- 1CAS Key Laboratory of Magnetic Materials and Devices, Ningbo Institute of Materials Technology and Engineering, Chinese Academy of Sciences, Ningbo, China
- 2Zhejiang Province Key Laboratory of Magnetic Materials and Application Technology, Ningbo Institute of Materials Technology and Engineering, Chinese Academy of Sciences, Ningbo, China
- 3Center of Materials Science and Optoelectronics Engineering, University of Chinese Academy of Sciences, Beijing, China
- 4School of Future Technology, University of Chinese Academy of Sciences, Beijing, China
Transition metal oxides (TMOs) have emerged as promising candidates for spintronic applications due to their unique electronic properties and novel quantum states. The intricate interplay between strong spin-orbit coupling and electronic correlations in TMOs gives rise to distinct spin and orbital textures, leading to enhanced spin-momentum locking and efficient charge-spin interconversion. Remarkably, recent researches have unveiled the significant and highly tunable nature of charge-spin interconversion efficiency in TMOs, which can be manipulated through strategies such as electric field gating, epitaxial strain, and heterostructure engineering. This review provides a comprehensive overview of the recent advances in understanding the electronic band structures of TMOs and their correlation with charge-spin interconversion mechanisms. We summarize the tunability of these properties through various experimental approaches and discuss the potential implications for spintronic device applications. The insights gained from this review can guide future research efforts towards the development of high-performance, energy-efficient spintronic devices based on TMOs.
1 Introduction
The rapid development of the information technologies, such as artificial intelligence, the Internet of Things, and big data, has led to an exponential growth in data generation and processing requirements. This unprecedented growth has created an urgent need for the development of novel materials and devices capable of efficient and reliable information storage and processing. While the current mainstream information storage (SRAM, DRAM, etc.) and processing (CPU, GPU, etc.) devices is based on transistor CMOS technology, which manipulates the charge properties of electrons, they face significant challenges in meeting the ever-increasing performance demands of the information technology era. In the past decades, CMOS devices have achieved remarkable improvements in performance, with transistor density doubling every 18 months, following Moore’s Law. This has been accomplished by miniaturizing transistor feature sizes to increase the number of transistors per unit area. However, as transistor dimensions approach the nanometer scale, further miniaturization poses unprecedented challenges related to device performance and reliability. First, the quantum tunneling effect becomes prominent, leading to a weakening of the channel’s ability to control charge flow and an increase in leakage current; second, while increasing current density can enhance charge control, it also leads to a significant rise in Joule heating, which can compromise device durability and energy efficiency. To address these challenges and enable sustainable growth in device performance, various solutions have been proposed, spanning from fundamental scientific research to technological innovations (Agarwal et al., 2021). Among these, spintronics has emerged as a promising alternative to conventional charge-based electronics.
Spintronics exploits the spin property of electrons, alongside their charge, to achieve high-performance information storage and processing, has become a widely focused topic (Manipatruni et al., 2018; Dieny et al., 2020). A key focus in the field of spintronics is the efficient conversion between charge and spin current, which is essential for the generation, manipulation, and detection of spin-based information. The charge-spin interconversion is mediated by the spin-orbit coupling (SOC) effect, which couples the motion of electrons (charge) with their spin angular momentum. The efficiency and mechanisms of charge-spin interconversion are strongly dependent on the electronic band structure of materials with strong SOC and crucial for developing efficient spin-based devices.
In this context, transition metal oxides have garnered significant attention as promising candidates for spintronic applications. These materials exhibit a rich variety of physical properties arising from the complex interplay between charge, spin, orbital, and lattice degrees of freedom (Ramesh and Schlom, 2019). This coupling gives rise to diverse phenomena such as magnetism, ferroelectricity, superconductivity, and colossal magnetoresistance, which are closely related to electron correlation effects (Schilling et al., 1993; Tomioka et al., 1995; Li et al., 2004; Vrejoiu et al., 2006). Moreover, recent studies have revealed that the electronic structures of transition metal oxides can host various novel quantum states, such as topological insulators, Dirac and Weyl semimetals, and quantum anomalous Hall insulators, which are intimately connected to SOC and highly sensitive to external perturbations. These exotic electronic structures are expected to significantly influence the spin transport properties and charge-spin interconversion efficiency of transition metal oxides, offering new opportunities for the development of advanced spintronic devices (Chen and Yi, 2021; Trier et al., 2021).
In this review, we present a comprehensive overview of the recent progress in exploration of novel quantum states and charge-spin interconversion in transition metal oxides. We begin by introducing the fundamental concepts and mechanisms underlying the emergence of novel electronic states in these materials and their impact on spin-dependent transport properties. We then discuss the experimental and theoretical advances in understanding and harnessing charge-spin interconversion in representative transition metal oxide systems, focusing on perovskite oxides with 3
2 Mechanisms of electronic structure and charge-spin interconversion
2.1 Generation of novel electronic structures
In transition metal oxides, due to the intricate coupling between spin, orbital, charge, and lattice degrees of freedom gives rise to a plethora of quantum states that have not been anticipated in other material systems, such as Mott insulators, topological insulators, topological semimetals, and axion insulators (Witczak-Krempa et al., 2014). These unique electronic structures form the foundation for the emergence of novel physical properties and functionalities in transition metal oxides. Moreover, at the oxide heterojunction/surface, the breaking of spatial inversion symmetry introduces additional influences, leading to the formation of surface states differ from the bulk electronic structure. Among these electronic states, non-trivial topological band structures and Rashba surface states are of particular interest due to their ability to strongly influence the spin orientation and momentum of electrons. This spin-momentum locking effect endows the related oxide materials with the property of charge-spin interconversion, enabling the efficient manipulation of spin current through other means.
