- 1Université Libre de Bruxelles, Ecole polytechnique de Bruxelles, Aero-Thermo-Mechanics Laboratory, Bruxelles, Belgium
- 2Université Libre de Bruxelles and Vrije Universiteit Brussel, Combustion and Robust Optimization Group (BURN), Brussels, Belgium
Moderate or Intense Low-oxygen Dilution (MILD) combustion is considered as one of the most promising novel combustion technologies, as it ensures high efficiency and very low emissions (NOx and CO). Because of the high level of dilution, the system reactivity is reduced and the chemical time scale is increased compared to conventional flames. Therefore, combustion models accounting for finite-rate chemistry are needed to study the characteristics of such flames. Reactor based models, such as the Partially Stirred Reactor and the Eddy Dissipation Concept models have been successfully used to model MILD combustion. This article describes recent progress and developments in the application of reactor based models for the simulation of the jet-in-hot-coflow burners that emulate MILD combustion. The main objective is to provide an overview about the current state of the art of reactor based models for turbulence-chemistry interactions in MILD regime and outline future prospects for the further development of such models. The literature acknowledges both Reynolds Averaged Navier Stokes and Large Eddy Simulations studies, with various operating conditions as well as different fuels. The results indicate that it is necessary to include both the mixing and chemical time scales explicitly in the combustion model formulation. Because of the distributed reaction area, according to recent investigations, Large Eddy Simulation grid can be sufficient to resolve the MILD combustion reacting structures. The present review underlines the importance of finite rate chemistry in MILD combustion simulations, as well as of providing reliable estimation of the characteristic time scales.
Introduction
Facing the current challenges of air pollution and energy shortage, it is urgent to develop fuel flexible, efficient and environmentally friendly combustion technologies. Novel combustion technologies with low emissions, high efficiency and fuel flexibility have become essential under these challenges (Li, 2019). In this context, one promising technology in energy production and manufacturing is Moderate or Intense Low oxygen Dilution (MILD) combustion (Wünning and Wünning, 1997; Cavaliere and de Joannon, 2004; de Joannon et al., 2012).
MILD combustion is established by diluting the fresh reactants with the combustion products and preheating the charge above the self-ignition temperature of the fuel (de Joannon et al., 2012). The high dilution is responsible for the widening of the reaction zone, while the mixing with hot exhaust gases ensures that reaction takes place also outside of the flammability limits. In terms of characteristic scales, the dilution lowers the oxygen concentration and smooths the temperature peaks. As a result, the chemical time scale increases and the strong interaction between chemistry reaction and mixing makes the investigation of such flames more challenging than conventional combustion regimes. The availability of validation data is then crucial to advance the current understanding of MILD combustion and further push its implementation in industrial applications. To decrease the influence of geometric complexity encountered in practical devices, simplified lab-scale axis-symmetric jet burners are generally used to emulate MILD conditions—for example, the jet-in-hot-coflow (JHC) burner (Dally et al., 2002; Oldenhof et al., 2010).
The JHC burner features a central jet and a secondary burner providing hot exhaust products as a coflow, reproducing the flue gas recirculation. The oxygen level in the coflow is highly diluted by adding nitrogen and it is generally controlled below 10
Numerical investigations on the JHC burners were carried out mainly using Reynolds Averaged Navier-Stokes (RANS) (Christo and Dally, 2005; Frassoldati et al., 2009; Mardani et al., 2010; Shabanian et al., 2012; Parente et al., 2015; Evans et al., 2015; Aminian et al., 2016; Mardani, 2017; Evans et al., 2017; Chen et al., 2017; Li et al., 2017; Li et al., 2018b) simulation and Large Eddy Simulation (LES) (Afarin et al., 2011; Ihme and See, 2011; Ihme et al., 2012; Li et al., 2019). LES can capture more faithfully the flow features while RANS is still important for preliminary investigations, because of its reduced computational cost. However, despite high computational efficiency of RANS simulation, non-equilibrium phenomena are not well captured by steady-state assumptions. To this end, Large Eddy Simulation (LES) can provide superior results with respect to RANS.
