for industrial furnaces and denoted as Well-Stirred model (Hewitt et al., 1994). Therefore, the ratio between radiative and convective term reads
In Figure 2 this dimensionless parameter is showed for both CO2 and H2O as a function of the gas temperature, Tg, at different wall temperatures, Tw (solid black lines). The values of the gas emissivity as a function of their temperature, εg(Tg), were read from Hottel's diagrams (Figure 1).
Figure 2. Trends of the ratio between radiation and convection for CO2 (left) and H2O (right) as a function of the mean gas temperature and at different wall temperatures, for a small-scale cyclonic reactor (Sorrentino et al., 2018).
In order to approximate the MILD operating conditions of the cyclonic burner LUCY, reported in Sorrentino et al. (2018), the product of pressure and length and the convective coefficient hf were set to 10 bar cm and 35 , respectively.
According to Sorrentino et al. (2018), MILD conditions are located in the red regions, where the radiative heat transfer between gases and walls is around 1.5 times the convective one, for both the gases (Figure 2). This outcome supports one of the main results of the referred work: radiative heat transfer modeling is essential in a cyclonic MILD burner for computations (Sorrentino et al., 2018).
The heat transfer analysis was here reported for a specific configuration (cyclonic burner with hf = 35 W/m2/K) for illustrative purposes. In such a case both the presence of cavity flows in the chamber and wall jets near the inlet region influenced the wall convective heat transfer. Clearly, such analysis can be replicated for different MILD/Flameless systems on the basis of the aerodynamics characteristics of the system. It is here worthwhile to note that the design choices for MILD systems related to flow pattern features and/or inlet jets configurations strongly influence the convective heat transfer rate.
Moreover, in Figure 2, the role of the temperature of the walls appears to be fundamental to move from a convective-based to a radiative-based heat transfer mode. The latter favors the stabilization of MILD conditions, as well proved by Chinnici et al. (2017) though a proper control of the heat flux distribution at walls.
The analysis carried out for the cyclonic burner in Figure 2 could be easily replicated for different MILD/Flameless systems with a large amount of internally recirculating combustion products (Rafidi and Blasiak, 2006; Noor et al., 2013; Huang et al., 2017; Zhang et al., 2019).
In black enclosures, radiative transfer depends on view factors and surface temperatures. For diffuse-gray enclosures, thermal radiation is also a function of the surface's emissivity (Modest, 2013). Considering gray and nearly opaque surfaces, part of the “gas-to-walls” radiative heat transfer can be seen as a “gas-to wall-to gas” contribution, which means that a portion of the radiation emitted by the mixture to the walls is reflected and absorbed from the gas itself, result in making the thermal field more uniform. This effect is enhanced by the “wall-to-wall” contribution of the apparatus and, therefore, by the tendency of the system to behave as a blackbody cavity.
In order to express this characteristic, the Gebhart factor (Gebhart, 1961) can be used. In fact, the radiative heat exchanged in an enclosure composed of surfaces with piecewise constant temperature and emissivity can be calculated in terms of Gebhart factors (Gebhart, 1961; Dahlquist and Björck, 2008), Gij, which are defined as a fraction of energy leaving surface i which reaches surface j and is absorbed. The Gebhart factors Gij equals the view factors, Fij, for black surface. The net radiative heat exchange between any surface k and all the others N surfaces of the enclosure can be expressed in terms of the Gebhart factors as
where Aj, εj, θj, Ak, εk and θk are area, emissivity, and temperature of surface j and area, emissivity, and temperature of surface k, respectively (Atherton et al., 1987). The proposed wall-to-wall heat transfer treatment is based on the assumption of temperature uniformity at the walls and transparent medium between the walls. This peculiar feature is well-reported for MILD reactors (Chinnici et al., 2017; Sorrentino et al., 2018) and it is emphasized for high values of the Surface/Volume ratio where the participating medium is distributed to the whole reactor volume.
MILD reactors with confinement, exhibit Gebhart factors (related to the outlet section) that are usually lower than 0.1 (Chinnici et al., 2017; Sorrentino et al., 2018; Zhang et al., 2019), when evaluated on the basis of reactor information.
MILD combustion is usually employed in furnaces or boilers where inner walls are built with refractory materials with emissivity that is usually higher than 0.7.
