- 1Bioceramics and Coating Division, CSIR-Central Glass and Ceramic Research Institute, Kolkata, India
- 2Centre for Healthcare Science and Technology, Indian Institute of Engineering Science and Technology, Shibpur, India
The field of Tissue engineering and regenerative medicine that work toward creating functional tissue-constructs mimicking native tissue for repair and/or replacement of damaged tissues or whole organs have evolved rapidly over the past few decades. However, traditional tissue engineering approaches comprising of scaffolds, growth factors and cells showed limited success in fabrication of complex 3D shapes and in vivo organ regeneration leading to their non-feasibility for clinical applications from a logistical and economical viewpoint. In this regard, 3D bioprinting, which is an extended application of additive manufacturing is now being explored for tissue engineering and regenerative medicine as it involves the top-down approach of building the complex tissue in a layer by layer fashion, thereby producing precise geometries due to controlled nature of matter deposition with the help of anatomically accurate 3D models of the tissue generated by computer graphics. Here, we aim to provide a comprehensive review of the 3D bioprinting technology along with associated 3D bioprinting strategies including ink-jet printing, extrusion printing, stereolithography and laser assisted bioprinting techniques. We then focus on the applications of 3D bioprinting technology on construction of various representative tissue and organs, including skin, cardiac, bone and cartilage etc. We further attempt to highlight the steps involved in each of those tissues/organs printing and discuss on the associated technological requirements based on the available reports from recent literature. We finally conclude with current challenges with 3D bioprinting technology along with potential solution for future technological advancement of efficient and cost-effective 3D bioprinting methods.
Introduction
Tissue damage and degeneration is a rather common phenomenon among humans; however, the regenerating capabilities of human body are rather insufficient to deal with this trauma. The traditional methods for treating these conditions is dependent upon tissue or organ transplantation which is again dependent upon the availability of a donor which can be rather scarce and comes with the risk of graft rejection due to immune response. Tissue engineering and regenerative medicine are rapidly evolving fields that work toward solving these issues (Bose et al., 2012). Additive manufacturing is one of the most advanced techniques that has been utilized in this area of tissue engineering. It encompasses the principles of material science with biology for the fabrication of organ and tissue framework (Bose and Bandyopadhyay, 2019; Bandyopadhyaya, 2020). Its primary objective is the restoration of damaged tissues or organs, with its fundamental goal being to emulate the native complexity of biological tissue (cellular niche) that will aid in the cell differentiation and tissue regeneration. Traditionally, this process requires the formation of an interphase between cell, scaffolds and growth factors. Scaffolds can provide the base on which cells can grow under the influence of growth factors (Satpathy et al., 2019). However, this process is rather random in nature and does not allow for a specific customized 3D distribution of cells or matrix (Bose et al., 2013), in addition to being time consuming and less efficient. This leads to their non-feasibility for clinical applications from a logistical and economical viewpoint (Singh and Williams, 2008). With this regard, additive manufacturing is now being explored for tissue engineering as it involves the top-down approach of building the complex tissue in a layer by layer fashion, thereby producing precise geometries due to controlled nature of matter deposition with the help of anatomically accurate 3D models of the tissue generated by computer graphics (Melchels et al., 2012).
3D bioprinting is an extended application of AM that involves building a tissue or organ layer-by-layer using bottoms-up approach. The aim of 3D bioprinting is to somehow mimic the natural cellular architecture by depositing materials and cells in a particular fashion which can restore the normal structure and functionality of complex tissues. In 3D bioprinting, cells or biomolecules are printed directly onto a substrate in a specific pattern such that the cells can hold together to form the required 3D construct (Xiongfa et al., 2018). Bioprinting deals with the living entities such as cells, tissues, etc., hence the modalities associated with the living tissues has to be observed in it, such as biocompatibility of the material being used, cell sensitivity to the printing methods, growth factor delivery and perfusion etc. (Murphy and Atala, 2014). Since the whole process is automated, it can give precise patterning of cells with controlled ECM organization. Because of the layer-by-layer construction of the bio printed tissues, they possess interconnected pores which are ideal for perfusion of gas and nutrients, as well as inter- and intra-cellular communications (Knowlton et al., 2018). These bio-printed tissues with improved intercellular communications can give a decent reference to in vivo physiology. Such a result can contribute toward the data obtained during pre-clinical trials, since animal model is not sufficiently equipped to predict human pathophysiological responses (Shanks et al., 2009).
One of the foremost requirements for 3D bioprinting is bioink. It is composite made up of biomaterials, cells, and other required components (Ozbolat, 2015a). The technology can be used for fabrication of functional human tissue or organ such as heart, liver, skin, bones etc., along with generating microfluidic models of organs-on-a-chip in the near future (Guillemot et al., 2011). However, despite these advantages and convenience offered by the 3D bioprinting, the state-of-the-art technology involves several challenges such as vascularization of the tissue, gas and nutrient exchange, biocompatibility and biodegradability of the material that is used as substrate, shape-fidelity and preservation of functionality of the printed tissue (Xu et al., 2012). To this effect, synthetic and natural polymers such as alginate, gelatin, collagen, Polyethylene glycol (PEG), Hydroxyapatite etc., because of their biocompatible nature and controllable physio-chemical properties that can be modified to suit the ECM structure and formation (Tevlek and Aydin, 2017; Bodhak et al., 2010).
This article aims to provide a comprehensive review of the 3D Bioprinting Process along with the different strategies involved in it such as ink-jet printing, extrusion printing, stereolithography and laser assisted bioprinting techniques. 3D Bioprinting is a very versatile technique which can be used for printing of complete organs. Here, we have tried to present an overview of bioprinting of skin tissue, cardiac tissue, bone and cartilage and to highlight the technological requirements and challenges associated with their bioprinting. The review outlines the process of bioprinting of the above tissues and the direction in which the current research is heading. The state-of-the-art literature in this field is concerned with narrow applications of the bioprinting technique. Hence, there was a need to present a comprehensive account of the requirements and procedures of different types of tissue in one place. This review aims to focus at different strategies applied toward bioprinting of natural and synthetic polymers as well their applications in different types of tissue engineering with respect to 3D printing of various tissue models such as bone, skin, cardiac and cartilage tissues etc. This allows us to acknowledge the difference between the procedure and technological requirements as the tissue type varies from soft tissue such as skin to the hard tissue such as bone and cartilage.
3D Bioprinting Strategies
The procedure of 3D printing is based on the exact layering of biomaterials. Briefly, the process follows a general outline in the form of three basic steps: Preparatory phase, Printing phase and Post-handling. Preparatory phase is the designing of anatomically accurate 3D models via computer graphics software such as CAD/CAM and rendering it into stack of 2D layers of user-demarcated thickness which will be fed into the bioprinter for printing. This step also included the material or bio-ink selection. The processing step involves the actual printing of the tissues by additive manufacturing techniques. Post-processing refers to the maturation of the fabricated construct in a bioreaction and it’s structural and functional characterization (Papaioannou et al., 2019). The flow-diagram given in Figure 1 gives an overview of the bioprinting process and the steps involved in it.
Bioprinting can be done either scaffold-based or scaffold free. In the scaffold based method, the biomaterial matrix forms the stratum for cellular deposition. This matrix can be hydrogel, nanofibers or films or any other 3D construct onto which the bioink can be patterned. Here, it is important to note that the 3D construct should closely mimic the native ECM environment such that it allows cells to grow and proliferate. In comparison, scaffold-free bioprinting involves direct deposition of cell or tissue aggregates in the form of spheroids, honeycomb, cylinder etc. The higher numbers of cells are expected to initiate ECM deposition on its own in the confined space of 3D print mold (Ozbolat, 2015b). The whole process involves loading the tissue spheroids into pipettes and then depositing it into printing molds via extrusion mechanism. The cells secrete their own ECM and form a network leading to maturation of the tissue and finally the mold is removed. The mold is just used as a supporting material and is itself not utilized. This method allows for the freeing up of cells from biomaterial that limit cellular interaction and slow down the cell growth. Self-organization of cells increases ECM production and preserves the tissue functionality (Khoshnood and Zamaniain, 2020). Several kinds of additive manufacturing techniques have been developed for selective patterning of cells and biomaterials for fabrication of viable tissue constructs such as inkjet based 3D bioprinting (Cui and Boland, 2009), extrusion based 3D bioprinting (Jones, 2012), laser assisted 3D bioprinting (Keriquel et al., 2017), and stereolithographic based 3D bioprinting (Dean et al., 2012) etc. Each of these 3D bioprinting techniques has been summarized in the following sections.
Inkjet Based 3D Bioprinting
This method employs the use of “bioink,” which is simply a low-viscosity suspension biomaterial along with viable cells etc. that can be deposited over a “bio paper” such as hydrogel substrate, culture dish or a polymer construct etc. This AM technique is a non-contact printing technique, where the printing takes place in a digitally controlled pattern. Ink-jet printing can be done in two basic ways, either in a continuous manner (continuous ink-jet printing) or in a drop-on-demand (DOD) fashion. In the continuous ink-jet printing, a continuous jet of droplets is created by applying pressure on the bioink which forces it out of a nozzle. Electric field is then applied which deflects this jet of bio-ink onto the substrate. The excess droplets which do not form the required pattern are deflected toward a gutter where they are collected for reuse. In drop-on-demand inkjet printing, the method employed for creating droplets is similar to CIJ, with the difference that the droplets are produced only on demand. A pressure pulse is hence used to force out the droplets instead of a continuous pressure. Bioprinting is better suited to DOD approach of printing because of its pulsed nature, since in CIJ approach, the ink that is not deflected onto the substrate is recirculated through the printer, thereby posing a risk of contamination. Other reason that CIJ is not particularly suitable for bioprinting is the need for conductive ink (Alamán et al., 2016). On the basis of droplet instigation mechanism, the DOD printing mechanism can be categorized into piezoelectric and thermal ink-jet printing. Thermal DOP utilizes pulsed electric current to a heating element. This heating element quickly vaporizes ink droplets in the microfluidic chamber and ink droplet is pushed onto the substrate through the nozzle orifice because of the pressure created by the vapor bubble. The cells are exposed to only a few microseconds of high temperature, hence they do not rise too much above the ambient temperature and thus remain viable (Cui et al., 2010). Piezoelectric DOD employs a piezoelectric transducer in the microfluidic chamber above the nozzle. Similar to thermal DOD, in this also pulsed voltage is applied to the transducer which creates the transient pressure for droplet actuation. The ability of the bioink to be printed is governed by the rheological properties of the ink in both forms of printing mechanisms. While the actual requirements for the ink differs depending upon the system, in general a typical viscosity requirement is 30 mPa/s (Derby, 2008). Droplet size is also affected by other attributes such as nozzle size, substrate to nozzle distance, temperature gradient in case of thermal DOD, piezo-deformation characteristics of the transducer in case of piezoelectric DOD as well as the frequency of the current applied. This technique has already been effectively used for mammalian cell printing and patterning in addition to DNA (Okamoto et al., 2000) and proteins (Delaney et al., 2009).
Inkjet printing is particularly advantageous because of its cheap and non-contact nature which reduces the chances of contamination (Dababneh and Ozbolat, 2014). Recently, Xu and his team developed vascular like alginate tubes with a hemi branching point using drop-on-demand inkjet printing, by utilizing a platform assisted 3D inkjet bioprinting device (Xu et al., 2012). A major characteristic of ink-jet printing is its ability to allow the formation of complex multicellular patterns and constructs by simultaneous printing of multiple cell types, biomaterials etc., in a single-fabrication operation by using different printheads. The evolution of 3D printing of tissues and organs started with the ink-jet bioprinting only. Cell bioprinting has been done by both thermal and piezoelectric DOD printers have been explored for cell bioprinting as depicted in Figure 2 (Kačarević et al., 2018), however thermal ink-jet printers have been more preferred (Cui et al., 2012).
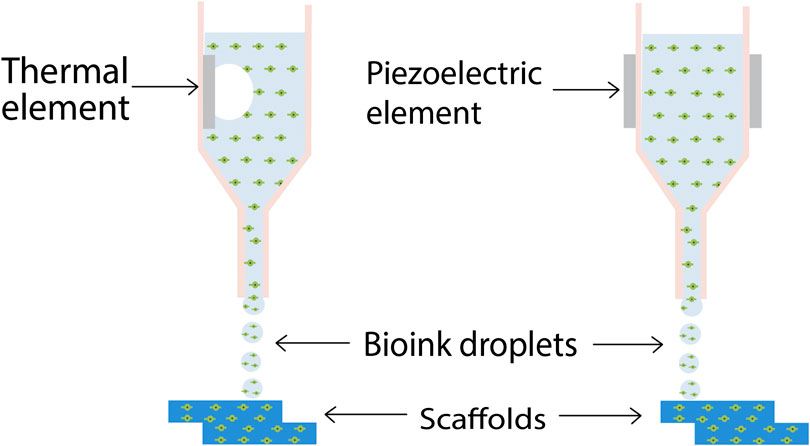
FIGURE 2. Schematic diagram of inkjet 3D bioprinting. Thermal inkjet printing induces droplet formation by means of a heating element, while in piezoelectric ink-jet printing, a piezoelectric element induces the droplet nucleation (Kačarević et al., 2018) (used from an open access journal).