2.1.1 Non-trivial band structures
The generation of non-trivial band structures is usually based on the occurrence of band inversion, that is, the originally separated conduction and valence bands change their energy or width under the action of SOC, structural changes, and other factors, resulting in mutual crossing (Figure 1). When SOC is present in the system, it further opens up an energy gap at the crossing points. Depending on the extent of the gap opening, various topological states can emerge. If the energy gap is fully opened, topological insulators may be produced. If the energy gap is not fully opened, leaving some contact points, linear dispersion Dirac points or Weyl points will be produced. If some contact lines are left, Dirac nodal lines will be produced. The generation of Dirac and Weyl states has different symmetry requirements: Dirac points require the material to simultaneously satisfy spatial inversion symmetry and time-reversal symmetry, while Weyl points require the breaking of spatial inversion symmetry or time-reversal symmetry. In other words, a Dirac point can be transformed into two Weyl points by breaking the symmetries (Yan and Felser, 2017; Armitage et al., 2018). Thus, by tuning the SOC strength and symmetry, one can control the electronic structure and realize the generation and transformation of novel quantum states.
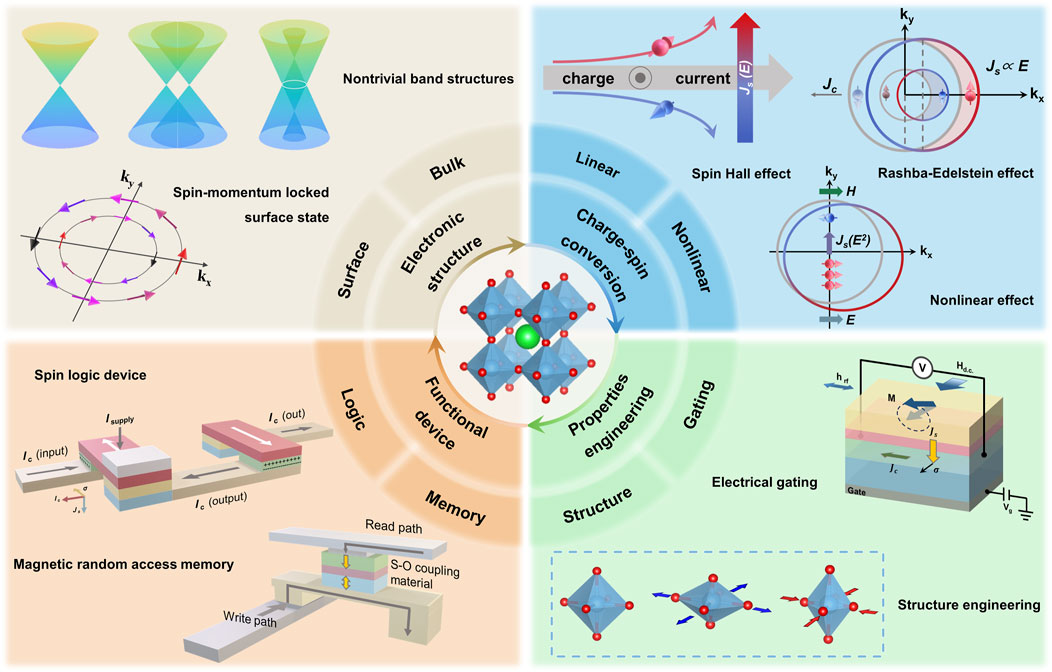
Figure 1. Transition metal oxides: Exploring novel electronic structures, charge-spin interconversion mechanisms, manipulation strategies, and their potential for advanced spintronic devices.
These topological band structures can produce large Berry curvature, which determines the intrinsic spin Hall effect (Xiao et al., 2010; Sinova et al., 2015). Therefore, when the Fermi surface is located near these topological band structures, it will significantly affect the material’s charge-spin interconversion properties. Although non-trivial band structures also exist in other material systems, the complex interplay of multiple degrees of freedom in transition metal oxides makes their electronic structure highly sensitive and tunable. Consequently, topological properties in these materials can be generated, changed, and enhanced through flexible regulation methods.
2.1.2 Surface states
Due to the inability of the non-trivial topological electronic structure to undergo continuous transformation at the vacuum or heterogeneous material interface, the naturally existing spatial inversion symmetry broken at the interface/surface will lead to surface states different from the bulk electronic structure. In topological materials with time-reversal symmetry, the energy
2.2 Mechanisms of charge-spin interconversion
2.2.1 Spin Hall effect
When a charge current passes through a material with strong SOC, electrons with different spin orientations will deflect in opposite directions, producing a spin current. This phenomenon is called the spin Hall effect (Figure 1). In the spin Hall effect, the charge current direction, spin current direction, and spin direction satisfy a mutually perpendicular relationship. The generated spin current enters the neighboring magnetic layer and exchanges angular momentum with the magnetic moments, producing a spin-orbit torque. The physical origins of the spin Hall effect include three mechanisms: skew scattering, side jump, and Berry curvature in the band (Sinova et al., 2015). Skew scattering and side jump are extrinsic contributions caused by impurity scattering, while Berry curvature is an intrinsic property determined by the band structure (Figure 1).
In the process of impurity scattering, SOC leads to an asymmetry in the scattering probabilities,
The total spin Hall conductivity
Studies have shown that transition metal oxides, especially 4
2.2.2 Rashba-Edelstein effect
In topological surface states and Rashba surface states, the spin-momentum locking property causes electronic states with opposite momenta to have opposite spin orientations at the Fermi surface. When a charge current passes through these surface states, the number of electrons with momenta in the same direction as the charge current increases, leading to an imbalance in the originally dynamically balanced spin electron numbers, resulting in spin accumulation (Figure 1). This phenomenon is called the Rashba-Edelstein effect (Manchon et al., 2015). The resulting spin accumulation can diffuse into the neighboring magnetic layer and interact with the magnetic moments to produce a spin-orbit torque.
Conversely, if a perpendicular spin current is injected into the surface states, spin accumulation will occur at the surface. Due to spin-momentum locking, the spin accumulation will cause asymmetric scattering between electrons with opposite momenta and spins, resulting in a greater number of electrons with momenta locked to the direction of spin accumulation, thus leading to a conversion from spin to charge. This process is called the inverse Rashba-Edelstein effect or spin galvanic effect and can be used to detect spin current at interfaces/surfaces.
The Rashba-Edelstein effect and its inverse process provide a mechanism for efficient interconversion between charge and spin currents in materials with strong spin-orbit coupling and broken inversion symmetry. The spin-momentum locking property of topological and Rashba surface states plays a crucial role in enabling this interconversion, making these states promising candidates for spintronic applications.