Therefore, these systems tend to behave as a cavity maximizing the “gas-to-wall-to-gas re-absorption.” This mechanism ensures uniform walls temperature inside the chamber. On the other hand, the radiation that cannot leave the system contributes to wall temperatures homogeneity with higher radiation-to-convection ratio, as reported in Figure 2.
The mini-review highlighted the role of the heat transfer for systems where MILD combustion is achieved by means of EGR. The investigation of the different transport mechanisms pointed out the key role of heat exchange mechanisms in realizing the performances and features of the MILD regime.
The “gas to gas” heat transfer mechanism is crucial both for convection and radiation. The convective term is due to the high velocities, required to ensure elevated EGR, and the confinement of the flows by the walls. Moreover, the recirculating combustion products (H2O/CO2 mixture) are involved in the radiative re-absorption.
Appropriate models are needed for gases radiative properties. Among them, WSGG model based on radiative databases created ad-hoc for mixtures of combustion products at high temperatures is a reasoning solution.
Regarding the “gas to wall” interaction, a quantitative analysis on a MILD reactor (Sorrentino et al., 2018) was carried out and it was demonstrated that the radiation is the dominant heat transfer mechanism between reactive mixture and walls. Such a result is known for traditional large-scale furnaces, but it represents an interesting finding for a small-scale Flameless system.
Finally, the “wall to wall” heat exchange was analyzed, pointing out that MILD systems behave as black body cavity due to their confined enclosures. Therefore, the walls at high temperature strongly exchange heat by irradiation contributing to the system homogeneity.
Convective and radiative heat transfer modes have remarkable effects also on design and simulation of MILD systems, especially concerning the temperature uniformity.
GS and GC were involved in the bibliographic research to include in the manuscript the most important literature contributions on the effect of heat transfer on MILD systems. They have also strongly contributed in writing several parts of the article. AC and MdJ gave their support in the theoretical background regarding identification and classification of MILD Combustion processes. RR gave important insights and hints in the physical description of convective and radiative heat transfer phenomena involved in combustion technologies. He also strongly revised the English language in the manuscript. All authors contributed to the article and approved the submitted version.
The authors declare that the research was conducted in the absence of any commercial or financial relationships that could be construed as a potential conflict of interest.
Abtahizadeh, E., van Oijen, J., and De Goey, P. (2012). Numerical study of mild combustion with entrainment of burned gas into oxidizer and/or fuel streams. Combust. Flame 159, 2155–2165. doi: 10.1016/j.combustflame.2012.02.004
Alberti, M., Weber, R., and Mancini, M. (2015). Re-creating Hottel's emissivity charts for carbon dioxide and extending them to 40 bar pressure using HITEMP-2010 data base. Combust. Flame 162, 597–612. doi: 10.1016/j.combustflame.2014.09.005
Atherton, L. J., Derby, J. J., and Brown, R. A. (1987). Radiative heat exchange in Czochralski crystal growth. J. Cryst. Growth 84, 57–78. doi: 10.1016/0022-0248(87)90114-X
Bordbar, M. H., Wecel, G., and Hyppänen, T. (2014). A line by line based weighted sum of gray gases model for inhomogeneous CO2-H2O mixture in oxy-fired combustion. Comb. Flame 161, 2435–2445. doi: 10.1016/j.combustflame.2014.03.013
Cabra, R., Chen, J. Y., Dibble, R. W., Karpetis, A. N., and Barlow, R. S. (2005). Lifted methane-air jet flames in a vitiated coflow. Combust. Flame 143, 491–506. doi: 10.1016/j.combustflame.2005.08.019