Extrusion Based 3D Bioprinting
Extrusion based bioprinting can be done by Direct ink writing (DIW) or pressure-assisted bioprinting methods as shown in Figure 3 (You et al., 2017). Direct ink writing is a material extrusion process in which the apparatus continuously extrudes material out of the nozzle, generating 3D architectures layer-by-layer. Suitable materials for DIW should possess specific rheological properties, which enable easy printability. The material should be shear thinning to enable extrusion out of the printing nozzle. It should also possess a shear yield stress. To induce flow, a shear stress above the yield stress of the resin is applied. Subsequently, the shear stress is released, and the resin recovers its rigidity when placed on a substrate. Polymer resins are commonly blended with fillers, e.g., silica particles or nano-clay to achieve desired rheological properties. The fillers induce shear thinning flow behavior and at optimal resin/filler compositions they can afford a material which possesses a shear yield stress. These rheological properties enable shape retention of the printed object, rendering self-standing structures (Truby and Lewis, 2016). Alternative solidifying processes include subsequent UV-curing of the printed layer, thermal cure or extrusion into a support bath. The latter holds the printed structure in place until the deposited ink is converted into a solid. This process is often called “freeform reversible embedding” (FRE) or embedded 3D printing (e-3D printing). Angelini et al. recently described this concept in detail and provided an overview of the required rheological properties of the support material (O’Bryan et al., 2017). Overall, the minimum printing resolution for viscous polymer resins using DIW range from hundreds of microns to the sub-microns range and is usually dictated by the nozzle dimensions (Lewis and Gratson, 2004).
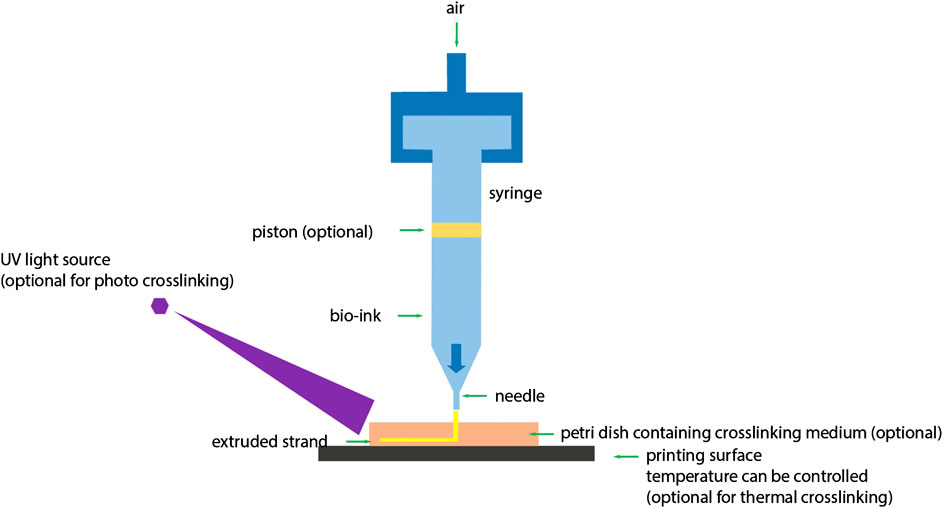
FIGURE 3. Schematic diagram of extrusion based 3D bioprinting. In extrusion based 3D bioprinting, application of pressure on bioink is optional (You et al., 2017) (used from an open access journal).
Traditional scaffolding processes such as solvent casting, electrospinning and salt-leaching, etc., lack the controlled pore architecture that is obtained from the CAD/CAM controlled additive manufacturing processes. To remedy this, pressure-assisted deposition has been used traditionally. Polymers such as Polycaprolactone (PCL), Polylactide (PLA), etc., and their blends or composites with ceramics such as tricalcium phosphate (TCP) and Hydroxyapatite (HAP) have been the focus for design and optimization of scaffolds by these processes (Xiong et al., 2001; Park et al., 2011). With the advent of organ bioprinting, the focus has shifted toward cell-encapsulated hydrogels obtained by direct printing. In one of the studies, researchers have encapsulated rodent hepatocytes in gelatin hydrogels in conjunction with alginate, chitosan and fibrinogen for fabrication of a functional liver construct by employing pressure-assisted multi-syringe deposition system. Thermal cross-linking of gelatin is done initially as it moves onto a warmer stage from a low-temperature syringe via extrusion. The construct is then strengthened through chemical cross-linking. The construct showed considerable cell viability and function as analyzed by liver tissue markers. The gelatin-chitosan constructs were difficult to stabilize because of enzymatic degradation, however the method still showed simultaneous deposition of cells and biomaterials (Xu et al., 2007). Gelatin-based hydrogels have been used by other researchers also for fabrication of biomimetic 3D constructs for hepatocytes and adipose-derived stem cells (Li et al., 2009). A study conducted by Fedorovich et al. (2012) have showed the incorporation various cells in Matrigel® such as bone grafts, chondrocytes for osteochondral grafts, etc. to form a multicellular, biomimetic construct.
Laser Assisted 3D Bioprinting or Laser Induced Forward Transfer
A pulsed laser beam is utilized in this process for deposition of bio-ink including cells onto a substrate. Utilization of laser for deposition of materials provides a non-contact direct writing process for 3D printing. As is visible in Figure 4 (Keriquel et al., 2017), there are three key elements to Laser Assisted 3D Bioprinting (LAB): a pulsed laser source, a ribbon coated with bio-ink and a receiving substrate on which the bio-ink is to be deposited. UV lasers or near UV wavelength lasers with nanosecond pulse wavelength are used as the energy source. The laser serves to cause volatilization of the heat-sensitive bio-ink from the “ribbon.” The bio-ink is coated onto a target plate made up of either quartz or that allows the transmission of laser through it. Viable cell transfer is assisted by a laser-absorbing, sacrificial interlayer between the bio-ink and ribbon depending upon the optical characteristics of laser and the ink. The substrate in which the ink is to be deposited is also coated with either a natural polymer or nutrient medium or a biopolymer to facilitate the deposition process and sustain cell growth. The bio-ink is volatile in nature, hence on application of a laser pulse, a high-speed jet of cell laden bioink is propelled onto the substrate (Barron et al., 2005). Researchers have developed “absorbing film-assisted laser-induced forward transfer (AFA-LIFT)” or “biological laser processing (BioLP)” (Barron et al., 2004; Hopp et al., 2004) along with “matrix-assisted pulsed laser evaporation direct writing (MAPLEDW)” (Patz et al., 2006).
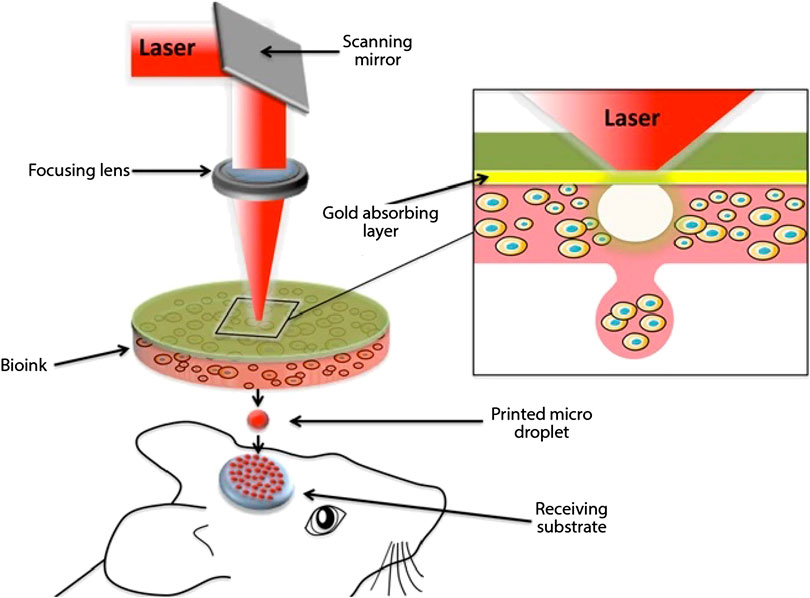
FIGURE 4. Schematic representation of laser assisted 3D bioprinting (Keriquel et al., 2017) (used from an open access journal).
On the other hand, laser induced forward transfer (LIFT) based technique was originally proposed by using a high-energy laser pulse for direct writing of metal features on an optically transparent substrate by direct deposition of it. This technique was extended for printing of biomolecules in the form of AFA-LIFT and BioLP (Duocastella et al., 2007). A laser-absorbing layer of any metal or its oxide (e.g., Ti, TiO2, Ag, etc.) is included at the interface of ribbon and bioink in the form of sacrificial layer to protect the cells from laser exposure. Application of a high-energy pulsed laser causes rapid thermal expansion of this sacrificial layer which allows for propulsion of small volume bio-ink onto the substrate with minimal cell damage. BioLP process slightly deviates from this in that it utilizes a low-powered pulsed laser and the sacrificial layer is a hydrogel such as Matrigel®. In this case, the hydrogel itself acts as binding medium for bio-ink onto the target plate or ribbon. The whole process is computer-controlled and employs CCD camera that allows for selective cell patterning (Nahmias et al., 2005). Cells can either be printed as encapsulated particles in ECM-like biomaterial or they can be directly imprinted onto/in the depths of ECM layer. Different parameters that effect cell viability during printing process are ECM thickness onto which the cells are deposited, the laser-pulse energy, viscosity of bioink, etc. High laser energy increases cell fatality, while increasing the thickness of sacrificial layer and bio-ink viscosity resulted in greater cell viability. Researchers (Guillotin et al., 2010) also studied the effects of printing speed on printing resolution. Their work provides evidence that fabrication of soft free form tissue that is able to host a high cell density in vivo by printing blends of cells onto the ECM via LAB.
Stereolithographic Based 3D Bioprinting
Stereolithographic method of bioprinting as depicted in Figure 5 (Derakhshanfar et al., 2018) is dependent upon the height of the design rather than its complexity as it builds up the design in a layered fashion by addition of materials by projecting light on a photo-sensitive heat-curable bio-ink in a plane by plane fashion (Morris et al., 2017). As the printing system employs light as an agent for cross-linking, the ink must have some photocurable moieties (Hollister et al., 2002). Acrylate derivatives of Polyethylene Glycol (PEG) such as PEG dimethacrylate (PEGDMA) and PEG diacrylate (PEGDA) are some of the widely employed moieties for photopolymerization of tissue engineering scaffolds (Zhu et al., 2006). Formation of 3D tissue constructs is accomplished by light-initiated polymerization (Mandrycky et al., 2016). Stereolithography has been coordinated with clinical imaging techniques such as CT scan/MRI for improvement of diagnostic techniques, quality and design of prosthesis and implants and useful achievement of complex surgeries. Two broad categories of stereolithographic printing is Single-photon method and Multiphoton method. Single photon method can be further subdivided into: 1) Visible radiation systems, 2) Conventional stereolithography, 3) IR stereolithography systems, 4) Stereo-thermal lithography systems. Light projection systems can be either directly implemented by laser writing, or mask projection systems can be used either physically or digitally (Bártolo, 2011). “Conventional single-photon stereolithography apparatus (SLA)” can cross-link UV sensitive fluid oligomers into sol-gel polymeric networks by using the photons from the UV radiations. A photosensitive resin has been utilized for this purpose that helps in polymerization by following three steps: initiation, elongation, and termination. A variety of resins gave been applied for SLA purposes, such as biodegradable resins for nontoxicity, elastomeric resins for flexibility and high strength resins for mechanical strength (Kačarević et al., 2018).
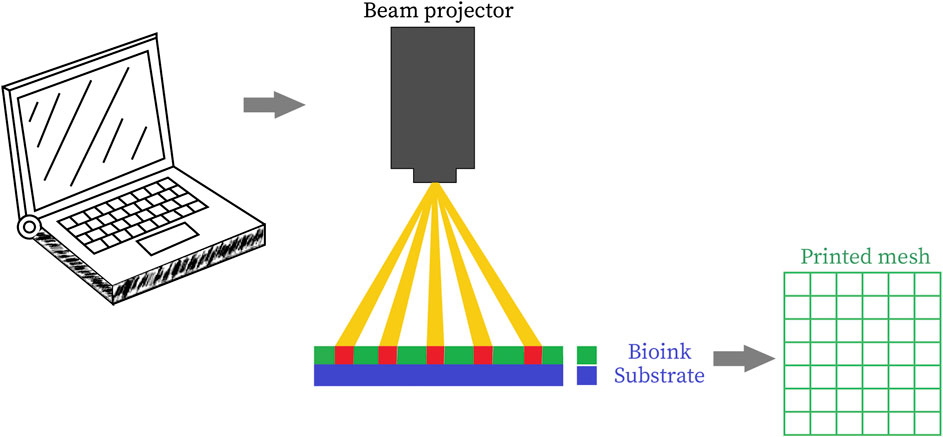
FIGURE 5. Schematic representation of stereolithographic 3D bioprinting (Derakhshanfar et al., 2018) (used from an open access journal).
Stereolithographic method has been admirably used in tissue engineering for fabrication of biocompatible scaffold in which resins help to prevent inflammatory responses during implantation as well good degradability with nontoxic by-products that results absolute renal clearance with tissue regeneration. Chu et al. (2002) demonstrated SLA to polymerize UV curable polymer-ceramic composites utilized for cell-seeding, by applying it to the minipig model bone regeneration can be showed. Another research group showed that utilizing vinyl ester resin bone regeneration scaffold can be fabricated by utilizing vinyl ester resin upon rabbit model for bone ingrowth and regeneration in defective site (Heller et al., 2009). A bioresorbable scaffold based on Hyaff 1, a hyaluronic acid derivative can be prepared by applying clinical imaging along with stereolithography based on UV lasers for utilization in cartilage tissue engineering (Naumann et al., 2003). Bashir and co-workers fabricated a two-dimension cardiac tissue construct in the form of connected cell sheets by utilizing primary cardiomyocytes isolated from neonatal rats via stereolithographic printing technique (Chan et al., 2012a). Another research group fabricated a tri-leaflet heart valve by stereolithographic printing of polyhydroxy octanoate and poly-4-hydroxybutyrate elastomers (Sodian et al., 2002). Vascular endothelial growth factor (VEGF) like molecules were shown to be secreted by fibroblast cells encapsulated in Poly ethylene glycol -based hydrogels that were fabricated by direct “laser-writing.” Secretion of VEGF is an evidence of neovasculature geometry and angiogenesis is tissue engineering (Jeong et al., 2012). Similarly, two-photon laser scanning photolithography was used to fabricate 3D liver tissue construct. They selectively polymerized photo-sensitive polymers with the help of pulsed laser and then functionalized it via collagen seeded with rat hepatocytes (Hsieh et al., 2010). Recently, a soft robotic device by the name of “biobot” has been developed by Bashir and coworkers that incorporates the auto-rhythmic and synchronous nature of cardiac tissue for locomotion purposes (Chan et al., 2012b). Some challenges related to stereolithographic methods related to fabrication of constructs using multiple materials, spatiotemporal regulation of deposition of materials onto the substrate matrix etc.