Due to the presence of the coflow and the intense low-oxygen dilution, combustion in JHC burner is characterised by a relatively low Damköhler number
Reactor Based Models
Both the Partially Stirred Reactor (PaSR) approach and the Eddy Dissipation Concept (EDC) model are based on the assumption that each computational cell can be separated into two zones. Th reacting zone is generally referred to as “fine structures” (Magnussen, 1981; Chomiak, 1990; Gran and Magnussen, 1996; Golovitchev and Chomiak, 2001; Magnussen, 2005) while the non-reacting one is the “surrounding fluid”. Combustion takes place in the fine structures in contact with a surrounding fluid. The average reaction rate in a cell is then the results of an exchange between the fine structure and surrounding fluid. The fine structures are modelled as Perfectly Stirred Reactors (PSR) or Plug Flow Reactors (PFR). A conceptual drawing of the PaSR and EDC model is presented in Figure 1.
Eddy Dissipation Concept Model
The EDC model is based on a cascade model (Gran and Magnussen, 1996) providing the mass fraction of the fine structures,
In Eq. 1 and Eq. 2, ν is the kinematic viscosity,
The term
Partially Stirred Reactor Model
In the PaSR model (Chomiak, 1990; Golovitchev and Chomiak, 2001), the parameter κ is used to represent the mass fraction of the reaction zone (fine structures) in the computational cell. It can be estimated as (Chomiak, 1990; Golovitchev and Chomiak, 2001; Kärrholm, 2008):
where
where
Finite-Rate Chemistry Effects
In both models, the mean mass fraction
where
Discussion
Successful predictions of JHC flames are reported in the literature. In the context of RANS simulation using EDC model, over-predictions of temperature were observed using standard EDC constants of
De et al. (2011) studied Delft JHC with Dutch natural gas as a fuel, numerically. Under-prediction of the flame lift-off height was observed with the EDC model, while the flame temperature were generally over-estimated. The authors proposed to change not only the time scale constant
The modified
Evans et al. (Evans et al., 2015) carried out a systematic study and found out that adjusting the EDC parameters to
The studies discussed above focused on the modification of
Several sensitivity analyses have been carried out on the JHC burner using EDC model (De et al., 2011; Aminian et al., 2012; Shabanian et al., 2012; Tu et al., 2016; Mardani, 2017; Li et al., 2018a; Li et al., 2018b), to investigate the effect of different modelling choices, focusing on turbulent and combustion model parameters, as well as on the fuel and co-flow compositions. A comprehensive sensitivity study of the JHC burner was carried out by Li et al. (2017), using RANS. Their investigation includes the effect of turbulent combustion model formulations, boundary conditions, differential diffusion, turbulence model parameters and kinetic mechanisms on the results. Results showed that the reactors chosen, namely as Perfectly Stirred Reactors (PSR) or Plug Flow Reactors (PFR), to model the reaction fine structures, do not have a major impact on the results. Moreover, increased kinetic mechanism complexity does not lead to major improvements on the numerical predictions, suggesting that low-temperature oxidation mechanism are not relevant to the fuels and conditions investigated. While the inclusion of differential (molecular) diffusion and the appropriate choice of turbulent non-dimensional values such as Schmidt and Prandtl numbers helped to improve the prediction accuracy. The effect of molecular diffusion was also investigated by Salavati-Zadeh et al. (2018) with the conclusion that the inclusion of the molecular diffusion with proper Schmidt numbers for each species improves the prediction accuracy. The importance of differential diffusion was recognized by Christo and Dally, (2005) and Mardani et al. (2010) as well. In the work of Li et al. (2017), three different Eddy Dissipation Concept (EDC) model formulations were compared as well, showing their interaction with the choice of the