Cassol, F., Brittes, R., França, F. H., and Ezekoye, O. A. (2014). Application of the weighted-sum-of-gray-gases model for media composed of arbitrary concentrations of H2O, CO2 and soot. Int. J. Heat Mass Transf. 79, 796–806. doi: 10.1016/j.ijheatmasstransfer.2014.08.032
Cavaliere, A., and de Joannon, M. (2004). Mild combustion. Progr. Energ. Comb. Sci. 30, 329–366. doi: 10.1016/j.pecs.2004.02.003
Cavigiolo, A., Galbiati, M. A., Effuggi, A., Gelosa, D., and Rota, R. (2003). Mild combustion in a laboratory-scale apparatus. Combust. Sci. Technol. 175, 1347–1367. doi: 10.1080/00102200302356
Ceriello, G., Sorrentino, G., Cavaliere, A., Sabia, P., de Joannon, M., and Ragucci, R. (2020). The role of dilution level and canonical configuration in the modeling of MILD combustion systems with internal recirculation. Fuel 264:116840. doi: 10.1016/j.fuel.2019.116840
Chen, L., Yong, S. Z., and Ghoniem, A. F. (2012). Oxy-fuel combustion of pulverized coal: characterization, fundamentals, stabilization and CFD modeling. Progr. Energ. Comb. Sci. 38, 156–214. doi: 10.1016/j.pecs.2011.09.003
Chen, Z. X., Doan, N. A. K., Lv, X. J., Swaminathan, N., Ceriello, G., Sorrentino, G., et al. (2018). A numerical study of a cyclonic combustor under MILD conditions using non-adiabatic tabulated chemistry. Energy Fuels 32, 10256–10265. doi: 10.1021/acs.energyfuels.8b01103
Cheong, K. P., Wang, G., Wang, B., Zhu, R., Ren, W., and Mi, J. (2019). Stability and emission characteristics of nonpremixed MILD combustion from a parallel-jet burner in a cylindrical furnace. Energy 170, 1181–1190. doi: 10.1016/j.energy.2018.12.146
Chinnici, A., Tian, Z. F., Lim, J. H., Nathan, G. J., and Dally, B. B. (2017). Comparison of system performance in a hybrid solar receiver combustor operating with MILD and conventional combustion. Part II: effect of the combustion mode. Sol. Energy 147, 479–488. doi: 10.1016/j.solener.2017.02.054
Chinnici, A., Tian, Z. F., Lim, J. H., Nathan, G. J., and Dally, B. B. (2019). Thermal performance analysis of a syngas-fuelled hybrid solar receiver combustor operated in the MILD combustion regime. Comb. Sci. Tech. 191, 2–17. doi: 10.1080/00102202.2018.1452381
Chu, H., Liu, F., and Zhou, H. (2011). Calculations of gas thermal radiation transfer in one-dimensional planar enclosure using LBL and SNB models. Int. J. Heat Mass Transf. 54, 4736–4745. doi: 10.1016/j.ijheatmasstransfer.2011.06.002
Chui, E. H., and Raithby, G. D. (1993). Computation of radiant heat transfer on a nonorthogonal mesh using the finite–volume method. Numer. Heat Transf. Part B Fundam. 23, 269–288. doi: 10.1080/10407799308914901
Dahlquist, G., and Björck, Å. (2008). Numerical Methods in Scientific Computing, Volume I. Philadelphia, PA: Society for Industrial and Applied Mathematics.
Danon, B., Cho, E. S., De Jong, W., and Roekaerts, D. J. E. M. (2011). Numerical investigation of burner positioning effects in a multi-burner flameless combustion furnace. Appl. Thermal Eng. 31, 3885–3896. doi: 10.1016/j.applthermaleng.2011.07.036
de Joannon, M., Sabia, P., Sorrentino, G., Bozza, P., and Ragucci, R. (2017). Small size burner combustion stabilization by means of strong cyclonic recirculation. Proc. Combust. Inst. 36, 3361–3369. doi: 10.1016/j.proci.2016.06.070
de Joannon, M., Sorrentino, G., and Cavaliere, A. (2012). MILD combustion in diffusion-controlled regimes of hot diluted fuel. Combust. Flame 159, 1832–1839. doi: 10.1016/j.combustflame.2012.01.013
De, A., and Dongre, A. (2015). Assessment of turbulence-chemistry interaction models in MILD combustion regime. Flow Turbul. Combust. 94, 439–478. doi: 10.1007/s10494-014-9587-8
Denison, M. K., and Webb, B. W. (1993). An absorption-line blackbody distribution function for efficient calculation of total gas radiative transfer. J. Quant. Spectrosc. Radiat. Transf. 50, 499–510. doi: 10.1016/0022-4073(93)90043-H
Dombrovsky, L. A., and Baillis, D. (2010). Thermal Radiation in Disperse Systems: An Engineering Approach. Redding, CT: Begell House.