Some of the key features and applications of the above described 3D bioprinting processes are mentioned in Table 1 given below.
Organ Regeneration via 3D Bioprinting Techniques
3D Bioprinting of Skin Tissue
As depicted in Figure 6 (Varkey et al., 2019), human skin has evolved into a complex structure with epidermis and dermis forming a major part and subcutaneous tissue forming the third region. Such a structure serves to protect the body from exposure to UV rays, prevents drying of skin as well as acts as barrier preventing the entry of toxins, pathogens etc. to the body. Skin is also known as first line of defense in the immune system (Vijayavenkataraman et al., 2016). The upper layer of epidermis is predominantly made up of keratinocytes which are arranged in keratinized stratified squamous epithelium. The growth of epidermis is from inwards to outwards, with the mature cells at the surface, and the proliferating keratinocytes at the bottom, in the basal layer. This basement membrane also acts as separation between epidermis and dermis. The proliferative cells undergo through differentiation in a sequential manner with the newer, undifferentiated cells at the bottom and terminally differentiated cells toward the outside in stratum corneum. Protection from UV rays is a function of melanin that is secreted by melanocytes. It is also responsible for skin pigmentation that gives the skin its characteristic color (Swope et al., 2002). Other cells in the epidermis include nerve endings and glandular ducts as well as cells of immune system such as Langerhans cells and T-cells. The second layer of skin, i.e., dermis is also made up of two layers; the upper papillary dermis which consist of loose, areolar connective tissue and consists of dermal papillae that protrude through the epidermis creating ridges in it that leave fingerprint while sweating, and the lower reticular dermis which is made up of dense, irregular connective tissue (Brown and Krishnamurthy, 2018). The papillary region contains high ratio of type III collagen, while the reticular region has type I collagen in higher amount. This difference in the collagen ratio in the Extra Cellular Matrix of dermis is responsible for the elasticity and mechanical strength of the skin (Hinderer et al., 2016). Dermis is the bridge between the rest of the body and skin because of the many cell types contained in it, which include vasculature, neurons, hair follicles, etc.
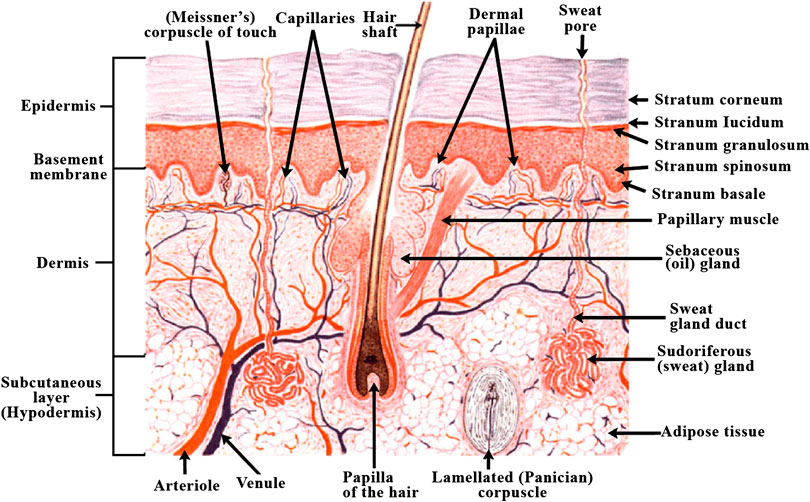
FIGURE 6. Diagram of human skin structure (Varkey et al., 2019) (used from an open access journal).
Because of the robustness of skin and its vitality, the creation of a skin construct in lab becomes of great importance. One of the earliest innovations in this field is creation of artificial skin grafting, that can act as bandage for wound and burn healing. Reconstruction of functional skin tissue by bioprinting has been approached by several research groups till date (Cubo et al., 2016; Pourchet et al., 2017). Bioprinting of skin construct has some of the obvious advantage over traditional fabrication methods in that the process is automated and by and large can be standardized for clinical application. This also ensures precision in cell deposition. Bioprinting also enables to avoid the long production process and times to obtain constructs with large surface area as burn wound typically require when compared to traditional scaffold based cell cultures (Cubo et al., 2016). Bioprinting can be done either directly at the site of injury, i.e., in situ bioprinting, or it can be done in in vitro manner, where the constructed is allowed to mature in a bioreactor before being transplanted. In situ bioprinting is rather advantageous that in vitro process, as it provides higher precision in cell deposition on the wound and eliminates unnecessary usage of expensive biomaterials as well as time required for in vitro differentiation (Ozbolat, 2015b).
Steps Involved in 3D Bioprinting of Skin Tissue
The whole process of skin bioprinting consists of four major steps; the first of which includes cell and biomaterials selection. This process is known as pre-processing, and is followed by the actual printing process. The printing process is followed by post-processing step that includes cell proliferation and maturation of printed skin construct. The final step in human skin bioprinting is the characterization of the printed tissue and its functional evaluation. The cells for printing process are obtained from skin biopsy, and then these are expanded via cell culture methods. The bio-ink is prepared by an amalgamation of cells and supporting biomaterials. The cells for bioprinting can be either primary cells, if the donor has healthy skin, or they can be stem cells if the donor has injured skin. Stem cell sources can differ on a case to case basis and range from adipose to mesenchymal as well as prenatal cells. Clinical images of the damaged area can be obtained by imaging techniques such as nuclear imaging techniques like PET, magnetic resonance imaging (MRI), etc. These images can then be used as input to design anatomically accurate models of the functional tissue in the form of STL files using CAD/CAM graphic interphase (Catros et al., 2015). The STL model can then be sliced into layers that characterize the boundary and features of each slice that is then used to create path for bioprinter heads. The bioprinter reads the STL file in a layered fashion for deposition of the bio-ink. The thickness of these slices usually lies in the range of 100–500 μm for the inkjet and extrusion based bioprinters, while laser assisted bioprinters have a resolution of 20–100 μm. It is important to note that printing resolutions below 100 μm provide precise patterning of cell-laden constructs in tissue models. High-quality image acquisition from clinical imaging is a prerequisite for precision bioprinting because the quality of fabricated construct is completely dependent upon the accuracy of the anatomical model. Although in vivo cell distribution can be realized by clinical imaging, it is rather difficult to utilize image processing tools to obtain anatomically accurate skin geometry. This is the reason that maturation of the printed tissue construct is required, especially in case of in vitro bioprinting, in which the printed construct undergoes maturation in a bioreactor as compared to in situ printing in which maturation occurs on the body itself at the injury site where the construct is printed.
Bioinks for 3D Bioprinting of Skin Tissue
The bio-ink that is chosen for the printing purpose should possess the desired biomechanical properties that will aid in deposition of ink in the patterns as specified in the STL file created by CAD modeling. This is essential as bio-ink facilitates the necessary cell-ECM interactions as well as affect the cell growth and proliferation. Bio-ink should be biocompatible and must support the morphology and function of the fabricated skin tissue construct. It should be able to facilitate cell differentiation as per the functionality required of it (Varkey et al., 2018). The choice of biomaterials for bio-inks can vary from natural polymers such as alginate, gelatin, collagen, hyaluronic acid, etc. to the man-made polymers like poly(lactic-co-glycolic acid) (PLGA), Polyethylene Glycol (PEG), Polycaprolactone (PCL), etc., or it can be a hybrid blend of natural and synthetic biomaterials (Arslan-Yildiz et al., 2016). Cells can be kept together during the bioprinting process by using materials such as Pluronic F-127 as sacrificial support materials. These materials could be simply washed away in the post-processing or maturation process. Some of the properties of the bio-ink that are considered while choosing them for bioprinting are the rheological properties of the ink, it’s gelation kinetics, shape fidelity and printing resolution of the ink (Parak et al., 2019). Researchers have worked on bio-ink suspension composed of amniotic fluid-derived stem cells (AFSCs) and bone-marrow derived stem cells suspended in fibrin-collagen, crosslinked with thrombin to be printed directly on wound site. Printing of primary human keratinocytes and fibroblasts on wound in athymic nude mice was done through ink-jet printing which led to complete re-epithelialization of wound in 8 weeks. This was further verified in larger pig-wound models (Varkey et al., 2019). We have summarized some of the important biomaterials that can be used as bioink for the printing of skin along with techniques commonly employed for the printing as well as the functionality provided by them in Table 2.
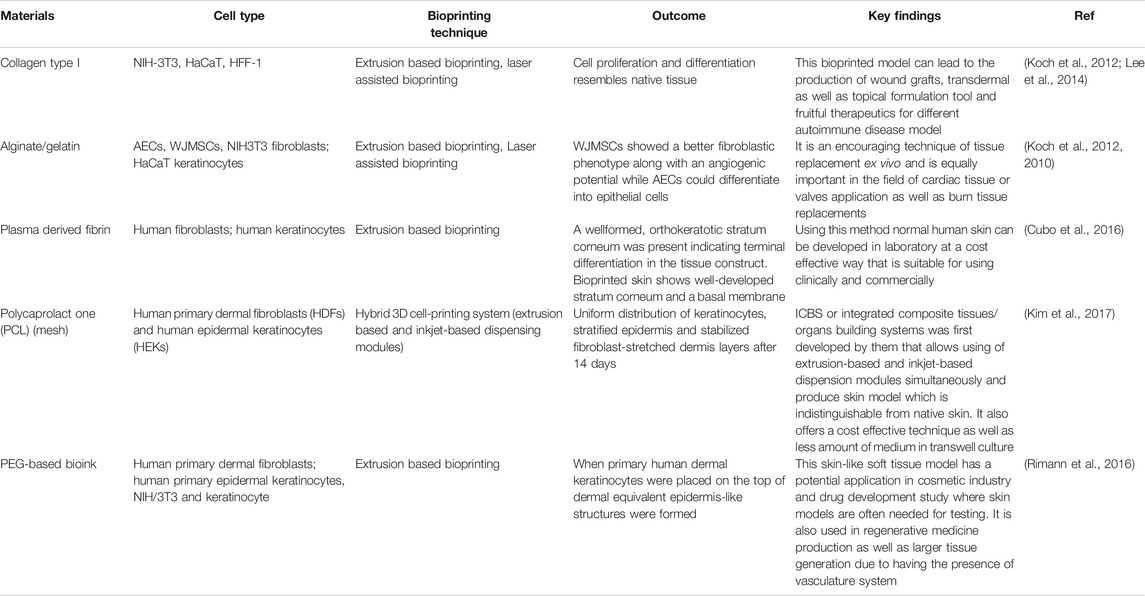
TABLE 2. Comparative summary of different 3D bioprinting techniques used in skin tissue regeneration.
3D Bioprinting of Cardiac Tissue
One of the major causes of mortality in terms of worldwide diseases are Cardiovascular diseases (CVDs), especially in the developed countries. An approximate estimation of total incidences of myocardial infarction per year gives a figure of about eight million. Other disease conditions such as stenosis etc., affect heart valves (Leoni and Rello, 2017). A major problem with all these heart related conditions is the loss of irreplaceable cardiomyocytes, as these cells lack any kind of repair or auto-regeneration process. Instead, the loss of cardiomyocytes is dealt by formation of non-functional scar tissue that increases the risk of acute cardiomyopathy exponentially. Currently, these conditions are managed by bypass grafting of coronary artery, cell therapy, left ventricular assist device etc., with the final option being heart transplantation (Tchantchaleishvili et al., 2014). This brings us to the next major issue of lack of donors for transplant, and the risks associated with the transplantation process including immune rejections leading to a not so encouraging success rate. Tissue engineering serves to mitigate these problems related to repair of damaged blood vessels, heart valves, etc. (Jawad et al., 2007). Traditional methods of cardiovascular tissue engineering involve the growth, maturation and proliferation on functional biomaterial scaffold to support stem cell differentiation. The scaffold fabrication for cardiovascular tissue engineering has been attempted from decellularized tissue matrices as well as synthetic and natural hydrogels because of their biocompatibility and similarity to the native tissue matrix of the cells (Zhu and Marchant, 2011). Autologous and allogenic stem cells are the cells of choice for cardiac tissue engineering because of reduced risks due to immune rejection of grafts and their widespread availability (Bursac, 2009).
Formation of a functional cardiac construct is a challenging endeavor because of the complexity of cardiac tissue which requires the integration of cells from multiple stem cell sources such as cardiomyocytes, fibroblasts, and endothelial cells. The difficulty also arises in attaining the auto-rhythmic nature of myocardium (Vunjak-Novakovic et al., 2010). 3D bioprinting can be useful in overcoming these challenges. It is capable of building a functional cardiac construct in a layer-by-layer approach. Multiple efforts have been made for restoration of functional myocardium via 3D printing in biomaterials such as scaffolds or tissues-on a-chip.