2.2.3 Nonlinear effects
The mechanisms described above reflect the charge-spin interconversion that is generated from the electronic structure of the material and exhibits a linear response relationship with the charge current (or electric field
3 Transition metal oxide material systems
When a charge current enters the spin source material, a portion of the charge current is converted into a spin current under the action of the charge-spin interconversion mechanism. The spin current passes through the interface into the neighboring magnetic material and exchanges angular momentum with the magnetic moments, producing a spin-orbit torque (SOT), including the damping-like torque ∼
Recent discoveries of novel quantum states in TMOs have opened up exciting possibilities for their use as high-performance spin source materials. These quantum states exhibit a strong correlation with charge-spin interconversion and offer a high degree of tunability. To unlock the full potential of TMOs as high-performance spin source materials, researchers are exploring two key strategies: the application of external electric fields and structural engineering techniques. External electric fields can be used to modulate the spin-orbit torque and control the efficiency and direction of charge-spin conversion in TMOs by influencing factors such as charge density, surface states, and SOC (Lesne et al., 2016; Ben Shalom et al., 2010; Caviglia et al., 2010; Vaz et al., 2019; Kaneta-Takada et al., 2022; Noël et al., 2020; Grezes et al., 2023; Gallego et al., 2024). In addition to external electric fields, structural engineering provides another powerful tool for enhancing charge-spin conversion efficiency in TMOs. Structural engineering techniques, including the control of oxygen octahedral rotations, strain engineering, crystal orientation selection, interface engineering, and thickness control, can be employed to precisely design the microscopic structure of TMOs and optimize their charge-spin conversion efficiency (Everhardt et al., 2019; Liu et al., 2019; Nan et al., 2019; Ou et al., 2019; Wang et al., 2019; Huang et al., 2021; Wei et al., 2021; Zhou et al., 2021; Lao et al., 2022; Liu et al., 2022; Zhang et al., 2022; Li et al., 2023; Zhang et al., 2023; Zhang et al., 2024; Zhao et al., 2024).
Spintronic devices based on charge-spin interconversion offer several compelling advantages for information storage, transmission, and processing, including non-volatility, high storage density, low power consumption, and fast response time. Researchers are exploring the use of spin-transfer torque (STT) and SOT to drive magnetization switching and achieve high-performance spintronic devices. Current-induced SOT has emerged as a promising, energy-efficient approach for next-generation spintronic devices (Figure 1). TMOs have garnered significant attention as spin source materials due to their remarkable and highly tunable charge-spin conversion efficiency, making them an ideal platform for spintronic applications. While TMOs have demonstrated efficient control of magnetic materials through SOT, achieving deterministic switching at room temperature without external magnetic fields remains a challenge. Recent breakthroughs in TMO-based SOT devices have shown promising results, but further research is needed to investigate and optimize field-free switching for practical spintronic applications (Liu et al., 2019; Liu et al., 2022; Li et al., 2023; Zhao et al., 2024; Tang et al., 2022).
In the following section, we will introduce promising spin source materials from the perovskite family, including 3
3.1 3d transition metal oxides: strontium titanate
3
The electronic structure of bulk insulating SrTiO3 undergoes changes at the surface/interface due to the influence of energy discontinuity and changes in the valence state, leading to the emergence of novel electronic properties that are distinct from those of the bulk material. The originally unoccupied
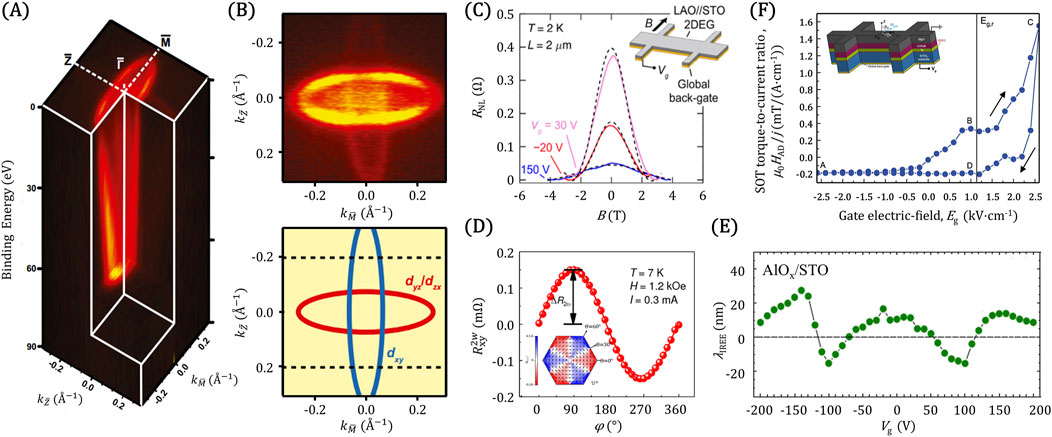
Figure 2. Electronic structure and charge-spin interconversion in the 2DEG at the surface/interface of SrTiO3: (A) Full photoemission mapping and (B) constant energy cuts and schematic constant-energy surfaces of the SrTiO3 2DEG measured by ARPES (Adapted with permission from Ref (Wang et al., 2014).); (C) Measurement of the spin Hall effect and inverse spin Hall effect in the 2DEG through nonlocal transport, and the voltage-controlled Hanle effect can be observed by applying an external magnetic field (Reproduced with permission from Ref (Trier et al., 2020).); (D) Angular dependence of the second harmonic planar Hall resistance
In view of the novel spin configurations exhibited by the SrTiO3 2DEG, its charge-spin interconversion properties have attracted great interest. Lesne et al. (2016) observed the inverse Rashba-Edelstein effect in the SrTiO3/LaAlO3 interface 2DEG by SP-FMR method at room temperature, quantifying the two-dimensional charge-spin conversion efficiency of the interface as
Building upon these findings. Lesne et al. (2016) not only measured a large charge-spin interconversion efficiency at the SrTiO3/LaAlO3 interface but also demonstrated the ability to tune the conversion efficiency at the interface using gate voltage. This voltage-driven tunability was previously observed by Ben Shalom et al. (2010) and Caviglia et al. (2010), who found that applying an external voltage enables effective modulation of the charge-spin interconversion. These discoveries underscore the potential for highly efficient and flexibly tunable performance in spintronic devices based on the SrTiO3 surface 2DEG. Vaz et al. (2019) observed the corresponding change of
The aforementioned research results confirm that the SrTiO3 interface 2DEG possesses significant and highly tunable charge-spin interconversion, which can be closely related to its novel electronic structure. In particular, the huge conversion efficiency recently observed at the SrTiO3/LaTiO3+δ interface indicates that the SOT properties of the 2DEG system still have great room for enhancement. In view of the intricate physical mechanisms involved, how to profoundly understand the influence of non-trivial electronic structures on charge-spin interconversion, and how to induce, characterize, and utilize novel topological states in a targeted manner to improve conversion efficiency are important research directions. It is noteworthy that current work primarily focuses on characterizing the charge-spin conversion efficiency. Given the enormous application potential of the SrTiO3 system, future research should place more emphasis on SOT manipulation of magnetization switching and developing functional spintronic devices.