All EDC modifications presented above were based on a fitting procedure aimed at alleviating the temperature over-estimation observed with the standard EDC formulation. Parente et al. (2015) proposed a modification of EDC based on the revision of the energy cascade, taking into account the microscopically distributed features of MILD combustion. In particular, it was assumed that the reacting structures propagated with a turbulent flame speed expressed using the Damköhler formulation for high-intensity turbulence. With this assumption, the energy cascade coefficients
With the exception of the EDC formulation proposed by Parente et al. (2015) and Evans et al. (2019), the mass fraction of the fine structures and the residence time are solely determined by flow properties in EDC. However, MILD combustion is driven by the strong overlap of fluid dynamic and chemical scales. This suggests that the characteristic chemical time scale shall be included in the definition of the reacting structure features. This has pushed the investigation of PaSR approach for modelling MILD combustion. As indicated in Section 2.2, both chemical time scale and mixing time scale are used for the determination of κ, which is the factor accounting for non-perfect mixing (turbulence-chemistry interaction). Li et al. (2017) compared the EDC model with standard model constants to the standard PaSR model, for the simulation of JHC burner with C2H4, H2, H2O as fuel. The prediction of mean temperate and species mass fraction (including
The JHC configuration was also investigated using LES, to improve the prediction of intermediate and minor species, as well as to capture the intermittency observed in some configurations when increasing the fuel jet Reynolds number (Parente et al., 2015). LES using a three-stream Flamelet Progress Variable (FPV) formulation (tabulated based model) was employed by Ihme et al. (Ihme and See, 2011; Ihme et al., 2012) to model the JHC flame. Satisfactory agreement with the experimental data were obtained for mean temperature and major species mass fractions. While the use of FPV-based approaches in MILD combustion is promising, the highly diluted feature of MILD combustion often leads to high dimensional tables (Lamouroux et al., 2014; Locci et al., 2014), whose generation can be challenging and time consuming. Afarin et al. (2011) used PaSR to investigate the reaction zone structure and the distribution of temperature and minor species mass fractions, showing satisfactory accuracy. Li et al. (2019) presented a detailed comparison between the conventional PaSR model and two implicit combustion models, in which the filtered source term comes directly from the chemical term, without inclusion of turbulence effects. Results indicated that all three models could accurately predict the combustion of CH
A detailed comparison of different models applied on the JHC configuration is presented in Table 1 and the prediction errors on several axial locations is summarized in Table 2. The errors reported in Table 2 focus on the JHC burner with CH
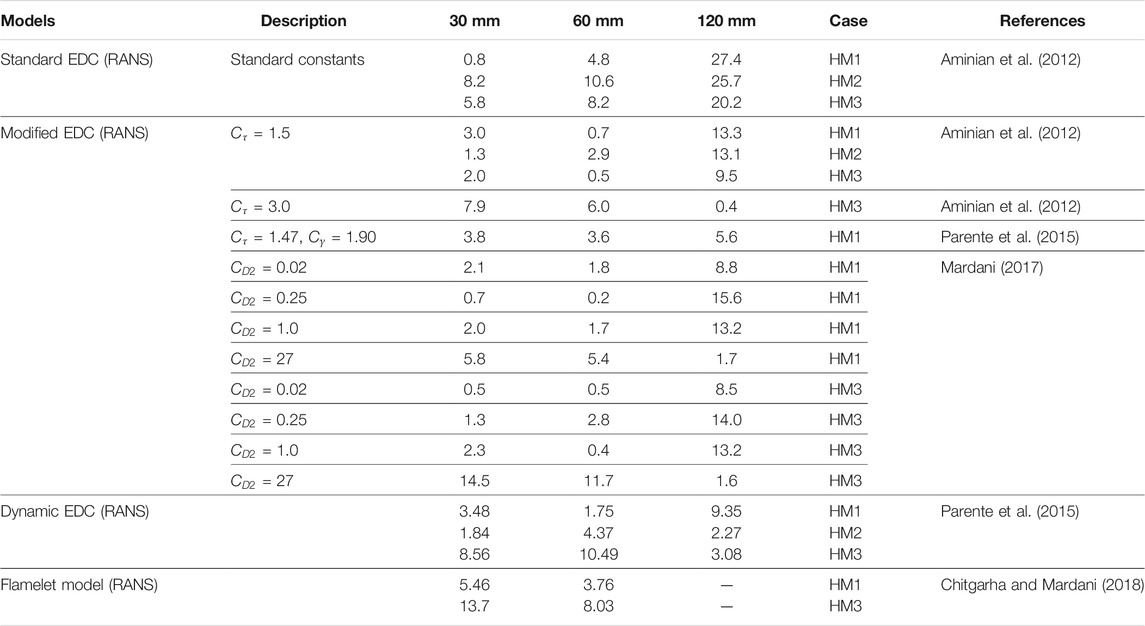
TABLE 2. Absolute errors for temperature predictions (
Conclusion
The present paper reports a short review on the application of reactor based models to the simulation of a canonical MILD combustion system, the jet in hot coflow (JHC) burner. Successful predictions of JHC burner with finite-rate models were reported, with both Reynolds Average Navier-Stokes (RANS) simulation and Large Eddy Simulation (LES). The main conclusions drawn can be summarized as follows:
• The Eddy Dissipation Concept (EDC) model and its modified versions (with modified model constants) were first considered by most authors (De et al., 2011; Aminian et al., 2012; Shabanian et al., 2012; Tu et al., 2016; Mardani, 2017; Li et al., 2018a; Li et al., 2018b) for the prediction of a jet-in-hot-coflow (JHC) burner with RANS simulation. However, there is a lack of generality.