Steps Involved in 3D Bioprinting of Cardiac Tissue
Similar to the skin bioprinting, cardiac bioprinting also involves the pre-processing, actual printing and post-processing steps. The first step in the bioprinting of functional cardiac construct is the creation of a 3D model via graphic modeling interphases such as CAD/CAM by utilizing the clinical imaging data from MRI and CT scans as input. High fidelity models can be obtained by medical imaging techniques such as nuclear imaging techniques like PET, Computed tomography (CT) scan, volumetric 3D echocardiography etc. which can provide volumetric images (Bücking et al., 2017). Generation of patient specific 3D model of the desired cardiovascular tissue is then possible with the help of image segmentation processes. The first step is the identification of the anatomic geometry of the targeted tissue by exporting the acquired data set from clinical imaging datasets into a digital imaging. The targeted cardiac tissue can then be segmented on the basis of the threshold intensity of pixels in the greyscale 2D image projections in various anatomical planes such as axial, sagittal, and coronal (Ripley et al., 2016). The next step is stacking of individual 2D images in the form of segmentation masks so that the pixels falling in the same intensity ranges are printed with a single material (Noecker et al., 2006). These masks or layers are then rendered into STL files via computer graphics where they can be altered to increase patient specificity. These STL files can be exported to bioprinter for printing of the tissue construct (Valverde et al., 2015). The tissue constructs thus fabricated can further undergo maturation in a bioreactor for functionality to accustom the construct for contraction, blood delivery etc. These can be characterized for mechanical and electrical stimulation to realize if it can sustain contractions and relaxations for elongated time periods, while maintaining the tissue morphology (Zhang et al., 2012). One of the major issues with cardiac bioprinting is the preservation of tissue morphology as the average adult has a resting heart rate of 70–80 bpm, which means that the printed tissue will be undergoing at least 70–80 contraction-relaxation cycles in a minute. Additionally, perfusion of heart tissue must be ensured in post-processing of the printed cardiac constructs (Eng et al., 2016).
Bioink for 3D Bioprinting of Cardiac Tissue
Similar to the bio-inks for skin bioprinting, cardiac bioprinting also requires some of the features in the ink such as the spatial control of hydrogel deposition by means of formation of stable filaments with gentle cross-linking mechanisms. Maintenance of cell viability is a major pre-requisite of bio-inks for cardiac bioprinting. Natural and synthetic polymers with capability to form hydrogels gelatin, collagen, hyaluronic acid, etc. are preferred for bio-ink formation (Tomov et al., 2019). The actual process of cardiac bioprinting can be done either with scaffold or without scaffold. When the printing is done with scaffold, then the scaffold itself can be pre-printed and thereafter cells can be seeded onto it scaffold and cells can be printed simultaneously, whereas in scaffold free process, direct printing of biomolecules and cells on the substrate (Ong et al., 2017). Table 3 gives a summary of various biomaterials that are used as bioink for the printing of cardiac tissue with the outcomes and compatibility with the native tissue’s along with the process involved in their bioprinting.
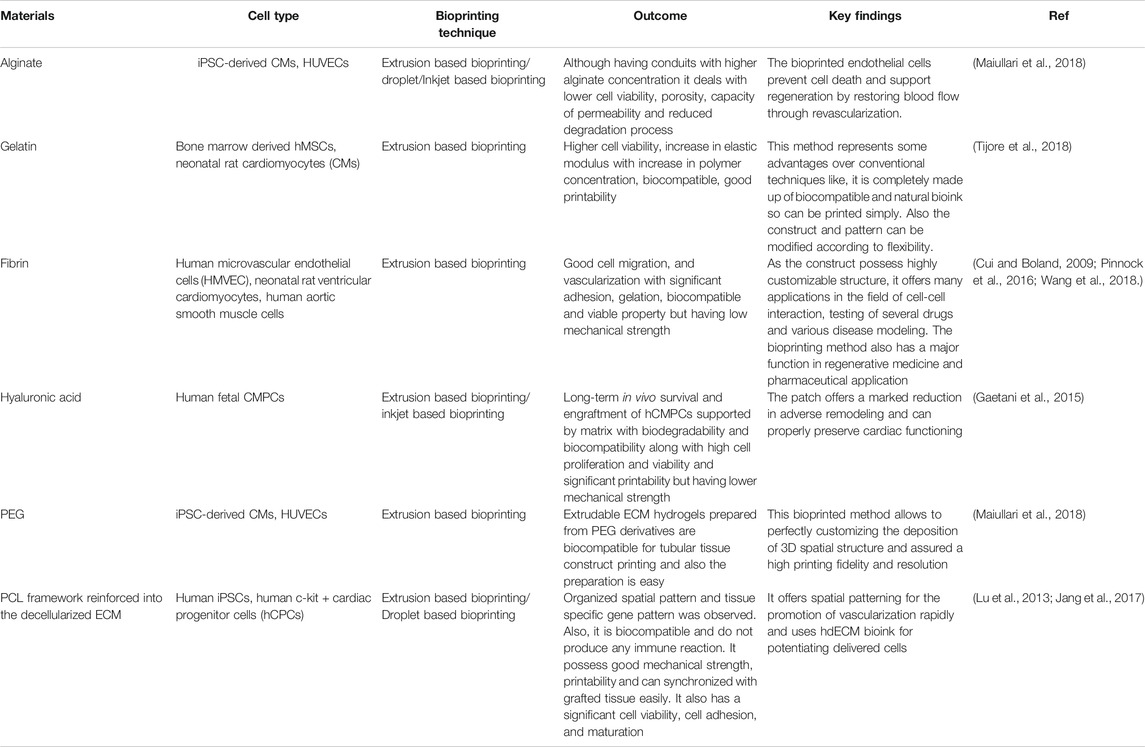
TABLE 3. Comparative summary of different 3D bioprinting techniques used in cardiac tissue regeneration.
3D Bioprinting of Cartilage Tissue
Articular cartilage, a unique smooth and white tissue that covers the end of the bones, has a complex structure made up of several biomolecules like collagen, proteoglycans and non-collagenase proteins. The structure is fabricated by chondrocytes embedded in an extracellular matrix. Though it can tolerate high amount of intensive and repetitive physical stress and often lasts lifetime, it can be degraded by a variety of mechanical, chemical and microbiological agents, leads to several disabling injuries. But due to avascular nature of the cartilage tissue as well as lacking of lymphatic and nervous system in it, any injury caused by trauma or excessive stress cannot be regenerated and finally results several degenerative diseases, like-Osteoarthritis (OA) and ultimately reduces the quality of life (Daly et al., 2017). Techniques already available to combat this disease are- microfracture, mosaicplasty, osteochondral grafts, autologous implantation as well as some therapies based on chondrocyte like MACI (autologous chondrocytes cultured on porcine collagen membrane) (Singh et al., 2019). But some drawbacks related with these techniques are–they provide short-term clinical solutions and often results poor performing cartilage. Another point of consideration is conventional tissue engineering typically aims to homogeneously distribute the biological factors across the tissue whereas the cartilage is composed of three zones i) superficial, ii) middle, and iii) deep zones that differ by gradients of two components, collagen and proteoglycan and arrangements of collagen fibers as depicted in Figure 7 (Daly et al., 2017). As a result, there has been significant motivation to the development of a potential alternative method. By depositing chondrocytes and polyethylene glycol diacrylate, bioprinting technology enables generation of spatial patterns and different grades of cartilage tissue in a controlled manner that imitates the different parts of cartilage anatomy, though the different regions of articular cartilage consists different cell densities, morphologies, mucopolysaccharides composition and mechanical properties (Wang, 2019).
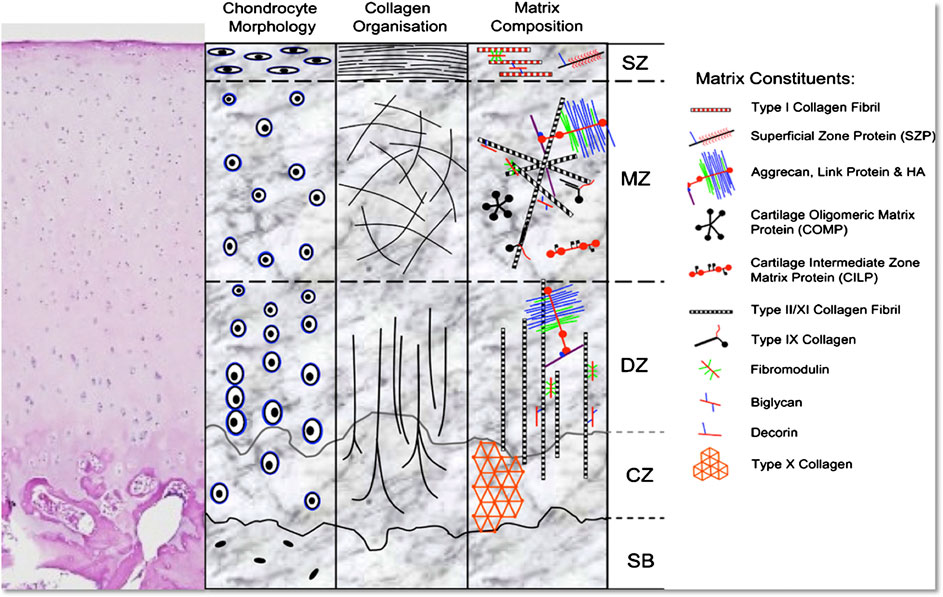
FIGURE 7. Representation of the morphology and structure of H&E stained hyaline cartilage schematically (SB, subchondral bone; CZ, calcified zone; DZ, deep zone; MZ, middle zone; SZ, superficial zone) (Di Bella et al., 2015) (used from an open access journal).
Steps Involved in 3D Bioprinting of Cartilage Tissue
3D-bioprinting of cartilage tissue involves six important steps including i) Imaging analysis, ii) Replacement tissue designing, iii) Preparation of material, iv) Preparation of cell, v) Bioprinting, vi) Implantation. The primary step in 3D cartilage Bioprinting is medical image generation by extracting target patient-specific structure through clinical imaging techniques like MRI/CT scans, etc., sorted and digitalized by computer graphics software like CAD/CAM. Drilling and sawing at a patient-specific manner help orthopedic personnel to the pedicle screws placements and replacement of total joint, respectively (Mouser et al., 2017). Usually, the 3D images sent for printing are divided into- horizontal and vertical slices. The image segmentation process starts from the gray scale appointing followed by indicating one or more starting point which are curved surface from where algorithm will begin and finally the optical format is transformed for subsequent 3D printing. Reduction of additional noise from original image is the main aim of this step. Next step generates the cross-sectional images that change synthetic image from an analytical anatomy. The design of 3D bio printing must contain properties like a) biomaterial should have optimal properties expresses the capability to support manufacturing hardness, known as printability, b) biomaterial should have, internal and external structures, wetting and swelling property, degradation kinetics, ranges from nano to micro scale and stability of structures, and c) development and remodeling of tissues must be considered in long term laboratory method. In the material preparation step, encapsulation of chondrocytes and stem cells into the alginate hydrogels, can retain viability and metabolic activity of the cells. Hydrogels should made including synthetic biomaterials like PGA, PCL, and HA to make sure a uniform 3D structure. The cells required to ECM support synthesis and enable functioning as well as implicated in the vascularization and generation of nervous system or proliferation and differentiation of stem cell niche, can range from 1 × 106 to 1 × 108 cells/ml. They should be enough robust to survive during printing process as well as having ability to maintain cellular function. Prolonged expansion causes dedifferentiation of cells that ultimately leads to loss of cell function. Popular techniques used for cartilage bioprinting involves- Droplet -based, Extrusion -based and stereolithography. These 3D architectures are Implanted after the total development of 3D anatomy by following these steps- immune acceptance, efficacy, safety and integrity function monitoring (Lim et al., 2019).
Bioink for 3D Bioprinting of Cartilage Tissue
Selection of suitable bioink on the basis of composition and mechanical properties is of greatest importance for the viable cartilage substitutes development. These are being formulated from various natural polymers such as collagen, fibrin etc. along with artificial polymers like, polyethylene glycol (PEG), etc. (Chameettachal et al., 2019). One of the main cartilage component hyaluronic acid can be co-printed with poly-lactic acid (PLA) to formulate a novel bioink for cartilage bioprinting (Antich et al., 2020). Sometimes cross-linker free bioink are made by utilizing self-gelling property of silk fibroin along-with gelatin as bulking agent (Singh et al., 2019). Table 4 describes a summary of cartilage bioprinting studies with the preferred bio-ink and the outcome and applications of various bioprinting techniques with regards to cardiovascular tissue.
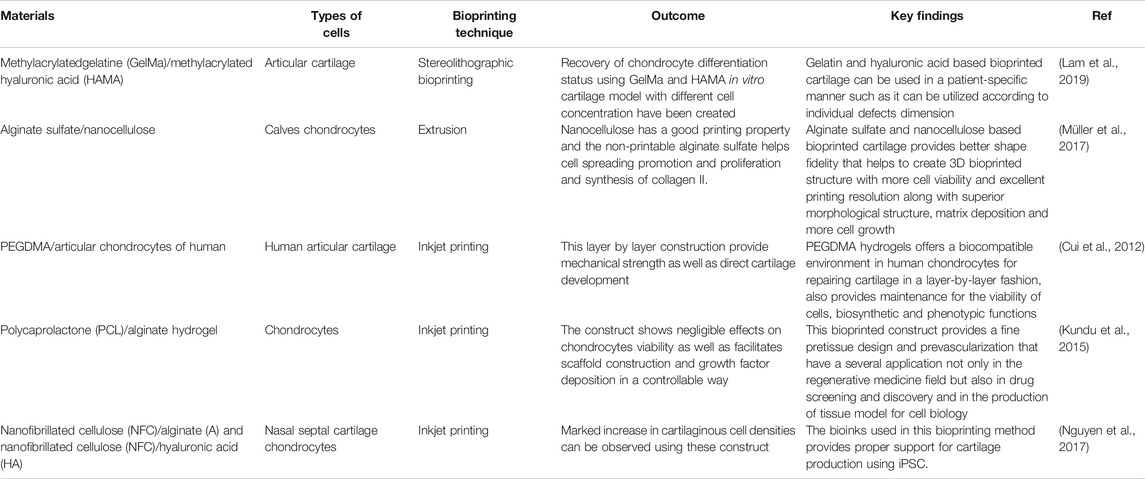
TABLE 4. Comparative summary of different 3D bioprinting techniques used in cartilage tissue regeneration.