Dorigon, L. J., Duciak, G., Brittes, R., Cassol, F., Galarça, M., and França, F. H. R. (2013). WSGG correlations based on HITEMP2010 for computation of thermal radiation in non-isothermal, non-homogeneous H2O/CO2 mixtures. Int. J. Heat Mass Transf. 64, 863–873. doi: 10.1016/j.ijheatmasstransfer.2013.05.010
Frassoldati, A., Sharma, P., Cuoci, A., Faravelli, T., and Ranzi, E. (2010). Kinetic and fluid dynamics modeling of methane/hydrogen jet flames in diluted coflow. Appl. Thermal Eng. 30, 376–383. doi: 10.1016/j.applthermaleng.2009.10.001
Fureby, C. (2000). Large Eddy simulation of combustion instabilities in a jet engine afterburner model. Combust. Sci. Technol. 161, 213–243. doi: 10.1080/00102200008935818
Galletti, C., Parente, A., and Tognotti, L. (2007). Numerical and experimental investigation of a mild combustion burner. Combust. Flame 151, 649–664. doi: 10.1016/j.combustflame.2007.07.016
Gebhart, B. (1961). Surface temperature calculations in radiant surroundings of arbitrary complexity-for gray, diffuse radiation. Int. J. Heat Mass Transf. 3, 341–346. doi: 10.1016/0017-9310(61)90048-5
Giménez-López, J., Aranda, V., Millera, A., Bilbao, R., and Alzueta, M. U. (2011). An experimental parametric study of gas reburning under conditions of interest for oxy-fuel combustion. Fuel Process. Technol. 92, 582–589. doi: 10.1016/j.fuproc.2010.11.014
Gordon, I. E., Rothman, L. S., Hill, C., Kochanov, R. V., Tan, Y., Bernath, P. F., et al. (2017). The HITRAN2016 molecular spectroscopic database. J. Quant. Spectrosc. Radiative Transf. 203, 3–69. doi: 10.1016/j.jqsrt.2017.06.038
Hewitt, G. F., Shires, G. L., and Bott, T. R. (1994). Process Heat Transfer. Boca Raton, FL: CRC Press.
Hottel, H. C., and Cohen, E. S. (1958). Radiant heat exchange in a gas-filled enclosure: allowance for nonuniformity of gas temperature. AIChE J. 4, 3–14. doi: 10.1002/aic.690040103
Huang, M., Zhang, Z., Shao, W., Xiong, Y., Liu, Y., Lei, F., et al. (2014). Effect of air preheat temperature on the MILD combustion of syngas. Energy Convers. Manag. 86, 356–364. doi: 10.1016/j.enconman.2014.05.038
Huang, X., Tummers, M. J., and Roekaerts, D. J. E. M. (2017). Experimental and numerical study of MILD combustion in a lab-scale furnace. Energy Procedia 120, 395–402. doi: 10.1016/j.egypro.2017.07.231
Karyeyen, S., Feser, J. S., and Gupta, A. K. (2019). Hydrogen concentration effects on swirl-stabilized oxy-colorless distributed combustion. Fuel 253, 772–780. doi: 10.1016/j.fuel.2019.05.008
Katsuki, M., and Hasegawa, T. (1998). “The science and technology of combustion in highly preheated air,” in Symposium (International) on Combustion, Vol. 27 (Pittsburgh, PA), 3135–3146. doi: 10.1016/S0082-0784(98)80176-8
Khalil, A. E. E., and Gupta, A. K. (2017). Towards colorless distributed combustion regime. Fuel 195, 113–122. doi: 10.1016/j.fuel.2016.12.093
Kim, H. Y., Baek, S. W., and Kim, S. W. (2012). Investigation of fuel lean reburning process in a 1.5 MW boiler. Appl. Energy 89, 183–192. doi: 10.1016/j.apenergy.2011.05.027
Kim, S. H., Huh, K. Y., and Dally, B. (2005). Conditional moment closure modeling of turbulent nonpremixed combustion in diluted hot coflow. Proc. Combust. Inst. 30, 751–757. doi: 10.1016/j.proci.2004.08.161
Koren, C., Vicquelin, R., and Gicquel, O. (2018). Multiphysics simulation combining large-eddy simulation, wall heat conduction and radiative energy transfer to predict wall temperature induced by a confined premixed swirling flame. Flow Turb. Comb. 101, 77–102. doi: 10.1007/s10494-018-9895-5
Kreith, F., Manglik, R., and Bohn, M. (2011). Principles of Heat Transfer, 7th Edn. Stamford, CT: Cengage Learning.