• The estimation of local EDC parameters based on the local turbulent Reynolds number and Damköhler numbers (Parente et al., 2015) could significantly improve the predictions in the context of MILD combustion, making the inclusion of chemical time scale (needed to estimate Damköhler number) important.
• In PaSR, the turbulence-chemistry interaction factor is based on a more general definition which requires the estimation of both chemical and mixing time scales. The choice of such scales has a crucial impact on the model prediction (Li et al., 2018a).
• A dynamic evaluation of mixing time scale in PaSR RANS formulation was proposed, presenting superior performance than the other globally defined mixing models under a wide range of JHC flame operating conditions (Li et al., 2018a; Ferrarotti et al., 2019).
The LES formulation of PaSR model along with other implicit combustion models show superior advantage for the prediction on the JHC flames (Li et al., 2019).
• Future studies shall focus on the generalisation and unification of reactor-based approaches, using available DNS data in MILD combustion (Minamoto and Swaminathan, 2015; Doan and Swaminathan, 2019) together with machine learning and optimization algorithms.
• Future studies should also target more complex fuels, such as oxygenated hydrocarbons and long-chain alkanes under MILD conditions. Because they have shown different characteristics, such as the appearance of visible flames and increased pollutant emissions, as indicated by (Weber et al., 2005; Saha et al., 2014; Ye et al., 2015b).
Conflict of Interest
The authors declare that the research was conducted in the absence of any commercial or financial relationships that could be construed as a potential conflict of interest.
Author Contributions
ZL wrote this paper. AP contributed to the paper conception and writing.
Funding
This project has received funding from the European Research Council, Starting Grant No. 714605, and the European Union’s Horizon 2020 research and innovation program under the Marie Skłodowska-Curie grant agreement No. 643134.
Acknowledgments
Some sentences in the article were rephrased from the PhD thesis (Li et al., 2019) of the first author ZL.
References
Afarin, Y., Tabejamaat, S., and Mardani, A. (2011). “Large eddy simulation study of H2/CH4 flame structure at MILD condition.” In Seventh mediterranean combustion symposium, Naples, Italy, September 11–September 15
Aminian, J., Galletti, C., Shahhosseini, S., and Tognotti, L. (2012). Numerical investigation of a MILD combustion burner: analysis of mixing field, chemical kinetics and turbulence-chemistry interaction. Flow, Turbul. Combust. 88, 597–623. doi:10.1007/s10494-012-9386-z
Aminian, J., Galletti, C., and Tognotti, L. (2016). Extended EDC local extinction model accounting finite-rate chemistry for MILD combustion. Fuel 165, 123–133. doi:10.1016/j.fuel.2015.10.041
Cavaliere, A., and de Joannon, M. (2004). MILD combustion. Prog. Energy Combust. Sci. 30, 329–366. doi:10.1016/j.pecs.2004.02.003
Chen, Z., Reddy, V., Ruan, S., Doan, N., Roberts, W., and Swaminathan, N. (2017). Simulation of mild combustion using perfectly stirred reactor model. Proc. Combust. Inst. 36, 4279–4286. doi:10.1016/j.proci.2016.06.007
Chitgarha, F., and Mardani, A. (2018). Assessment of steady and unsteady flamelet models for mild combustion modeling. Int. J. Hydrogen Energy 43, 15551–15563. doi:10.1016/j.ijhydene.2018.06.071
Chomiak, J. (1990). Combustion: a study in theory, fact and application. New York, NY: Abacus Press/Gorden and Breach Science Publishers
Christo, F. C., and Dally, B. B. (2005). Modelling turbulent reacting jets issuing into a hot and diluted coflow. Combust. Flame 142, 117–129. doi:10.1016/j.combustflame.2005.03.002