3D Bioprinting of Bone Tissue
Bone is structurally complex, highly vascularized tissue in nature and is composed of a ceramic phase in a gel like matrix of protein and polysaccharides as described in Figure 8 (Blausen.com Staff 2014; Gong et al., 2015). Several bone fractures and osteo-degenerative diseases caused by incidences of trauma or any injuries related to diseases or dysfunctional tissue can direct to chronic bone defects that necessitates regeneration of bones that can restore those damaged tissue. Available techniques have some limitations in mimicking bone hierarchical anatomy and construction at a large human scale. They lack cross talking in 3D niche among different cell types (osteoblasts, osteoclasts and endothelial cells) and the tissue construct formed can be inconsistent (Bodhak et al., 2018). Hydrogels used for bone Tissue Engineering are incapable to form mineralized matrix. To overcome these problems, 3D bioprinting, used for bone TE, possesses several benefits, over conventional TE methods, such as they provide enough mechanical backing during regeneration of injured tissues. Other advantages include maintenance of shape and chemistry in a controllable manner with interconnected porosity. These Tissue Engineering constructs have no risk of tissue rejection or disease transfer as there is no need of obtaining tissue from donors or other parts of the body. Another added benefit of using 3D printing is that they utilize anatomically precise models of patient specific data acquired via clinical imaging by means of computer graphics like CAD/CAM for mimicking the complex bone morphology (Midha et al., 2019).
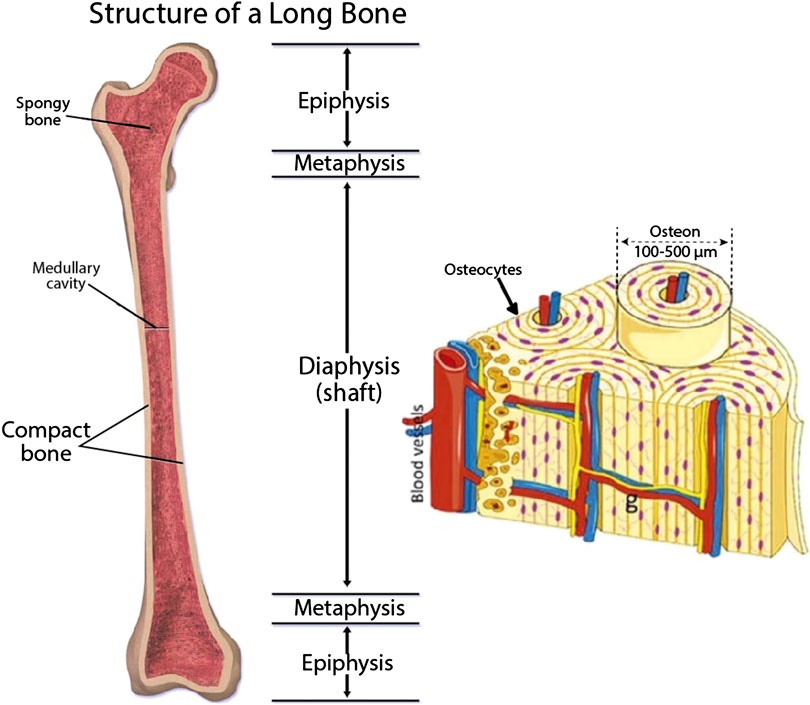
FIGURE 8. Structure and components of bone (Blausen.com Staff 2014; Gong et al., 2015) (used from an open access journal).
Steps Involved in 3D Bioprinting of Bone Tissue
Bone bioprinting involves three successive phases viz., I) pre-processing, II) processing and III) post-processing phase. In first (pre-processing) phase, all of the requirements related to bio printed tissue formation will be planned. Anatomical structure analysis of targeted tissue following CAD for translating images into blue print of 3D bio printed tissue by using CT, MRI etc. data. Additional special programs related to software, e.g., AutoCAD, SOLIDWORKS and CATIA are utilized to make the 3D bio printed tissue model in a layered fashion. Main construction is utilized in the next in processing phase by utilizing bioink composed of various growth factors, some cellular materials (ESCs, MSCs, iPSCs, etc.) and many cellular or synthetic materials can act as scaffold. The final step is the post-processing step that occurred in a bioreactor for full maturation and to make ready to be utilized appendage in vivo (Bishop et al., 2017). Another process of Bone bioprinting is described to be done via a two-steps process that include, synthesis of methacrylatedethanolamide, a gelatin (GE-MA) derivative, at first step followed by photochemical co-crosslinking by methacrylated hyaluronic acid (HA-MA)that produces fluid having gel-like consistency, which is biocompatible enough to support cell adhesion and proliferation (Skardal et al., 2010).
Bioink for 3D Bioprinting of Bone Tissue
Selection of Bio ink is crucial as it should be selected for specific cell types as well as for cytocompatibility and bio functionality. Many natural as well as synthetic materials have been utilized for bioink preparation in bone bioprinting. One of the studies describes gelatin based ink preparation cross-linked through Hydroxyapatite that can imitate the natural bone composition by significantly increasing the viscosity of the bioink. One of the issues with synthetic Hydroxyapatite is that it has comparatively lower osteogenic activity than living tissue (Bodhak et al., 2009). Functionality of hydroxyapatite can be increased to achieve higher osteointegration by doping it with ions such as Magnesium and Strontium (Bodhak et al., 2011a; Bodhak et al., 2011b; Bodhak et al., 2013). Hydroxyapatite can be mixed with another biodegradable polyester Polycaprolactone having higher mechanical properties combined with alginate. Another method of Bioink formulation can be done using sodium alginate and poloxamer to meet enhanced mechanical and rheological properties. One more study of bioink preparation was done by incorporating polycaprolactone microfibers and nanofibers, incorporated with collagen and alginate for fabrication of functional 3D construct for MSC proliferation and differentiation (Jang et al., 2016). Table 5 describes a summary of bone bioprinting studies with special attention to the bio-inks and their application with regards to printing of bone tissue.
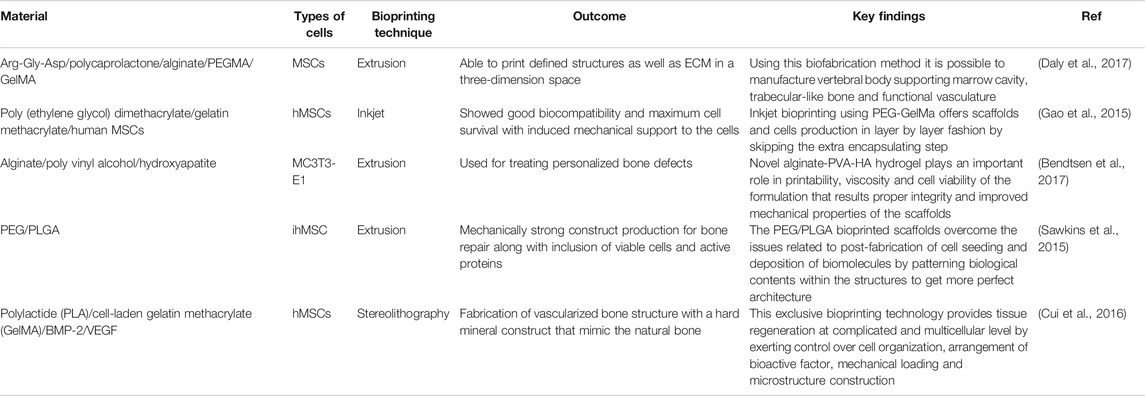
TABLE 5. Comparative summary of different 3D bioprinting techniques used in bone tissue regeneration.
Current Challenges and Future Aspects of 3D Bioprinting Techniques
FDA has issued a guidance document for production of medical devices, “Technical considerations for Additive Manufactured Devices” that provides guidelines for the additive manufacturing including 3D printing. With the technological advancement in the printing technique and development of efficient and cost-effective printing methods, it becomes necessary to regulate the quality control standard before transplantation in each step during the process, such as while designing a model, selection of bioink, printing validation, maturation of post-printing and assessment of product quality (Balla et al., 2020). Further, the number of components involved in printing process is one of the big issues with 3D bioprinting. Lack of software that can define the placements of cells, biomaterials and biological molecules virtually following the robust designing and translation that drive downstream manufacturing operations cause bioprinting to be hampered (Starly and Shirwaiker, 2015). Another challenge is, it is necessary to manufacture an adequately stable as well as mechanically inflexible 3D construct during transplantation. During hard tissue repairing, porosity and structure designed by 3D bioprinting should maintain a high elastic modulus so that they can support the natural cell growth during implantation (Hollinger et al., 1996). Due to scaffold deformation newly formed tissues will probably fail if proper structural maintenance and mechanical support are not given by the scaffold (Hollister, 2005). Proper vascularization in vivo is another important need for a bio printed construct in TE that provides the cells’ growth factors, oxygen, nutrients and removes waste. In vivo capillaries, which are present within 100 mm from maximum cells, exhibit sufficient diffusion, that is needed for survival of the cell (Kaully et al., 2009). Some of the issues that arise with the scalability and wide-spread adoption of bioprinting techniques have been highlighted here in Figure 9.
Today organ transplantation could be a lifesaving treatment choice but few people are available as donors. According to Organdonor.gov, 18 people die in the US everyday due to appropriate organ transplant. Therefore, this emerging 3D bioprinting technology could be an option for organ transplantations around the world and could end the heavy demand on organs. Future developments in bioprinting is expected to witness rapid developments in bioprinters which can be readily deployed in hospitals. The bioprinters will be expected to perform bioprinting with high resolution, mechanical strengths and cell viability. In addition, to obtain bioprinted constructs for clinical translation, it is necessary to integrate functional vasculature in the grafts to ensure long term cell survival (Datta et al., 2018). Different tissues of the body have differing requirements of cell densities, number of different types of cells, spati-temporal distribution of cells inside the constructs. Moreover, when using stem cells, different matrix properties may modulate the differentiation and trans-differentiation of cells into specific lineages (Even-Ram et al., 2006; Barui et al., 2018). Bioprinting is multi-step process and each step should be well-coordinated with other steps in the process. Perfusion bioreactors are expected to be another key area where bioprinting technologies will witness increased integration. However, apart from all the aboe future directions, most important would be rapid envelopment of bioinks with optimized bioprintability and biofunctional properties. At present, most biopolymers used in bioprinting are borrowed from polymers generally used for tissue engineering and seldom possess optimal rheological and crosslinking properties ideal for a bioprinting process. Therefore, there exists a significant challenges of developing ideal bioinks (Skardal, 2018). Finally, since the ultimate aim of bioprinting is to provide functional tissue constructs there is also need to develop better assays, which can analyze cell functionality in 3D constructs. given the rapid pace at which bioprinting is emerging and the tremendous interest in this technology cutting across different scientific disciplines, it is expected that the above challenges could be overcome and bioprinted constructs will become available for translational studies as well as speed up the drug development process especially considering the fact that Pharmaceutical companies will spend over $50 billion dollars on research and development to get drug approval from the FDA for animal, preclinical, and clinical testing. It is expected that in the future, 3D Bio bioprinters can cheapen this expense and quicken testing time with better prediction of drug reaction and without waste money or time. Figure 10 provides a comprehensive picture of the current applications in bioprinting with some of the approved research going on in this field.
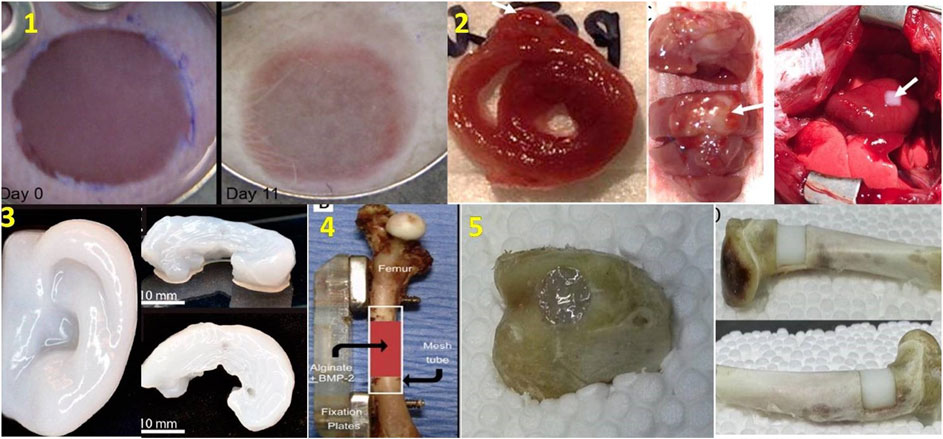
FIGURE 10. Different bioprinted organs. 1) Skin construct includes 20 layers of keratinocytes and fibroblasts implanted into the wound Day 0 (at left) and Day 11 (at right) (He et al., 2018), 2) Cross-section of 3D bioprinted cardiac patches (at left), anterior aspect (at middle), In vivo transplantation of cardiac patches (at right) (Ong et al.,2017) 3) 3D bioprinted human ear (at left) and sheep meniscus (at right) (Mori et al., 2018), 4) Treatment of femur defect using polymeric hydrogel and growth factor (Mori et al., 2018) 5) Fixing of bone defects using in situ 3D bioprinting with alginate hydrogel, transparent before photopolymerization (at left), becomes milky white after photopolymerization (at right) (Li et al., 2017) (used from open access journals).