Krishnamoorthy, G., Sami, M., Orsino, S., Perera, A., Shahnam, M., and Huckaby, E. D. (2010). Radiation modelling in oxy-fuel combustion scenarios. Int. J. Comput. Fluid Dyn. 24, 69–82. doi: 10.1080/10618562.2010.485567
Kruse, S., Kerschgens, B., Berger, L., Varea, E., and Pitsch, H. (2015). Experimental and numerical study of MILD combustion for gas turbine applications. Appl. Energy 148, 456–465. doi: 10.1016/j.apenergy.2015.03.054
Lamouroux, J., Ihme, M., Fiorina, B., and Gicquel, O. (2014). Tabulated chemistry approach for diluted combustion regimes with internal recirculation and heat losses. Combust. Flame 161, 2120–2136. doi: 10.1016/j.combustflame.2014.01.015
Lefebvre, A. H., and Ballal, D. R. (2010). Gas Turbine Combustion: Alternative Fuels and Emissions, 3rd Edn. Boca Raton, FL: CRC Press.
Li, P., Wang, F., Mi, J., Dally, B. B., and Mei, Z. (2014). MILD combustion under different premixing patterns and characteristics of the reaction regime. Energy Fuels 28, 2211–2226. doi: 10.1021/ef402357t
Liu, B., Wang, Y. H., and Xu, H. (2015). Mild combustion in forward flow furnace of refinery-off gas for low-emissions by deflector. Appl. Therm. Eng. 91, 1048–1058. doi: 10.1016/j.applthermaleng.2015.08.078
Medwell, P. R., Kalt, P. A. M., and Dally, B. B. (2008). Imaging of diluted turbulent ethylene flames stabilized on a Jet in Hot Coflow (JHC) burner. Combust. Flame 152, 100–113. doi: 10.1016/j.combustflame.2007.09.003
Miller, C. A., Touati, A. D., Becker, J., and Wendt, J. O. L. (1998). “NOx abatement by fuel-lean reburning: laboratory combustor and pilot-scale package boiler results,” in Symposium (International) on Combustion, Vol. 27, (Pittsburgh, PA), 3189–3195. doi: 10.1016/S0082-0784(98)80182-3
Minamoto, Y., Dunstan, T. D., Swaminathan, N., and Cant, R. S. (2013). DNS of EGR-type turbulent flame in MILD condition. Proc. Combust. Inst. 34, 3231–3238 doi: 10.1016/j.proci.2012.06.041
Modest, M. F. (1991). The weighted-sum-of-gray-gases model for arbitrary solution methods in radiative transfer. ASME J. Heat Transfer. 113, 650–656. doi: 10.1115/1.2910614
Modest, M. F. (2013). Radiative Heat Transfer. San Diego, CA: Academic Press. doi: 10.1016/B978-0-12-386944-9.50023-6
Nemitallah, M. A., Rashwan, S. S., Mansir, I. B., Abdelhafez, A. A., and Habib, M. A. (2018). Review of novel combustion techniques for clean power production in gas turbines. Energy Fuels 32, 979–1004. doi: 10.1021/acs.energyfuels.7b03607
Noor, M. M., Wandel, A. P., and Yusaf, T. (2013). Design and development of MILD combustion burner. J. Mech. Eng. Sci. 5, 662–676. doi: 10.15282/jmes.5.2013.13.0064
Oldenhof, E., Tummers, M. J., van Veen, E. H., and Roekaerts, D. J. E. M. (2010). Ignition kernel formation and lift-off behaviour of jet-in-hot-coflow flames. Combust. Flame 157, 1167–1178. doi: 10.1016/j.combustflame.2010.01.002
Özdemir, I. B., and Peters, N. (2001). Characteristics of the reaction zone in a combustor operating at mild combustion. Exp. Fluids 30, 683–695. doi: 10.1007/s003480000248
Özışık, M. N. (1973). Radiative Transfer and Interactions With Conduction and Convection. New York, NY: Werbel & Peck.