3.2 4d transition metal oxides: Strontium ruthenate
4
Theoretical calculations predict that the band structure of SrRuO3 has multiple linear dispersive band crossing points near the Fermi surface, as shown in Figure 3A. These configurations, composed of
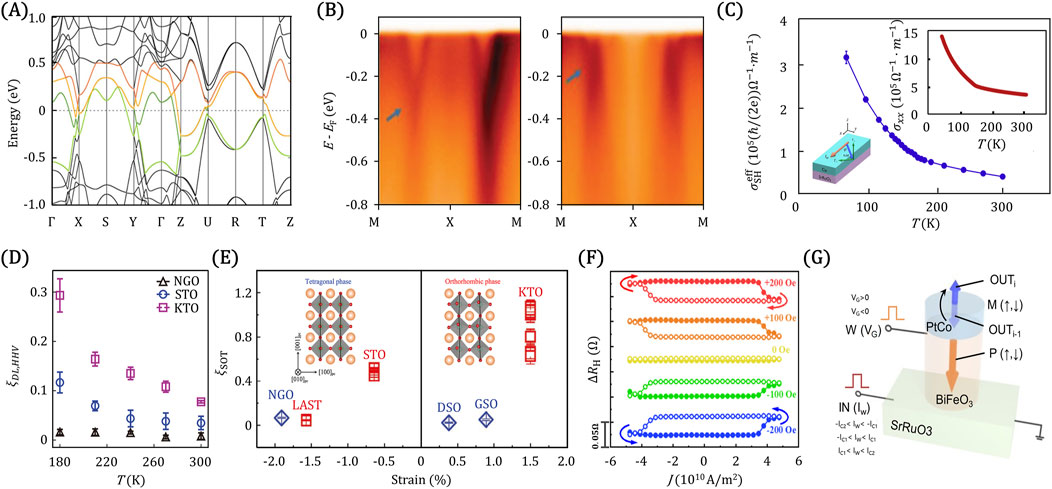
Figure 3. Electronic structures, spin transport properties and SOT associated characterization results of SrRuO3: (A) Band structure of SrRuO3 based on GGA calculations, considering the effects of magnetism, SOC and Coulomb interaction (Reproduced with permission from Ref (Takiguchi et al., 2020).); (B) Energy momentum mappings for SrRuO3(001) measured by ARPES. The Wely point are indicated with arrows (Reproduced with permission from Ref (Lin et al., 2021).); (C) Spin Hall conductivity of SrRuO3 as a function of temperature ranging from 300 to 60 K (inset: the corresponding electrical conductivity in the same temperature range) (Adapted with permission from Ref (Ou et al., 2019).); (D) Charge-spin conversion efficiency of SrRuO3 grown on various substrates as a function of temperature (Reproduced with permission from Ref (Zhou et al., 2021).); (E) Comparison of charge-spin conversion efficiency between orthorhombic (red square) and tetragonal (blue diamond) SrRuO3 grown on various substrates (Adapted with permission from Ref (Wei et al., 2021).); (F) Magnetization switching driven by pulse current under different external magnetic fields
The Berry curvature in the electronic bands of SrRuO3 not only contributes to the intrinsic anomalous Hall effect, but can also produce the spin Hall effect, thus bringing significant charge-spin interconversion. Haidar et al. (2015) measured the voltage signal generated by SrTiO3(001)/La0.67Sr0.33MnO3(LSMO)/SrRuO3 at room temperature by the spin pumping-ferromagnetic resonance (SP-FMR) method. They found a component that has a
The above studies demonstrate well the picture that the novel electronic structure of SrRuO3 is the source of its spin transport properties. However, compared to the large Berry curvature reflected by the anomalous Hall effect, the Berry curvature reflected by the inverse spin Hall effect is not significant. This contradiction is most likely due to the inevitable parasitic rectification effect in the SP-FMR experiment leading to an underestimation of the inverse spin Hall voltage (Mosendz et al., 2010). Therefore, recent related work has focused on characterizing the SOT generated by the spin Hall effect of SrRuO3 to more accurately and deeply investigate its charge-spin interconversion. Ou et al. (2019) systematically characterized the spin Hall conductivity in Si/SrTiO3/SrRuO3/Co by the spin torque-ferromagnetic resonance (ST-FMR) method. As shown in Figure 3C, the spin Hall conductivity shows a monotonic increase in the temperature range from 300 K to 60 K, up to 3 × 105
In addition to discussing the charge-spin conversion. Ou et al. (2019) also observed a vertical spin polarization component below the Curie temperature and pointed out that it may be related to the strong anisotropic magnetoresistance of SrRuO3. Moreover, combined with the characterization results of the crystal structure, they found that the spin Hall effect strength of SrRuO3 is closely related to the degree of rotation of the RuO6 oxygen octahedra, suggesting that enhanced conversion efficiency could be achieved through structural regulation. Recently. Zhou et al. (2021) and Wei et al. (2021) systematically studied the evolution of the SOT-related properties of SrRuO3 under different strain and crystal structures by regulating the crystal structure of SrRuO3 through epitaxial strain. They found that by