Dally, B. B., Karpetis, A. N., and Barlow, R. S. (2002). Structure of turbulent non-premixed jet flames in a diluted hot coflow. Proc. Combust. Inst. 29, 1147–1154. doi:10.1016/s1540-7489(02)80145-6
De, A., Oldenhof, E., Sathiah, P., and Roekaerts, D. (2011). Numerical simulation of Delft-Jet-in-Hot-Coflow (DJHC) flames using the Eddy Dissipation Concept model for turbulence-chemistry interaction. Flow, Turbul. Combust. 87, 537–567. doi:10.1007/s10494-011-9337-0
de Joannon, M., Sorrentino, G., and Cavaliere, A. (2012). MILD combustion in diffusion-controlled regimes of hot diluted fuel. Combust. Flame 159, 1832–1839. doi:10.1016/j.combustflame.2012.01.013
Doan, N. A. K., and Swaminathan, N. (2019). Autoignition and flame propagation in non-premixed MILD combustion. Combust. Flame 201, 234–243. doi:10.1016/j.combustflame.2018.12.025
Evans, M., Chinnici, A., Medwell, P., and Ye, J. (2017). Ignition features of methane and ethylene fuel-blends in hot and diluted coflows. Fuel 203, 279–289. doi:10.1016/j.combustflame.2018.12.025
Evans, M. J., Medwell, P. R., and Tian, Z. F. (2015). Modelling lifted jet flames in a heated coflow using an optimised eddy dissipation concept model. Combust. Sci. Technol. 187, 1093–1109. doi:10.1016/j.fuel.2017.04.113
Evans, M., Petre, C., Medwell, P., and Parente, A. (2019). Generalisation of the eddy-dissipation concept for jet flames with low turbulence and low damköhler number. Proc. Combust. Inst. 37, 4497–4505. doi:10.1080/00102202.2014.1002836
Ferrarotti, M., Li, Z., and Parente, A. (2019). On the role of mixing models in the simulation of MILD combustion using finite-rate chemistry combustion models. Proc. Combust. Inst. 37, 4531–4538. doi:10.1016/j.proci.2018.06.017
Frassoldati, A., Sharma, P., Cuoci, A., Faravelli, T., and Ranzi, E. (2009). Kinetic and fluid dynamics modeling of methane/hydrogen jet flames in diluted coflow. Appl. Therm. Eng. 30, 376–383. doi:10.1016/j.applthermaleng.2009.10.001
Golovitchev, V., and Chomiak, J. (2001). Numerical modeling of high temperature air flameless combustion. In The 4th international symposium on high temperature air combustion and gasification
Gran, I., and Magnussen, B. F. (1996). A numerical study of a bluff-body stabilized diffusion flame, part 2: influence of combustion modelling and finite-rate chemistry. Combust. Sci. Technol. 119, 191–217. doi:10.1080/00102209608951999
Haworth, D., and Pope, S. (2010). Transported probability density function methods for Reynolds-averaged and large-eddy simulations. New York, NY:(Springer)
Ihme, M., and See, Y. C. (2011). LES flamelet modeling of a three-stream MILD combustor: analysis of flame sensitivity to scalar inflow conditions. Proc. Combust. Inst. 33, 1309–1317. doi:10.1016/j.proci.2010.05.019
Ihme, M., Zhang, J., He, G., and Dally, B. (2012). Large Eddy Simulation of a Jet-in-Hot-Coflow burner operating in the oxygen-diluted combustion regime. Flow, Turbul. Combust. 89, 449–464 doi:10.1007/s10494-012-9399-7
Kärrholm, F. P. (2008). Numerical Modelling of diesel spray injection, turbulence Interaction and combustion. Phd thesis, Chalmers University of Technology, Chalmers, Sweden
Kim, S. H., Huh, K. Y., and Dally, B. (2005). Conditional moment closure modeling of turbulent nonpremixed combustion in diluted hot coflow. Proc. Combust. Inst. 30, 751–757. doi:10.1016/j.proci.2004.08.161
Lamouroux, J., Ihme, M., Fiorina, B., and Gicquel, O. (2014). Tabulated chemistry approach for diluted combustion regimes with internal recirculation and heat losses. Combust. Flame 161, 2120–2136. doi:10.1016/j.combustflame.2014.01.015
Lewandowski, M. T., and Ertesvåg, I. S. (2018). Analysis of the eddy dissipation concept formulation for mild combustion modeling. Fuel 224, 687–700. doi:10.1016/j.fuel.2018.03.110