Pallarés, J., Arauzo, I., and Williams, A. (2007). Integration of CFD codes and advanced combustion models for quantitative burnout determination. Fuel 86, 2283–2290. doi: 10.1016/j.fuel.2007.01.036
Pannier, E., and Laux, C. O. (2019). RADIS: a nonequilibrium line-by-line radiative code for CO2 and HITRAN-like database species. J. Quant. Spectrosc. Radiative Transf. 222, 12–25. doi: 10.1016/j.jqsrt.2018.09.027
Parente, A., Galletti, C., and Tognotti, L. (2008). Effect of the combustion model and kinetic mechanism on the MILD combustion in an industrial burner fed with hydrogen enriched fuels. Int. J. Hydrogen Energy 33, 7553–7564. doi: 10.1016/j.ijhydene.2008.09.058
Parr, T. P., Gutmark, E., Wilson, K., Hanson-Parr, D. M., Yu, K., Smith, R. A., et al. (1996). Compact incinerator afterburner concept based on vortex combustion. Symp. Combust. 26, 2471–2477. doi: 10.1016/S0082-0784(96)80078-6
Paul, C., Haworth, D. C., and Modest, M. F. (2019). A simplified CFD model for spectral radiative heat transfer in high-pressure hydrocarbon–air combustion systems. Proc. Comb. Inst. 37, 4617–4624. doi: 10.1016/j.proci.2018.08.024
Perpignan, A. A. V., Gangoli Rao, A., and Roekaerts, D. J. E. M. (2018). Flameless combustion and its potential towards gas turbines. Prog. Energy Combust. Sci. 69, 28–62. doi: 10.1016/j.pecs.2018.06.002
Rafidi, N., and Blasiak, W. (2006). Heat transfer characteristics of HiTAC heating furnace using regenerative burners. Appl. Therm. Eng. 26, 2027–2034. doi: 10.1016/j.applthermaleng.2005.12.016
Ren, T., Modest, M. F., and Roy, S. (2018). Monte Carlo simulation for radiative transfer in a high-pressure industrial gas turbine combustion chamber. J. Eng. Gas Turb. Power 140:051503. doi: 10.1115/1.4038153
Riahi, S., Roekaerts, D. J. E. M., and Lupant, D. (2013). “Numerical comparative study of heat transfer in flameless and conventional combustion in a 30-kW furnace,” in Proceedings of the European Combustion Meeting (Lund).
Rodrigues, P., Gicquel, O., Franzelli, B., Darabiha, N., and Vicquelin, R. (2019). Analysis of radiative transfer in a turbulent sooting jet flame using a Monte Carlo method coupled to large eddy simulation. J. Quant. Spectrosc. Radiative Transf. 235, 187–203. doi: 10.1016/j.jqsrt.2019.07.003
Rothman, L. S., Gordon, I. E., Barber, R. J., Dothe, H., Gamache, R. R., Goldman, A., et al. (2010). HITEMP, the high-temperature molecular spectroscopic database. J. Quant. Spectrosc. Radiat. Transf. 111, 2139–2150. doi: 10.1016/j.jqsrt.2010.05.001
Sabia, P., Sorrentino, G., Bozza, P., Ceriello, G., Ragucci, R., and de Joannon, M. (2019). Fuel and thermal load flexibility of a MILD burner. Proc. Combust. Inst. 37, 4547–4554. doi: 10.1016/j.proci.2018.09.003
Smith, T. F., Shen, Z. F., and Friedman, J. N. (1982). Evaluation of coefficients for the weighted sum of gray gases model. J. Heat Transf. 104, 602–608. doi: 10.1115/1.3245174
Sorrentino, G., Ceriello, G., de Joannon, M., Sabia, P., Ragucci, R., Van Oijen, J., et al. (2018). Numerical investigation of moderate or intense low-oxygen dilution combustion in a cyclonic burner using a flamelet-generated manifold approach. Energy Fuels 32, 10656–10667. doi: 10.1021/acs.energyfuels.8b01099
Sorrentino, G., Göktolga, U., De Joannon, M., Van Oijen, J., Cavaliere, A., and De Goey, P. (2017). An experimental and numerical study of MILD combustion in a cyclonic burner. Energy Procedia 120, 649–656. doi: 10.1016/j.egypro.2017.07.173
Sorrentino, G., Sabia, P., De Joannon, M., Cavaliere, A., and Ragucci, R. (2016). The effect of diluent on the sustainability of MILD combustion in a cyclonic burner. Flow Turbul. Combust. 96, 449–468. doi: 10.1007/s10494-015-9668-3
Szegö, G. G., Dally, B. B., and Nathan, G. J. (2009). Operational characteristics of a parallel jet MILD combustion burner system. Combust. Flame 156, 429–438. doi: 10.1016/j.combustflame.2008.08.009
Tu, Y., Liu, H., Chen, S., Liu, Z., Zhao, H., and Zheng, C. (2015). Effects of furnace chamber shape on the MILD combustion of natural gas. Appl. Therm. Eng. 76, 64–75. doi: 10.1016/j.applthermaleng.2014.11.007
Veríssimo, A. S., Rocha, A. M. A., and Costa, M. (2013). Importance of the inlet air velocity on the establishment of flameless combustion in a laboratory combustor. Exp. Therm. Fluid Sci. 44, 75–81. doi: 10.1016/j.expthermflusci.2012.05.015
Viskanta, R. (2005). Radiative Transfer in Combustion Systems: Fundamentals and Applications. West Lafayette, IN: Purdue University.