Conclusions
Additive manufacturing in the context of bioprinting offers a huge potential in the field of tissue and organ regeneration. It enables the fabrication of physiologically-relevant tissue with better and consistent functional outcomes in patients. Such techniques are advantageous over autografting or allografting considering autologous grafts cause unnecessary stress on the patient and there is an acute shortage of allograft donors. 3D bioprinting presents a unique opportunity in that it builds the tissue from bottom up and as such the risk of immunological graft rejection is not present all the while mitigating the issues related to donor scarcity. The use of 3D bioprinting could potentially lead to a personalized treatment for the patient which translates to better clinical outcomes as well as is aesthetically pleasing. However, despite all the advances that have been made in the field, there are still many challenges with regards to the biocompatibility and integration of the printed construct with the body. Maintenance of cell viability in the bio-ink formulation and then printing them in precise geometries requires standardization of the printing methods and meticulous quality-control to maintain the quality of the printed construct. To keep up with the demands of tissue engineering field, the technique for 3D bioprinting has evolved to present multiple approaches for tissue fabrication such as inkjet printing, laser assisted bioprinting, extrusion bioprinting, stereolithography, etc. Overall, 3D bioprinting techniques offer viable and high-throughput tissue printing with better spatial control and precise patterning of cells when compared to manual methods of tissue culture.
Author Contributions
The article was written, edited, and approved by SA, SS, VB, AP, AB, and SB.
Conflict of Interest
The authors declare that the research was conducted in the absence of any commercial or financial relationships that could be construed as a potential conflict of interest
Funding
The authors would like to acknowledge the Ramalingaswami Re-Entry Fellowship, Department of Biotechnology (DBT), Government of India (BT/RLF/Re-entry/13/2016) and Science and Engineering Research Board (SERB), Department of Science and Technology, Government of India (PDF/2018/000182) for financial support.
References
Alamán, J., Alicante, R., Peña, J., and Sánchez-Somolinos, C. (2016). Inkjet printing of functional materials for optical and photonic applications. Materials 9, 910. doi:10.3390/ma9110910
Antich, C., de Vicente, J., Jiménez, G., Chocarro, C., Carrillo, E., Montañez, E., et al. (2020). Bio-inspired hydrogel composed of hyaluronic acid and alginate as a potential bioink for 3D bioprinting of articular cartilage engineering constructs. Acta Biomater. 106, 114–123. doi:10.1016/j.actbio.2020.01.046
Arslan-Yildiz, A., El Assal, R., Chen, P., Guven, S., Inci, F., and Demirci, U. (2016). Towards artificial tissue models: past, present, and future of 3D bioprinting. Biofabrication 8 (1), 014103. doi:10.1088/1758-5090/8/1/014103
Bártolo, P. J. (2011). “Stereolithographic processes,” in Stereolithography (Boston, MA: Springer), 1–36.
Bücking, T. M., Hill, E. R., Robertson, J. L., Maneas, E., Plumb, A. A., and Nikitichev, D. I. (2017). From medical imaging data to 3D printed anatomical models. PLos One 12 (5), e0178540. doi:10.1371/journal.pone.0178540
Balla, V. K., Bodhak, S., Datta, P., Kundu, B., Das, M., Bandyopadhyay, A., et al. (2020). “Biointegration of three-dimensional–printed biomaterials and biomedical devices,” in Biointegration of medical implant materials. Editor C. P. Sharma (Netherlands: Elsevier), 433–482.
Bandyopadhyay, A., Zhang, Y., and Bose, S. (2020). Recent developments in metal additive manufacturing. Curr. Opin. Chem. Eng. 28, 96–104. doi:10.1016/j.coche.2020.03.001
Barron, J. A., Krizman, D. B., and Ringeisen, B. R. (2005). Laser printing of single cells: statistical analysis, cell viability, and stress. Ann. Biomed. Eng. 33 (2), 121–130. doi:10.1007/s10439-005-8971-x
Barron, J. A., Ringeisen, B. R., Kim, H., Spargo, B. J., and Chrisey, D. B. (2004). Application of laser printing to mammalian cells. Thin Solid Films 453–454, 383–387. doi:10.1016/j.tsf.2003.11.161
Barui, A., Chowdhury, F., Pandit, A., and Datta, P. (2018). Rerouting mesenchymal stem cell trajectory towards epithelial lineage by engineering cellular niche. Biomaterials 156, 28–44. doi:10.1016/j.biomaterials.2017.11.036
Bendtsen, S. T., Quinnell, S. T., and Wei, M. (2017). Development of a novel alginate-polyvinyl alcohol-hydroxyapatite hydrogel for 3D bioprinting bone tissue engineered scaffolds. J. Biomed. Mater. Res. 105 (5), 1457–1468. doi:10.1002/jbm.a.36036
Bishop, E. S., Mostafa, S., Pakvasa, M., Luu, H. H., Lee, M. J., Wolf, J. M., et al. (2017). 3-D bioprinting technologies in tissue engineering and regenerative medicine: current and future trends. Genes Dis. 4 (4), 185–195. doi:10.1016/j.gendis.2017.10.002
Bose, S., and Bandyopadhyay, A. (2019). “Additive manufacturing: the future of manufacturing in a flat world,” in Additive manufacturing, 2nd Edn (Boca Raton, FL: CRC Press), 451–461.
Bodhak, S., Bose, S., and Bandyopadhyay, A. (2009). Role of surface charge and wettability on early stage mineralization and bone cell-materials interactions of polarized hydroxyapatite. Acta Biomater. 5 (6), 2178–2188. doi:10.1016/j.actbio.2009.02.023
Bodhak, S., Bose, S., and Bandyopadhyay, A. (2010). Electrically polarized HAp-coated Ti: in vitro bone cell-material interactions. Acta Biomater. 6 (2), 641–651. doi:10.1016/j.actbio.2009.08.008
Bodhak, S., Bose, S., and Bandyopadhyay, A. (2011a). Bone cell-material interactions on metal-ion doped polarized hydroxyapatite. Mater. Sci. Eng. C 31 (4), 755–761. doi:10.1016/j.msec.2011.01.003
Bodhak, S., Bose, S., and Bandyopadhyay, A. (2011b). Influence of MgO, SrO, and ZnO dopants on electro-thermal polarization behavior and in vitro biological properties of hydroxyapatite ceramics. J. Am. Ceram. Soc. 94 (4), 1281–1288. doi:10.1111/j.1551-2916.2010.04228.x
Bodhak, S., de Castro, L. F., Kuznetsov, S. A., Azusa, M., Bonfim, D., Robey, P. G., et al. (2018). Combinatorial cassettes to systematically evaluate tissue-engineered constructs in recipient mice. Biomaterials 186, 31–43. doi:10.1016/j.biomaterials.2018.09.035
Bodhak, S., Kikuchi, M., Sogo, Y., Tsurushima, H., Ito, A., and Oyane, A. (2013). Calcium phosphate coating on a bioresorbable hydroxyapatite/collagen nanocomposite for surface functionalization. Chem. Lett. 42 (9), 1029–1031. doi:10.1246/cl.130327
Bose, S., Roy, M., and Bandyopadhyay, A. (2012). Recent advances in bone tissue engineering scaffolds. Trends Biotechnol. 30 (10), 546–554. doi:10.1016/j.tibtech.2012.07.005
Bose, S., Vahabzadeh, S., and Bandyopadhyay, A. (2013). Bone tissue engineering using 3D printing. Mater. Today 16 (12), 496–504. doi:10.1016/j.mattod.2013.11.017
Brown, T. M., and Krishnamurthy, K. (2018). “Histology, dermis,” in StatPearls [Internet] (Treasure Island, FL: StatPearls Publishing).
Bursac, N. (2009). Cardiac tissue engineering using stem cells. IEEE Eng. Med. Biol. Mag. 28 (2), 80–89. doi:10.1109/memb.2009.931792
Catros, S., Keriquel, V., Fricain, J. C., and Guillemot, F. (2015). “In vivo and in situ biofabrication by laser-assisted bioprinting,” in Essentials of 3D biofabrication and translation (Cambridge, MA: Academic Press), 81–87.
Chameettachal, S., Sasikumar, S., Sethi, S., Sriya, Y., and Pati, F. (2019). Tissue/organ-derived bioink formulation for 3D bioprinting. J. 3D Print. Med. 3 (1), 39–54. doi:10.2217/3dp-2018-0024
Chan, V., Jeong, J. H., Bajaj, P., Collens, M., Saif, T., Kong, H., et al. (2012a). Multi-material bio-fabrication of hydrogel cantilevers and actuators with stereolithography. Lab Chip 12 (1), 88–98. doi:10.1039/c1lc20688e
Chan, V., Park, K., Collens, M. B., Kong, H., Saif, T. A., and Bashir, R. (2012b). Development of miniaturized walking biological machines. Sci. Rep. 2, 857. doi:10.1038/srep00857
Chu, T.-M. G., Orton, D. G., Hollister, S. J., Feinberg, S. E., and Halloran, J. W. (2002). Mechanical and in vivo performance of hydroxyapatite implants with controlled architectures. Biomaterials 23 (5), 1283–1293. doi:10.1016/s0142-9612(01)00243-5
Cubo, N., Garcia, M., Del Cañizo, J. F., Velasco, D., and Jorcano, J. L. (2016). 3D bioprinting of functional human skin: production and in vivo analysis. Biofabrication 9 (1), 015006. doi:10.1088/1758-5090/9/1/015006
Cui, H., Zhu, W., Nowicki, M., Zhou, X., Khademhosseini, A., and Zhang, G. (2016). Hierarchical fabrication of engineered vascularized bone biphasic constructs via dual 3D bioprinting: integrating regional bioactive factors into architectural design. Adv. Healthcare Mater. 5 (17), 2174–2181. doi:10.1002/adhm.201600505
Cui, X., Boland, T., D’Lima, D. D., and Lotz, M. (2012). Thermal inkjet printing in tissue engineering and regenerative medicine. Recent Pat. Drug Deliv. Formul. 6 (2), 149–155. doi:10.2174/187221112800672949
Cui, X., and Boland, T. (2009). Human microvasculature fabrication using thermal inkjet printing technology. Biomaterials 30 (31), 6221–6227. doi:10.1016/j.biomaterials.2009.07.056
Cui, X., Dean, D., Ruggeri, Z. M., and Boland, T. (2010). Cell damage evaluation of thermal inkjet printed Chinese hamster ovary cells. Biotechnol. Bioeng. 106 (6), 963–969. doi:10.1002/bit.22762
Dababneh, A. B., and Ozbolat, I. T. (2014). Bioprinting technology: a current state-of-the-art review. J. Manuf. Sci. Eng. 136 (6), 061016. doi:10.1115/1.4028512
Daly, A. C., Freeman, F. E., Gonzalez-Fernandez, T., Critchley, S. E., Nulty, J., and Kelly, D. J. (2017). 3D bioprinting for cartilage and osteochondral tissue engineering. Adv. Healthcare Mater. 6 (22), 1700298. doi:10.1002/adhm.201700298
Datta, P., Barui, A., Wu, Y., Ozbolat, V., Moncal, K. K., and Ozbolat, I. T. (2018). Essential steps in bioprinting: from pre- to post-bioprinting. Biotechnol. Adv. 36 (5), 1481–1504. doi:10.1016/j.biotechadv.2018.06.003
Dean, D., Wallace, J., Siblani, A., Wang, M. O., Kim, K., Mikos, A. G., et al. (2012). Continuous digital light processing (cDLP): highly accurate additive manufacturing of tissue engineered bone scaffolds. Virtual Phys. Prototyp. 7 (1), 13–24. doi:10.1080/17452759.2012.673152
Delaney, J. T., Smith, P. J., and Schubert, U. S. (2009). Inkjet printing of proteins. Soft Matter 5 (24), 4866–4877. doi:10.1039/b909878j
Derakhshanfar, S., Mbeleck, R., Xu, K., Zhang, X., Zhong, W., and Xing, M. (2018). 3D bioprinting for biomedical devices and tissue engineering: a review of recent trends and advances. Bioactive Materials 3 (2), 144–156. doi:10.1016/j.bioactmat.2017.11.008
Derby, B. (2008). Bioprinting: inkjet printing proteins and hybrid cell-containing materials and structures. J. Mater. Chem. 18 (47), 5717–5721. doi:10.1039/b807560c
Di Bella, C., Fosang, A., Donati, D. M., Wallace, G. G., and Choong, P. F. M. (2015). 3D bioprinting of cartilage for orthopedic surgeons: reading between the lines. Front. Surg. 2, 39. doi:10.3389/fsurg.2015.00039
Duocastella, M., Colina, M., Fernández-Pradas, J. M., Serra, P., and Morenza, J. L. (2007). Study of the laser-induced forward transfer of liquids for laser bioprinting. Appl. Surf. Sci. 253 (19), 7855–7859. doi:10.1016/j.apsusc.2007.02.097
Eng, G., Lee, B. W., Protas, L., Gagliardi, M., Brown, K., Kass, R. S., et al. (2016). Autonomous beating rate adaptation in human stem cell-derived cardiomyocytes. Nat. Commun. 7 (1), 10312. doi:10.1038/ncomms10312
Even-Ram, S., Artym, V., and Yamada, K. M. (2006). Matrix control of stem cell fate. Cell 126 (4), 645–647. doi:10.1016/j.cell.2006.08.008