applying −1.9% to +1.5% epitaxial strain to SrRuO3 through varying a series of substrates, its crystal structure undergoes changes in the tetragonal and orthorhombic phases, greatly affecting its charge-spin conversion efficiency. In the work of Zhou et al. (2021), as shown in Figure 3D, under different strain states, the conversion efficiency of SrRuO3 increases monotonically with decreasing temperature, indicating that the intrinsic spin Hall effect is the main contribution source, combined with the measurement of the change in conductivity. When the SrRuO3 thin films are grown on NdGaO3 substrates, the RuO6 octahedra only rotate out-of-plane, resulting in a tetragonal crystal structure with a conversion efficiency of
SrRuO3 exhibits significant and highly tunable charge-spin conversion efficiency, and further utilizing the generated SOT to drive magnetization switching is a key step for spin-based data storage and logic. Tang et al. (2022) designed an SrRuO3/FeGd heterostructure and achieved SOT-driven magnetization switching at room temperature with a low threshold current density of 4.5×106
Furthermore, Chai et al., (2023) designed a magnon-mediated spin torque (MST) device by constructing an SrRuO3/BiFeO3/CoPt multilayer heterostructure, demonstrating effective spin transmission and magnetization switching. By applying current pulses to the SrRuO3 layer, spin accumulation is generated at the interface, exciting magneton modes in BiFeO3. When the magnons transmit to the CoPt layer, the magnetization direction is controlled through magnon-mediated spin torque (MST), realizing parallel non-volatile writing of multiple storage units. As shown in Figure 3G, by applying different VG and
The above SOT results confirm that SrRuO3 possesses significant Berry curvature and strong spin Hall effect, and can achieve efficient charge-spin interconversion. Further regulating the electronic structure of SrRuO3 through strain engineering greatly enhances its charge-spin conversion efficiency, surpassing traditional heavy metals. In addition, considering that SrRuO3 also possesses excellent thermal stability, chemical stability, and high conductivity, as well as good compatibility with magnetic metals and oxides, it is a very promising spin source material. However, regarding the efficiency of SrRuO3’s charge-spin interconversion and its evolution with temperature, there are still some divergences at present. First, by comparing the results of Wahler et al. (2016) and Ou et al. (2019), it can be seen that in the non-magnetic temperature range, the spin Hall effect-related parameters of SrRuO3 increase with decreasing temperature, showing a consistent trend. However, below the Curie temperature (160 K), Wahler et al. observed a sharp decrease in the inverse spin Hall voltage, while Ou et al. found a continued increase in the spin Hall conductivity. This contradictory result concerns the influence of SrRuO3’s magnetism on its charge-spin interconversion capability, and needs to be further confirmed, with its underlying physical mechanism clarified. Second, although it is currently widely accepted that the source of SrRuO3’s efficient charge-spin interconversion is the significant Berry curvature brought about by its electronic band structure, there are still large discrepancies in the specific charge-spin conversion efficiency values. As shown in Table 1, in the same SrTiO3(001)/SrRuO3/Py structure, although there are some slight differences in the thickness of each layer, the conversion efficiency
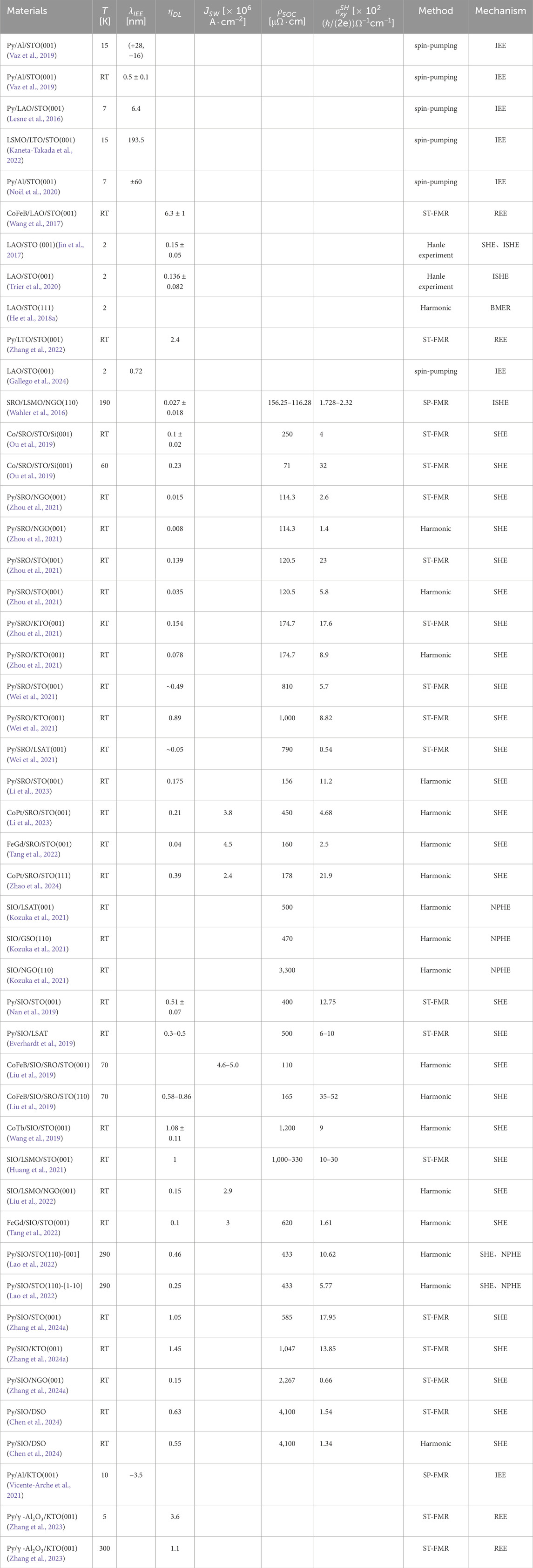
Table 1. Comparison of the SOT-efficiencies and spin Hall conductivity in transition metal oxide systems.