Lewandowski, M. T., Li, Z., Parente, A., and Pozorski, J. (2020a). Generalised eddy dissipation concept for mild combustion regime at low local Reynolds and damköhler numbers. part 2: validation of the model. Fuel 278, 117773 doi:https://doi.org/10.1016/j.fuel.2020.117773
Lewandowski, M. T., Parente, A., and Pozorski, J. (2020b). Generalised eddy dissipation concept for mild combustion regime at low local Reynolds and damköhler numbers. part 1: model framework development. Fuel 278, 117743, doi:10.1016/j.fuel.2020.117743
Li, Z., Cuoci, A., and Parente, A. (2019). Large eddy simulation of mild combustion using finite rate chemistry: effect of combustion sub-grid closure. Proc. Combust. Inst. 37, 4519–4529. doi:10.1016/j.proci.2018.09.033
Li, Z., Cuoci, A., Sadiki, A., and Parente, A. (2017). Comprehensive numerical study of the Adelaide Jet in Hot-Coflow burner by means of RANS and detailed chemistry. Energy 139, 555–570. doi:10.1016/j.energy.2017.07.132
Li, Z., Ferrarotti, M., Cuoci, A., and Parente, A. (2018a). Finite-rate chemistry modelling of non-conventional combustion regimes using a partially-stirred reactor closure: combustion model formulation and implementation details. Appl. Energy 225, 637–655. doi:10.1016/j.apenergy.2018.04.085
Li, Z., Lewandowski, M. T., Contino, F., and Parente, A. (2018b). Assessment of on-the-fly chemistry reduction and tabulation approaches for the simulation of moderate or intense low-oxygen dilution combustion. Energy and Fuels 32, 10121–10131. doi:10.1021/acs.energyfuels.8b01001
Li, Z. (2019). Sub-grid models for Large Eddy Simulation of non-conventional combustion regimes. Ph.D. thesis. Darmstadt(Germany): Université libre de Bruxelles and Technische Universitæt Darmstadt
Locci, C., Colin, O., and Michel, J. B. (2014). Large Eddy simulations of a small-scale flameless combustor by means of diluted homogeneous reactors. Flow, Turbul. Combust. 93, 305–347. doi:10.1007/s10494-014-9548-2
Ma, L., Naud, B., and Roekaerts, D. (2016). Transported pdf modeling of ethanol spray in hot-diluted coflow flame. Flow, Turbul. Combust. 96, 469–502. doi:10.1007/s10494-015-9623-3
Magnussen, B. F. (1981). “On the structure of turbulence and a generalized Eddy Dissipation Concept for chemical reaction in turbulent flow.” In 19
Magnussen, B. F. (2005). “The Eddy Dissipation Concept a bridge between science and technology.” In ECCOMAS thematic conference on computational combustion, Lisbon, Portugal
Mahalegi, H. K. M., and Mardani, A. (2019). Ethanol spray combustion under a mild condition: a chemical kinetic study. Energy & Fuels 33, 11861–11886. doi:10.1021/acs.energyfuels.9b02665
Mardani, A., and Karimi Motaalegh Mahalegi, H. (2019). Hydrogen enrichment of methane and syngas for mild combustion. Int. J. Hydrogen Energy 44, 9423–9437. doi:10.1016/j.ijhydene.2019.02.072
Mardani, A. (2017). Optimization of the Eddy Dissipation Concept (EDC) model for turbulence-chemistry interactions under hot diluted combustion of ch4/h2. Fuel 191, 114–129. doi:10.1016/j.fuel.2016.11.056
Mardani, A., Tabejamaat, S., and Ghamari, M. (2010). Numerical study of influence of molecular diffusion in the MILD combustion regime. Combust. Theor. Model. 14, 747–774. doi:10.1080/13647830.2010.512959
Medwell, P. R., and Dally, B. B. (2012). Effect of fuel composition on jet flames in a heated and diluted oxidant stream. Combust. Flame 159, 3138–3145. doi:10.1016/j.combustflame.2012.04.012
Medwell, P. R., Kalt, P. A., and Dally, B. B. (2007). Imaging of diluted turbulent ethylene flames stabilized on a Jet in Hot Coflow (JHC) burner. Combust. Flame 152, 100–113. doi:10.1016/j.combustflame.2007.09.003
Minamoto, Y., and Swaminathan, N. (2015). Subgrid scale modelling for MILD combustion. Proc. Combust. Inst. 35, 3529–3536. doi:10.1016/j.proci.2014.07.025