Webb, B. W., Ma, J., Pearson, J. T., and Solovjov, V. P. (2018). SLW modeling of radiation transfer in comprehensive combustion predictions. Combust. Sci. Technol. 190, 1392–1408. doi: 10.1080/00102202.2018.1452123
Wünning, J. A., and Wünning, J. G. (1997). Flameless oxidation to reduce thermal NO-formation. Prog. Energy Combust. Sci. 23, 81–94. doi: 10.1016/S0360-1285(97)00006-3
Yang, Y. B., Newman, R., Sharifi, V., Swithenbank, J., and Ariss, J. (2007). Mathematical modelling of straw combustion in a 38 MWe power plant furnace and effect of operating conditions. Fuel 86, 129–142. doi: 10.1016/j.fuel.2006.06.023
Zeng, X., Liang, C., Duan, L., Chen, X., Liu, D., and Ma, J. (2020). A GPU-based line-by-line method for thermal radiation transfer of H2O, CO2, and H2O/CO2 mixture. Appl. Thermal Eng. 167:114799. doi: 10.1016/j.applthermaleng.2019.114799
Zhang, Z., Li, X., Zhang, L., Luo, C., Lu, B., Xu, Y., et al. (2019). Effect of H2O/CO2 mixture on heat transfer characteristics of pulverized coal MILD-oxy combustion. Fuel Process. Technol. 184, 27–35. doi: 10.1016/j.fuproc.2018.11.011
Keywords: MILD combustion, heat transfer, radiation, WSGG model, blackbody
Citation: Ceriello G, Sorrentino G, Cavaliere A, de Joannon M and Ragucci R (2021) Mini-Review: Heat Transfer Mechanisms in MILD Combustion Systems. Front. Mech. Eng. 7:505923. doi: 10.3389/fmech.2021.505923
Received: 18 October 2019; Accepted: 26 March 2021;
Published: 05 May 2021.
Edited by:
Dipankar Chatterjee, Central Mechanical Engineering Research Institute (CSIR), IndiaReviewed by:
Dirk J. E. M. Roekaerts, Delft University of Technology, NetherlandsCopyright © 2021 Ceriello, Sorrentino, Cavaliere, de Joannon and Ragucci. This is an open-access article distributed under the terms of the Creative Commons Attribution License (CC BY). The use, distribution or reproduction in other forums is permitted, provided the original author(s) and the copyright owner(s) are credited and that the original publication in this journal is cited, in accordance with accepted academic practice. No use, distribution or reproduction is permitted which does not comply with these terms.
*Correspondence: Giancarlo Sorrentino, Zy5zb3JyZW50aW5vQHVuaW5hLml0
Disclaimer: All claims expressed in this article are solely those of the authors and do not necessarily represent those of their affiliated organizations, or those of the publisher, the editors and the reviewers. Any product that may be evaluated in this article or claim that may be made by its manufacturer is not guaranteed or endorsed by the publisher.
Research integrity at Frontiers
Learn more about the work of our research integrity team to safeguard the quality of each article we publish.