3.3 5d transition metal oxides
3.3.1 Strontium iridate
5
Theoretical studies predict the existence of a variety of correlated topological quantum states in perovskite-structured SrIrO3 thin films and heterostructures. Carter et al. (2012) employed tight-binding Hamiltonian model calculations to predict the existence of a three-dimensional topological nodal semimetal state in bulk perovskite-structured SrIrO3, which is protected by spin-orbit coupling and lattice symmetry. Kim et al. (2015) predicted the existence of a Dirac fermion state on the SrIrO3 (001) surface, with a non-trivial topological
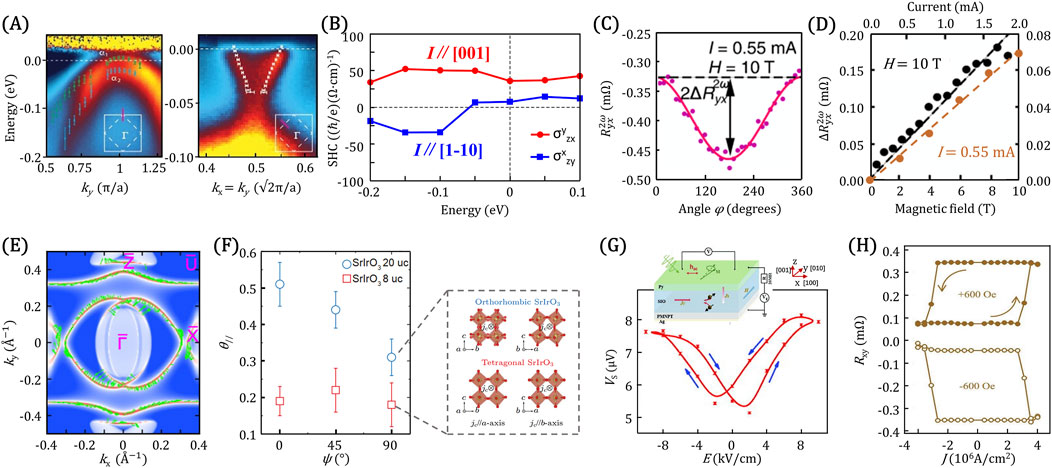
Figure 4. Electronic structures, spin transport properties and SOT associated characterization results of SrIrO3: (A) The electronic structure map near the Fermi surface measured by ARPES. left: the hole like bands near the Fermi surface. right: the linearly dispersive electron band near the Fermi surface (Reproduced with permission from Ref (Nie et al., 2015).); (B) Spin Hall conductivities for current
Regarding spin transport properties, Patri et al. (2018) conducted theoretical calculations on the spin Hall effect of bulk SrIrO3 and found that the combination of strong SOC and the Dirac nodal line band structure give rise to a huge Berry curvature, endowing SrIrO3 with significant intrinsic spin Hall conductivity, which can reach values on the order of 104
Beyond the aforementioned studies on linear charge-spin interconversion, Kozuka et al. (2021) observed a nonlinear planar Hall effect in SrIrO3, as shown in Figures 4C, D. The second harmonic Hall resistance
Given the high tunability of SrIrO3’s topological properties under the coupling of multiple degrees of freedom, it is evident that these properties can be effectively modulated through various means, such as epitaxial strain, elemental doping, and external field stimulus. This, in turn, allows for the regulation and enhancement of the charge-spin interconversion in the system, paving the way for the realization of high-performance SOT devices. As observed in the work of Nan et al. (2019), Liu et al. (2019), Lao et al. (2022), the conversion efficiency exhibits a strong dependence on the crystal orientation, with
In terms of spintronic applications, SrIrO3 has been proven to be capable of efficiently manipulating magnetic materials and achieving deterministic switching between different magnetization states. Liu et al. (2019) used the SOT generated by SrIrO3 to manipulate the magnetization state of SrRuO3 with perpendicular magnetic anisotropy at 70 K, and achieved deterministic switching without an external field by fine-tuning the easy axis angle of SrRuO3 through crystal structure engineering; Additionally, Liu et al. (2022) successfully switched the magnetic oxide LSMO with extremely low damping coefficient at room temperature through SrIrO3. The aforementioned SOT devices based on SrIrO3 only require a threshold current of 3-5 × 106
The above results confirm that SrIrO3 exhibits highly efficient and tunable charge-spin interconversion, holding promise for the realization of multifunctional spintronic devices. However, there are still some deficiencies in understanding the contribution of each mechanism to the charge-spin conversion efficiency. Firstly, it is currently widely believed that the damping-like efficiency of SrIrO3 originates from the spin Hall conductivity. However, based on the measurement results of SrIrO3/LSMO (Huang et al., 2021) and other topological materials (Mellnik et al., 2014; Li et al., 2018), as well as theoretical calculations (Amin and Stiles, 2016), spin-momentum locking also contributes to the damping-like efficiency, necessitating the clarification of the specific physical mechanisms. Secondly, although spin-momentum locking can contribute to the field-like efficiency, there is a large discrepancy in the actual
3.3.2 Potassium tantalate
Potassium tantalate (KTaO3) is a perovskite-structured insulating material with tantalum ions in its oxygen octahedra exhibiting a
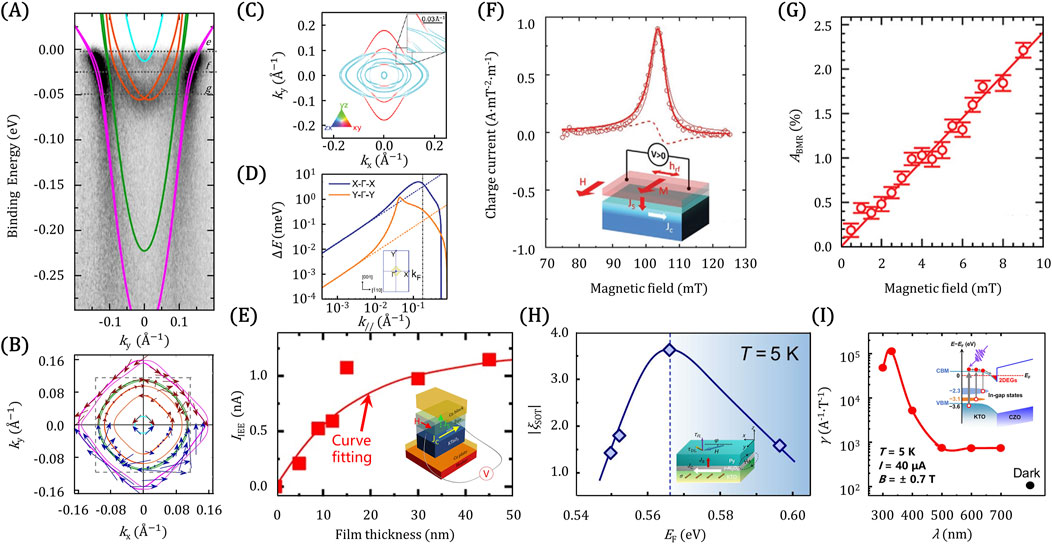
Figure 5. Electronic structures, spin transport properties and SOT-related characterization results of KTaO3: (A) ARPES results of surface 2DEG in KTaO3(001). The measured band structure is represented by colored lines: pink and green correspond to
Beyond the (001) orientation, Bruno et al. (2019) also observed similar electronic structure characteristics of 2DEG in KTaO3(111). However, due to the contribution of all
The strong spin-orbit coupling and unique electronic band structure of the KTaO3 interface 2DEG have sparked significant interest in exploring its charge-spin interconversion properties. Zhang et al. (2019b) successfully generated a 1
The significant SOC and complex carrier transport properties at the interface of KTaO3 make its electronic structure and charge-spin interconversion properties more sensitive and tunable. Zhang et al. (2023) not only obtained a large charge-spin conversion efficiency at the KTaO3(001)/γ-Al2O3 interface but also observed a strong dependence of the conversion efficiency on the band filling state at low temperatures (5 K) (Figure 5H). They controlled the Fermi level filling state by adjusting the thickness of γ-Al2O3. At low Fermi energies, only one type of carrier exists in the 2DEG, occupying the lower energy