Oldenhof, E., Tummers, M. J., van Veen, E., and Roekaerts, D. (2010). Ignition kernel formation and lift-off behaviour of Jet-in-Hot-Coflow flames. Combust. Flame 157, 1167–1178. doi:10.1016/j.combustflame.2010.01.002
Parente, A., Malik, M. R., Contino, F., Cuoci, A., and Dally, B. B. (2015). Extension of the Eddy Dissipation Concept for turbulence/chemistry interactions to MILD combustion. Fuel 163, 98–111. doi:10.1016/j.fuel.2015.09.020
Saha, M., Dally, B. B., Medwell, P. R., and Cleary, E. M. (2014). Moderate or Intense Low oxygen Dilution (MILD) combustion characteristics of pulverized coal in a self-recuperative furnace. Energy & Fuels 28, 6046–6057. doi:10.1021/ef5006836
Salavati-Zadeh, A., Esfahanian, V., Najafi], S. B. N., Saeed, H., and Mohammadi, M. (2018). Kinetic simulation of flameless burners with methane/hydrogen blended fuel: effects of molecular diffusion and schmidt number. Int. J. Hydrogen Energy 43, 5972–5983. doi:10.1016/j.ijhydene.2017.11.149
Shabanian, S. R., Medwell, P. R., Rahimi, M., Frassoldati, A., and Cuoci, A. (2012). Kinetic and fluid dynamic modeling of ethylene jet flames in diluted and heated oxidant stream combustion conditions. Appl. Therm. Eng. 52, 538–554. doi:10.1016/j.applthermaleng.2012.12.024
Tu, Y., Su, K., Liu, H., Chen, S., Liu, Z., and Zheng, C. (2016). Physical and chemical effects of CO2 addition on CH4/H2 flames on a Jet-in-Hot-Coflow (JHC) burner. Energy and Fuels 30, 1390–1399. doi:10.1021/acs.energyfuels.5b02499
Wünning, J. A., and Wünning, J. G. (1997). Flameless oxidation to reduce thermal NO-formation. Prog. Energy Combust. Sci. 23, 81–94. doi:10.1016/s0360-1285(97)00006-3
Weber, R., Smart, J. P., and vd Kamp, W. (2005). On the (MILD) combustion of gaseous, liquid, and solid fuels in high temperature preheated air. Proc. Combust. Inst. 30, 2623–2629. doi:10.1016/j.proci.2004.08.101
Ye, J., Medwell, P. R., Evans, M. J., and Dally, B. B. (2017). Characteristics of turbulent n-heptane jet flames in a hot and diluted coflow. Combust. Flame 183, 330–342. doi:10.1016/j.combustflame.2017.05.027
Ye, J., Medwell, P. R., Kleinheinz, K., Evans, M. J., Dally, B. B., and Pitsch, H. G. (2018). Structural differences of ethanol and dme jet flames in a hot diluted coflow. Combust. Flame 192, 473 – 494. doi:10.1016/j.combustflame.2018.02.025
Ye, J., Medwell, P. R., Varea, E., Kruse, S., Dally, B. B., and Pitsch, H. G. (2015a). An experimental study on mild combustion of prevaporised liquid fuels. Appl. Energy 151, 93–101. doi:10.1016/j.apenergy.2015.04.019
Keywords: jet-in-hot-coflow burner, reactor-based models, MILD combustion, turbulence-chemistry interaction, finite rate chemistry
Citation: Li Z and Parente A (2020) A Review of the Numerical Investigations of Jet-In-Hot-Coflow Burner With Reactor-Based Models. Front. Mech. Eng. 6:512501. doi: 10.3389/fmech.2020.512501
Received: 15 November 2019; Accepted: 05 October 2020;
Published: 16 November 2020.
Edited by:
Timothy S. Fisher, University of California, Los Angeles, United StatesReviewed by:
Kan Zha, Sandia National Laboratories (SNL), United StatesAmir Mardani, Sharif University of Technology, Iran
Copyright © 2020 Li and Parente. This is an open-access article distributed under the terms of the Creative Commons Attribution License (CC BY). The use, distribution or reproduction in other forums is permitted, provided the original author(s) and the copyright owner(s) are credited and that the original publication in this journal is cited, in accordance with accepted academic practice. No use, distribution or reproduction is permitted which does not comply with these terms.
*Correspondence: Zhiyi Li, emhpeWkubGlAdWxiLmFjLmJl; Alessandro Parente, YWxlc3NhbmRyby5wYXJlbnRlQHVsYi5hYy5iZQ==