Fedorovich, N. E., Schuurman, W., Wijnberg, H. M., Prins, H.-J., Van Weeren, P. R., Malda, J., et al. (2012). Biofabrication of osteochondral tissue equivalents by printing topologically defined, cell-laden hydrogel scaffolds. Tissue Eng. C Methods 18 (1), 33–44. doi:10.1089/ten.tec.2011.0060
Gaetani, R., Feyen, D. A. M., Verhage, V., Slaats, R., Messina, E., Christman, K. L., et al. , (2015). Epicardial application of cardiac progenitor cells in a 3D-printed gelatin/hyaluronic acid patch preserves cardiac function after myocardial infarction. Biomaterials 61, 339–348. doi:10.1016/j.biomaterials.2015.05.005
Gao, G., Schilling, A. F., Hubbell, K., Yonezawa, T., Truong, D., Hong, Y., et al. (2015) Improved properties of bone and cartilage tissue from 3D inkjet-bioprinted human mesenchymal stem cells by simultaneous deposition and photocrosslinking in PEG-GelMA. Biotechnol. Lett. 37 (11), 2349–2355. doi:10.1007/s10529-015-1921-2
Gong, T., Xie, J., Liao, J., Zhang, T., Lin, S., and Lin, Y. (2015). Nanomaterials and bone regeneration. Bone Res. 3, 15029. doi:10.1038/boneres.2015.29
Guillemot, F., Guillotin, B., Fontaine, A., Ali, M., Catros, S., Kériquel, V., et al. (2011). Laser-assisted bioprinting to deal with tissue complexity in regenerative medicine. MRS Bull. 36 (12), 1015. doi:10.1557/mrs.2011.272
Guillotin, B., Souquet, A., Catros, S., Duocastella, M., Pippenger, B., Bellance, S., et al. (2010). Laser assisted bioprinting of engineered tissue with high cell density and microscale organization. Biomaterials 31 (28), 7250–7256. doi:10.1016/j.biomaterials.2010.05.055
He, P., Zhao, J., Zhang, J., Bo., L., Gou, Z., Gou, M., et al. (2018). Bioprinting of skin construct for wound healing. Burns Trauma 6, 5. doi:10.1186/s41038-017-0104-x
Heller, C., Schwentenwein, M., Russmueller, G., Varga, F., Stampfl, J., and Liska, R. (2009). Vinyl esters: low cytotoxicity monomers for the fabrication of biocompatible 3D scaffolds by lithography based additive manufacturing. J. Polym. Sci. A Polym. Chem. 47 (24), 6941–6954. doi:10.1002/pola.23734
Hinderer, S., Layland, S. L., and Schenke-Layland, K. (2016). ECM and ECM-like materials-biomaterials for applications in regenerative medicine and cancer therapy. Adv. Drug Deliv. Rev. 97, 260–269. doi:10.1016/j.addr.2015.11.019
Hollinger, J. O., Brekke, J., Gruskin, E., and Lee, D. (1996). Role of bone substitutes. Clin. Orthop. Relat. Res. 324, 55–65. doi:10.1097/00003086-199603000-00008
Hollister, S. J., Maddox, R. D., and Taboas, J. M. (2002). Optimal design and fabrication of scaffolds to mimic tissue properties and satisfy biological constraints. Biomaterials 23 (20), 4095–4103. doi:10.1016/s0142-9612(02)00148-5
Hollister, S. J. (2005). Porous scaffold design for tissue engineering. Nat. Mater. 4 (7), 518–524. doi:10.1038/nmat1421
Hopp, B., Smausz, T., Antal, Z., Kresz, N., Bor, Z., and Chrisey, D. (2004). Absorbing film assisted laser induced forward transfer of fungi (Trichoderma conidia). J. Appl. Phys. 96 (6), 3478–3481. doi:10.1063/1.1782275
Hsieh, T. M., Benjamin Ng, C. W., Narayanan, K., Wan, A. C. A., and Ying, J. Y. (2010). Three-dimensional microstructured tissue scaffolds fabricated by two-photon laser scanning photolithography. Biomaterials 31 (30), 7648–7652. doi:10.1016/j.biomaterials.2010.06.029
Jang, C. H., Ahn, S. H., Yang, G.-H., and Kim, G. H. (2016). A MSCs-laden polycaprolactone/collagen scaffold for bone tissue regeneration. RSC Adv. 6 (8), 6259–6265. doi:10.1039/c5ra20627h
Jang, J., Park, H.-J., Kim, S.-W., Kim, H., Park, J. Y., Na, S. J., et al. (2017). 3D printed complex tissue construct using stem cell-laden decellularized extracellular matrix bioinks for cardiac repair. Biomaterials 112, 264–274. doi:10.1016/j.biomaterials.2016.10.026
Jawad, H., Ali, N. N., Lyon, A. R., Chen, Q. Z., Harding, S. E., and Boccaccini, A. R. (2007). Myocardial tissue engineering: a review. J. Tissue Eng. Regen. Med. 1 (5), 327–342. doi:10.1002/term.46
Jeong, J. H., Chan, V., Cha, C., Zorlutuna, P., Dyck, C., Hsia, K. J., et al. (2012), “Living” microvascular stamp for patterning of functional neovessels; orchestrated control of matrix property and geometry. Adv. Mater. 24, 58–63. doi:10.1002/adma.201103207
Jones, N. (2012). Science in three dimensions: the print revolution. Nature 487 (7405), 22–23. doi:10.1038/487022a
Kačarević, Ž. P., Rider, P. M., Alkildani, S., Retnasingh, S., Smeets, R., Jung, O., et al. (2018). An introduction to 3D bioprinting: possibilities, challenges and future aspects. Materials, 11 (11), 2199. doi:10.3390/ma11112199
Kaully, T., Kaufman-Francis, K., Lesman, A., and Levenberg, S. (2009). Vascularization-the conduit to viable engineered tissues. Tissue Eng. B Rev. 15 (2), 159–169. doi:10.1089/ten.teb.2008.0193
Keriquel, V., Oliveira, H., Rémy, M., Ziane, S., Delmond, S., Rousseau, B., et al. (2017). In situ printing of mesenchymal stromal cells, by laser-assisted bioprinting, for in vivo bone regeneration applications. Sci. Rep. 7 (1), 1778. doi:10.1038/s41598-017-01914-x
Khoshnood, N., and Zamaniain, A. (2020). A comprehensive review on scaffold-free bioinks for bioprinting. Bioprinting 19, e00088. doi:10.1016/j.bprint.2020.e00088
Kim, B. S., Lee, J. S., Gao, G., and Cho, D. W. (2017). Direct 3D cell-printing of human skin with functional transwell system. Biofabrication 9 (2), 025034. doi:10.1088/1758-5090/aa71c8
Knowlton, S., Anand, S., Shah, T., and Tasoglu, S. (2018). Bioprinting for neural tissue engineering. Trends Neurosci. 41 (1), 31–46. doi:10.1016/j.tins.2017.11.001
Koch, L., Deiwick, A., Schlie, S., Michael, S., Gruene, M., Coger, V., et al. (2012). Skin tissue generation by laser cell printing. Biotechnol. Bioeng. 109 (7), 1855–1863. doi:10.1002/bit.24455
Koch, L., Kuhn, S., Sorg, H., Gruene, M., Schlie, S., Gaebel, R., et al. (2010). Laser printing of skin cells and human stem cells. Tissue Eng. C Methods 16 (5), 847–854. doi:10.1089/ten.tec.2009.0397
Kundu, J., Shim, J.-H., Jang, J., Kim, S.-W., and Cho, D.-W. (2015) An additive manufacturing-based PCL-alginate-chondrocyte bioprinted scaffold for cartilage tissue engineering. J. Tissue Eng. Regen. Med. 9, 1286–1297. doi:10.1002/term.1682
Lam, T., Dehne, T., Krüger, J. P., Hondke, S., Endres, M., Thomas, A., et al. (2019). Photopolymerizable gelatin and hyaluronic acid for stereolithographic 3D bioprinting of tissue‐engineered cartilage. J. Biomed. Mater. Res. B Appl. Biomater. 107, 2649–2657. doi:10.1002/jbm.b.34354
Lee, V., Singh, G., Trasatti, J. P., Bjornsson, C., Xu, X., Tran, T. N., et al. (2014). Design and fabrication of human skin by three-dimensional bioprinting. Tissue Eng. C Methods, 20 (6), 473–484. doi:10.1089/ten.tec.2013.0335
Leoni, D., and Rello, J. (2017). Cardiac arrest among patients with infections: causes, clinical practice and research implications. Clin. Microbiol. Infect., 23 (10), 730–735. doi:10.1016/j.cmi.2016.11.018
Lewis, J. A., and Gratson, G. M. (2004). Direct writing in three dimensions. Mater. Today 7 (7–8), 32–39. doi:10.1016/s1369-7021(04)00344-x
Li, L., Yui, F., Shi, J., Shen, S., Teng, H., Yang, J., et al. (2017). In situ repair of bone and cartilage defects using 3D scanning and 3D printing. Sci. Rep. 7, 9416. doi:10.1038/s41598-017-10060-3
Li, S., Xiong, Z., Wang, X., Yan, Y., Liu, H., and Zhang, R. (2009). Direct fabrication of a hybrid cell/hydrogel construct by a double-nozzle assembling technology. J. Bioact. Compat. Polym. 24 (3), 249–265. doi:10.1177/0883911509103357
Lim, W., Kim, B., and Moon, Y. L. (2019). Three-dimensional bioprinting for bone and cartilage transplantation. Ann. Joint 4 (1), 7. doi:10.21037/aoj.2018.12.06
Lu, T. Y., Lin, B., Kim, J., Sullivan, M., Tobita, K., Salama, G., and Yang, L. (2013). Repopulation of decellularized mouse heart with human induced pluripotent stem cell-derived cardiovascular progenitor cells. Nat. Commun. 4 (1), 2307. doi:10.1038/ncomms3307
Müller, M., Öztürk, E., Arlov, Ø., Gatenholm, P., and Zenobi-Wong, M. (2017). Alginate sulfate-nanocellulose bioinks for cartilage bioprinting applications. Ann. Biomed. Eng. 45 (1), 210–223. doi:10.1007/s10439-016-1704-5
Maiullari, F., Costantini, M., Milan, M., Pace, V., Chirivì, M., Maiullari, S., et al. (2018). A multi-cellular 3D bioprinting approach for vascularized heart tissue engineering based on HUVECs and iPSC-derived cardiomyocytes. Sci. Rep. 8 (1), 13532. doi:10.1038/s41598-018-31848-x
Mandrycky, C., Wang, Z., Kim, K., and Kim, D.-H. (2016). 3D bioprinting for engineering complex tissues. Biotechnol. Adv. 34 (4), 422–434. doi:10.1016/j.biotechadv.2015.12.011
Marchioli, G., van Gurp, L., Van Krieken, P. P., Stamatialis, D., Engelse, M., Van Blitterswijk, C. A., et al. (2015). Fabrication of three-dimensional bioplotted hydrogel scaffolds for islets of Langerhans transplantation. Biofabrication 7 (2), 025009. doi:10.1088/1758-5090/7/2/025009
Melchels, F. P. W., Domingos, M. A. N., Klein, T. J., Malda, J., Bartolo, P. J., and Hutmacher, D. W. (2012). Additive manufacturing of tissues and organs. Prog. Polym. Sci. 37 (8), 1079–1104. doi:10.1016/j.progpolymsci.2011.11.007
Midha, S., Dalela, M., Sybil, D., Patra, P., and Mohanty, S. (2019). Advances in three‐dimensional bioprinting of bone: progress and challenges. J. Tissue Eng. Regen. Med. 13 (6), 925–945.
Miri, A. K., Nieto, D., Iglesias, L., Goodarzi Hosseinabadi, H., Maharjan, S., Ruiz-Esparza, G. U., et al. 2018). Microfluidics-enabled multimaterial maskless stereolithographic bioprinting. Adv. Mater. 30 (27), 1800242. doi:10.1002/adma.201800242
Mori, A. D., Fernandez, M. P., Blunn, G., Tozzi, G., and Roldo, M. (2018). 3D printing and electrospinning of composite hydrogels for cartilage and bone tissue engineering. Polymers 10, 285.
Morris, V. B., Nimbalkar, S., Younesi, M., McClellan, P., and Akkus, O. (2017). Mechanical properties, cytocompatibility and manufacturability of chitosan:PEGDA hybrid-gel scaffolds by stereolithography. Ann. Biomed. Eng. 45 (1), 286–296. doi:10.1007/s10439-016-1643-1
Mouser, V. H., Levato, R., Bonassar, L. J., D’Lima, D. D., Grande, D. A., Klein, T. J., et al. (2017). Three-dimensional bioprinting and its potential in the field of articular cartilage regeneration. Cartilage 8 (4), 327–340. doi:10.1177/1947603516665445
Murphy, S. V., and Atala, A. (2014). 3D bioprinting of tissues and organs. Nat. Biotechnol. 32 (8), 773–785. doi:10.1038/nbt.2958
Nahmias, Y., Schwartz, R. E., Verfaillie, C. M., and Odde, D. J. (2005). Laser-guided direct writing for three-dimensional tissue engineering. Biotechnol. Bioeng. 92 (2), 129–136. doi:10.1002/bit.20585
Naumann, A., Aigner, J., Staudenmaier, R., Seemann, M., Bruening, R., Englmeier, K. H., et al. , 2003. Clinical aspects and strategy for biomaterial engineering of an auricle based on three-dimensional stereolithography. Eur. Arch. Otorhinolaryngol. 260 (10), 568–575. doi:10.1007/s00405-003-0636-5
Nguyen, D., Hägg, D. A., Forsman, A., Ekholm, J., Nimkingratana, P., Brantsing, C., et al. , 2017.Cartilage tissue engineering by the 3D bioprinting of iPS cells in a nanocellulose/alginate bioink. Sci. Rep. 7, 658. doi:10.1038/s41598-017-00690-y
Noecker, A. M., Chen, J.-F., Zhou, Q., White, R. D., Kopcak, M. W., Arruda, M. J., et al. (2006). Development of patient-specific three-dimensional pediatric cardiac models. Am. Soc. Artif. Intern. Organs J. 52 (3), 349–353. doi:10.1097/01.mat.0000217962.98619.ab
O’Bryan, C. S., Bhattacharjee, T., Niemi, S. R., Balachandar, S., Baldwin, N., Ellison, S. T., et al. (2017). Three-dimensional printing with sacrificial materials for soft matter manufacturing. MRS Bull. 42 (8), 571–577.