Although the current research on the charge-spin interconversion of KTaO3 is still in its infancy, its excellent performance comparable to SrTiO3, indicates its enormous potential as a spin source material. Moreover, its rich and tunable electronic structure and charge-spin interconversion properties suggest that KTaO3 is an outstanding material for exploring novel quantum states and related new spintronic devices.
4 Outlook
Compared to the heavy metal systems, which have been extensively studied as spin source materials in early SOT devices, transition metal oxides have rapidly gained significant attention in this field. The 3
Since recent research has focused on only a few materials, there are still many systems with great potential in the vast transition metal oxide family that await further exploration. By designing oxide heterostructures and exploiting the ability of interfaces to control crystal structure, magnetoelectric properties, and electronic structure, researchers expect to significantly influence and enhance charge-spin interconversion (Ramesh and Schlom, 2019; Hwang et al., 2012). This can be achieved, for example, by inducing spin transport properties that cannot be present in a single bulk phase through the use of multi-component superlattices, or by maintaining high conversion efficiency up to room temperature in 2DEG systems via interface design. Moreover, by engineering the symmetry of complex oxide systems, the spin orientation of the generated spin current can be effectively manipulated, enabling achieve all-electric switching of the magnetization state, which is a crucial step toward practical applications. In heavy metals and two-dimensional materials, constructing symmetry-broken structures, such as creating vertical or lateral material composition gradients, incorporating antiferromagnets, or using ferroelectric materials (Yu et al., 2014; Oh et al., 2016; Zheng et al., 2021), can generate spin currents with out-of-plane spin polarization. This allows for deterministic switching of perpendicular moments without the need of an external magnetic field, which is essential for the realization of low-power, high-density SOT devices. In transition metal oxides, the spin-momentum locking in the surface state of SrTiO3(111) 2DEG has an out-of-plane spin polarization component (He et al., 2018a), making it promising system for realizing deterministic switching of perpendicular moments without an external field. Although there are no relevant reports in other oxides yet, it is feasible to influence the symmetry by fine-tuning the material composition and crystal structure during the growth process, and related research should also be carried out systematically in the future. Furthermore, substituting the
In addition to expanding the range of materials, researchers anticipate achieving precise control over SOT strength in transition metal oxide thin films and heterostructures by leveraging the characteristics of multi degrees of freedom coupling. The aforementioned spin Hall effect and surface states contribute differently to the damping-like torque and field-like torque of spin-orbit torque, and the ratio of these two components can significantly affect the energy consumption and response time of the magnetization switching process, domain wall motion velocity, and other dynamic processes (Katine et al., 2000; Legrand et al., 2015; Yoon et al., 2017; Liu et al., 2021; Taniguchi et al., 2015; Gomonay et al., 2016; Zhu and Zhao, 2020). Therefore, by influencing the electronic structure through multiple degrees of freedom, precise control of the strength and direction of the damping-like torque and field-like torque, respectively, is an important research direction for realizing high-performance SOT devices.
Finally, establishing the correlation between the electronic structure of oxides and charge-spin interconversion is crucial for understanding and controlling device performance. Currently, in the 2DEG of the 3
Author contributions
YH: Writing–review and editing, Writing–original draft. BL: Writing–review and editing. XZ: Writing–review and editing. SL: Writing–review and editing. R-WL: Writing–review and editing. ZW: Writing–review and editing, Writing–original draft, Funding acquisition.
Funding
The author(s) declare that financial support was received for the research, authorship, and/or publication of this article. This work was supported by the National Key Research and Development Program of China (Nos 2019YFA0307800 and 2017YFA0303600), the National Natural Science Foundation of China (Nos 12174406, 11874367, 51931011, and 52127803), the Ningbo Key Scientific and Technological Project (Grant No. 2022Z094), the Ningbo Natural Science Foundation (No. 2023J411).
Conflict of interest
The authors declare that the research was conducted in the absence of any commercial or financial relationships that could be construed as a potential conflict of interest.
Publisher’s note
All claims expressed in this article are solely those of the authors and do not necessarily represent those of their affiliated organizations, or those of the publisher, the editors and the reviewers. Any product that may be evaluated in this article, or claim that may be made by its manufacturer, is not guaranteed or endorsed by the publisher.