Okamoto, T., Suzuki, T., and Yamamoto, N. (2000). Microarray fabrication with covalent attachment of DNA using bubble jet technology. Nat. Biotechnol. 18 (4), 438–441. doi:10.1038/74507
Ong, C. S., Fukunishi, T., Zhang, H., Huang, C. Y., Nashed, A., Blazeski, A., et al. (2017). Biomaterial-free three-dimensional bioprinting of cardiac tissue using human induced pluripotent stem cell derived cardiomyocytes. Sci. Rep. 7 (1), 4566. doi:10.1038/s41598-017-05018-4
Ozbolat, I. T. (2015a). Scaffold-based or scaffold-free bioprinting: competing or complementing approaches? J. Nanotechnol. Eng. Med. 6 (2), 024701. doi:10.1115/1.4030414
Ozbolat, I. T. (2015b). Bioprinting scale-up tissue and organ constructs for transplantation. Trends Biotechnol. 33 (7), 395–400. doi:10.1016/j.tibtech.2015.04.005
Papaioannou, T. G., Manolesou, D., Dimakakos, E., Tsoucalas, G., Vavuranakis, M., and Tousoulis, D. (2019). 3D bioprinting methods and techniques: applications on artificial blood vessel fabrication. Acta Cardiol. Sin. 35 (3), 284–289. doi:10.6515/ACS.201905_35(3).20181115A
Parak, A., Pradeep, P., du Toit, L. C., Kumar, P., Choonara, Y. E., and Pillay, V. (2019). Functionalizing bioinks for 3D bioprinting applications. Drug Discov. Today 24 (1), 198–205. doi:10.1016/j.drudis.2018.09.012
Park, S. A., Lee, S. H., and Kim, W. D. (2011). Fabrication of porous polycaprolactone/hydroxyapatite (PCL/HA) blend scaffolds using a 3D plotting system for bone tissue engineering. Bioprocess Biosyst. Eng. 34 (4), 505–513. doi:10.1007/s00449-010-0499-2
Patz, T. M., Doraiswamy, A., Narayan, R. J., He, W., Zhong, Y., Bellamkonda, R., et al. (2006). Three-dimensional direct writing of B35 neuronal cells. J. Biomed. Mater. Res. B Appl. Biomater. 78 (1), 124–130. doi:10.1002/jbm.b.30473
Pinnock, C. B., Meier, E. M., Joshi, N. N., Wu, B., and Lam, M. T. (2016). Customizable engineered blood vessels using 3D printed inserts. Methods 99, 20–27. doi:10.1016/j.ymeth.2015.12.015
Pourchet, L. J., Thepot, A., Albouy, M., Courtial, E. J., Boher, A., Blum, L. J., et al. (2017). Human skin 3D bioprinting using scaffold-free approach. Adv. Healthcare Mater. 6 (4), 1601101. doi:10.1002/adhm.201601101
Rimann, M., Bono, E., Annaheim, H., Bleisch, M., and Graf-Hausner, U. (2016). Standardized 3D bioprinting of soft tissue models with human primary cells. J. Lab. Autom. 21 (4), 496–509. doi:10.1177/2211068214567146
Ripley, B., Kelil, T., Cheezum, M. K., Goncalves, A., Di Carli, M. F., Rybicki, F. J., et al. (2016). 3D printing based on cardiac CT assists anatomic visualization prior to transcatheter aortic valve replacement. J. Cardiovasc. Comput. Tomogr. 10 (1), 28–36. doi:10.1016/j.jcct.2015.12.004
Satpathy, A., Pal, A., Sengupta, S., Das, A., Hasan, M. M., Ratha, I., et al. (2019). Bioactive nano-hydroxyapatite doped electrospun PVA-chitosan composite nanofibers for bone tissue engineering applications. J. Indian Inst. Sci. 99, 289–302. doi:10.1007/s41745-019-00118-8
Sawkins, M. J., Mistry, P., Brown, B. N., Shakesheff, K. M., Bonassar, L. J., and Yang, J.. (2015). Cell and protein compatible 3D bioprinting of mechanically strong constructs for bone repair. Biofabrication 7 (3), 035004. doi:10.1088/1758%20135090/7/3/035004
Shanks, N., Greek, R., and Greek, J. (2009). Are animal models predictive for humans? Philos. Ethics Humanit. Med. 4 (1), 2. doi:10.1186/1747-5341-4-2
Singh, P., and Williams, D. J. (2008). Cell therapies: realizing the potential of this new dimension to medical therapeutics. J. Tissue Eng. Regen. Med. 2 (6), 307–319. doi:10.1002/term.108
Singh, Y. P., Bandyopadhyay, A., and Mandal, B. B. (2019). 3D bioprinting using cross-linker-free silk-gelatin bioink for cartilage tissue engineering. ACS Appl. Mater. Interfaces 11 (37), 33684–33696. doi:10.1021/acsami.9b11644
Skardal, A. (2018). Perspective: “Universal” bioink technology for advancing extrusion bioprinting-based biomanufacturing, Bioprinting 10, e00026. doi:10.1016/j.bprint.2018.e00026
Skardal, A., Zhang, J., McCoard, L., Xu, X., Oottamasathien, S., and Prestwich, G. D. (2010). Photocrosslinkable hyaluronan-gelatin hydrogels for two-step bioprinting. Tissue Eng. 16 (8), 2675–2685. doi:10.1089/ten.tea.2009.0798
Sodian, R., Loebe, M., Hein, A., Martin, D. P., Hoerstrup, S. P., Potapov, E. V., et al. (2002). Application of stereolithography for scaffold fabrication for tissue engineered heart valves. Am. Soc. Artif. Intern. Organs J. 48 (1), 12–16. doi:10.1097/00002480-200201000-00004
Starly, B., and Shirwaiker, R. (2015). “3D bioprinting techniques,” in 3D bioprinting nanotechnology in tissue engineering regenerative medicine (Cambridge, MA: Elsevier), 57–77.
Swope, V. B., Supp, A. P., and Boyce, S. T. (2002). Regulation of cutaneous pigmentation by titration of human melanocytes in cultured skin substitutes grafted to athymic mice. Wound Repair Regen. 10 (6), 378–386. doi:10.1046/j.1524-475x.2002.10607.x
Tchantchaleishvili, V., Schubmehl, H., Swartz, M. F., Hallinan, W., and Massey, H. T. (2014). Evolving strategies in the treatment of acute myocardial infarction-induced cardiogenic shock. Ann. Cardiothorac. Surg. 3 (6), 606–611. doi:10.3978/j.issn.2225-319X.2014.08.03
Tevlek, A., and Aydin, H. M. (2017). Poly (glycerol-sebacate) elastomer: a mini review. Ortho Surg. Open Access (OSOA. 000507), 1 (2), 1–4. doi:10.31031/OOIJ.2017.01.000507
Tijore, A., Irvine, S. A., Sarig, U., Mhaisalkar, P., Baisane, V., and Venkatraman, S. (2018). Contact guidance for cardiac tissue engineering using 3D bioprinted gelatin patterned hydrogel. Biofabrication 10 (2), 025003. doi:10.1088/1758-5090/aaa15d
Tomov, M. L., Theus, A., Sarasani, R., Chen, H., and Serpooshan, V. (2019). “3D bioprinting of cardiovascular tissue constructs: cardiac bioinks,” in cardiovascular regenerative medicine (Cham, Switzerland: Springer Publishing), 63–77.
Truby, R. L., and Lewis, J. A. (2016). Printing soft matter in three dimensions. Nature 540 (7633), 371–378. doi:10.1038/nature21003
Valverde, I., Gomez, G., Gonzalez, A., Suarez-Mejias, C., Adsuar, A., Coserria, J. F., et al. (2015). Three-dimensional patient-specific cardiac model for surgical planning in Nikaidoh procedure. Cardiol. Young 25 (4), 698–704. doi:10.1017/s1047951114000742
Varkey, M., Atala, A., Khademhosseini, A., and Camci-Unal, G. (2018). “Current challenges and future perspectives of bioprinting,” in 3D bioprinting in regenerative engineering: principles and applications (Boca Raton, FL: Taylor & Francis).
Varkey, M., Visscher, D. O., van Zuijlen, P. P. M., Atala, A., and Yoo, J. J. (2019). Skin bioprinting: the future of burn wound reconstruction? Burns Trauma 7, 4. doi:10.1186/s41038-019-0142-7
Vijayavenkataraman, S., Lu, W. F., and Fuh, J. Y. H. (2016). 3D bioprinting of skin: a state-of-the-art review on modelling, materials, and processes. Biofabrication 8 (3), 032001. doi:10.1088/1758-5090/8/3/032001
Vunjak-Novakovic, G., Tandon, N., Godier, A., Maidhof, R., Marsano, A., Martens, T. P., et al. (2010). Challenges in cardiac tissue engineering. Tissue Eng. B Rev. 16 (2), 169–187. doi:10.1089/ten.teb.2009.0352
Wang, Y. (2019). “Application of 3D bioprinting in cartilage tissue,” In AIP conference proceedings 2058 (Melville, NY: AIP Publishing LLC), 1, 020047.
Wang, Z., Lee, S. J., Cheng, H.-J., Yoo, J. J., and Atala, A. (2018). 3D bioprinted functional and contractile cardiac tissue constructs. Acta Biomater. 70, 48–56. doi:10.1016/j.actbio.2018.02.007
Xiong, Z., Yan, Y., Zhang, R., and Sun, L. (2001). Fabrication of porous poly(l-lactic acid) scaffolds for bone tissue engineering via precise extrusion. Scripta Mater. 45 (7), 773–779. doi:10.1016/s1359-6462(01)01094-6
Xiongfa, J., Hao, Z., Liming, Z., and Jun, X. (2018). Recent advances in 3D bioprinting for the regeneration of functional cartilage. Regen. Med. 13 (1), 73–87. doi:10.2217/rme-2017-0106
Xu, C., Chai, W., Huang, Y., and Markwald, R. R. (2012). Scaffold-free inkjet printing of three-dimensional zigzag cellular tubes. Biotechnol. Bioeng. 109 (12), 3152–3160. doi:10.1002/bit.24591
Xu, W., Wang, X., Yan, Y., Zheng, W., Xiong, Z., Lin, F., et al. (2007). Rapid prototyping three-dimensional cell/gelatin/fibrinogen constructs for medical regeneration. J. Bioact. Compat Polym. 22 (4), 363–377.
You, F., Eames, B. F., and Chen, X. (2017). Application of extrusion-based hydrogel bioprinting for cartilage tissue engineering. Int. J. Mol. Sci. 18 (7), 1597. doi:10.3390/ijms18071597
Zhang, T., Wan, L. Q., Xiong, Z., Marsano, A., Maidhof, R., Park, M., et al. (2012). Channelled scaffolds for engineering myocardium with mechanical stimulation. J. Tissue Eng. Regen. Med. 6 (9), 748–756. doi:10.1002/term.481
Zhang, Y. S., Arneri, A., Bersini, S., Shin, S.-R., Zhu, K., Goli-Malekabadi, Z., et al. (2016). Bioprinting 3D microfibrous scaffolds for engineering endothelialized myocardium and heart-on-a-chip. Biomaterials 110, 45–59. doi:10.1016/j.biomaterials.2016.09.003
Zhou, X., Zhu, W., Nowicki, M., Miao, S., Cui, H., Holmes, B., et al. (2016). 3D bioprinting a cell-laden bone matrix for breast cancer metastasis study. ACS Appl. Mater. Interfaces 8 (44), 30017–30026. doi:10.1021/acsami.6b10673
Zhu, J., Beamish, J. A., Tang, C., Kottke-Marchant, K., and Marchant, R. E. (2006). Extracellular matrix-like cell-adhesive hydrogels from RGD-containing poly(ethylene glycol) diacrylate. Macromolecules 39 (4), 1305–1307. doi:10.1021/ma052333s
Keywords: additive manufacturing, 3D bioprinting methodologies, bioink, tissue engineering, organ regeneration
Citation: Agarwal S, Saha S, Balla VK, Pal A, Barui A and Bodhak S (2020) Current Developments in 3D Bioprinting for Tissue and Organ Regeneration–A Review. Front. Mech. Eng. 6:589171. doi:10.3389/fmech.2020.589171
Received: 30 July 2020; Accepted: 22 September 2020;
Published: 30 October 2020.
Edited by:
Amit Bandyopadhyay, Washington State University, United StatesCopyright © 2020 Agarwal, Saha, Balla, Pal, Barui and Bodhak. This is an open-access article distributed under the terms of the Creative Commons Attribution License (CC BY). The use, distribution or reproduction in other forums is permitted, provided the original author(s) and the copyright owner(s) are credited and that the original publication in this journal is cited, in accordance with accepted academic practice. No use, distribution or reproduction is permitted which does not comply with these terms.
*Correspondence: Subhadip Bodhak, sbodhak@gmail.com, sbodhak@cgcri.res.in