References
Agarwal, S., Aimone, B., Akinaga, H., Akinola, O., Badaroglu, M., Bersuker, G., et al. (2021). Beyond CMOS 2018. Int. Roadmap For Devices And Syst., 1–129. doi:10.1109/IRDS54852.2021.00011
Amin, V. P., and Stiles, M. D. (2016). Spin transport at interfaces with spin-orbit coupling: phenomenology. Phys. Rev. B 94 (10), 104420. doi:10.1103/PhysRevB.94.104420
Armitage, N. P., Mele, E. J., and Vishwanath, A. (2018). Weyl and Dirac semimetals in three-dimensional solids. Rev. Mod. Phys. 90 (1), 015001. doi:10.1103/RevModPhys.90.015001
Bai, H., Han, L., Feng, X. Y., Zhou, Y., Su, R., Wang, Q., et al. (2022). Observation of spin splitting torque in a collinear antiferromagnet RuO2. Phys. Rev. Lett. 128 (19), 197202. doi:10.1103/PhysRevLett.128.197202
Ben Shalom, M., Sachs, M., Rakhmilevitch, D., Palevski, A., and Dagan, Y. (2010). Tuning spin-orbit coupling and superconductivity at the SrTiO3/LaAlO3 interface: a magnetotransport study. Phys. Rev. Lett. 104 (12), 126802. doi:10.1103/PhysRevLett.104.126802
Berger, L. (1996). Emission of spin waves by a magnetic multilayer traversed by a current. Phys. Rev. B 54 (13), 9353–9358. doi:10.1103/PhysRevB.54.9353
Bose, A., Schreiber, N. J., Jain, R., Shao, D. F., Nair, H. P., Sun, J., et al. (2022). Tilted spin current generated by the collinear antiferromagnet ruthenium dioxide. Nat. Electron. 5 (5), 267–274. doi:10.1038/s41928-022-00744-8
Bruno, F. Y., McKeown Walker, S., Riccò, S., de la Torre, A., Wang, Z., Tamai, A., et al. (2019). Band structure and spin–orbital texture of the (111)-KTaO3 2D electron gas. Adv. Electron. Mater. 5 (5). doi:10.1002/aelm.201800860
Carter, J.-M., Shankar, V. V., Zeb, M. A., and Kee, H. Y. (2012). Semimetal and topological insulator in perovskite iridates. Phys. Rev. B 85 (11), 115105. doi:10.1103/PhysRevB.85.115105
Caviglia, A. D., Gabay, M., Gariglio, S., Reyren, N., Cancellieri, C., and Triscone, J. M. (2010). Tunable Rashba spin-orbit interaction at oxide interfaces. Phys. Rev. Lett. 104 (12), 126803. doi:10.1103/PhysRevLett.104.126803
Chai, Y., Liang, Y., Xiao, C., Wang, Y., Li, B., Jiang, D., et al. (2023). Multiferroic magnon spin-torque based reconfigurable logic-in-memory. arXiv Prepr. arXiv:2309.14614.
Chen, H., Jiang, D., Zhang, Q., Liang, Y., Liu, J., Tang, A., et al. (2024). Tuning stoichiometry for enhanced spin-charge interconversion in transition metal oxides. Adv. Electron. Mater. 10 (4), 2300666. doi:10.1002/aelm.202300666
Chen, H., and Yi, D. (2021). Spin–charge conversion in transition metal oxides. Apl. Mater. 9 (6). doi:10.1063/5.0052304
Chen, Y., Bergman, D. L., and Burkov, A. A. (2013). Weyl fermions and the anomalous Hall effect in metallic ferromagnets. Phys. Rev. B 88 (12), 125110. doi:10.1103/PhysRevB.88.125110
Chen, Y., Lu, Y.-M., and Kee, H.-Y. (2015). Topological crystalline metal in orthorhombic perovskite iridates. Nat. Commun. 6 (1), 6593. doi:10.1038/ncomms7593
Chen, Z., Liu, Y., Zhang, H., Liu, Z., Tian, H., Sun, Y., et al. (2021). Electric field control of superconductivity at the LaAlO3/KTaO3(111) interface. Science 372 (6543), 721–724. doi:10.1126/science.abb3848
Cui, D., Xu, Y., Zhou, L., Zhang, L., Luan, Z., Li, C., et al. (2021). Electrically tunable inverse spin Hall effect in SrIrO3/Pb(Mg1/3Nb2/3)0.7 Ti0.3O3 heterostructures through interface strain coupling. Appl. Phys. Lett. 118 (5), 052904. doi:10.1063/5.0027125
Dieny, B., Prejbeanu, I. L., Garello, K., Gambardella, P., Freitas, P., Lehndorff, R., et al. (2020). Opportunities and challenges for spintronics in the microelectronics industry. Nat. Electron. 3 (8), 446–459. doi:10.1038/s41928-020-0461-5
Dyrdał, A., Barnaś, J., and Fert, A. (2020). Spin-momentum-locking inhomogeneities as a source of bilinear magnetoresistance in topological insulators. Phys. Rev. Lett. 124 (4), 046802. doi:10.1103/PhysRevLett.124.046802
Everhardt, A. S., Dc, M., Huang, X., Sayed, S., Gosavi, T. A., Tang, Y., et al. (2019). Tunable charge to spin conversion in strontium iridate thin films. Phys. Rev. Mater. 3 (5), 051201. doi:10.1103/PhysRevMaterials.3.051201
Fang, Z., Nagaosa, N., Takahashi, K. S., Asamitsu, A., Mathieu, R., Ogasawara, T., et al. (2003). The anomalous Hall effect and magnetic monopoles in momentum space. Science 302 (5642), 92–95. doi:10.1126/science.1089408
Fujioka, J., Yamada, R., Kawamura, M., Sakai, S., Hirayama, M., Arita, R., et al. (2019). Strong-correlation induced high-mobility electrons in Dirac semimetal of perovskite oxide. Nat. Commun. 10 (1), 362. doi:10.1038/s41467-018-08149-y
Gallego, F., Tornos, J., Beltran, J. I., Peralta, A., Garcia-Barriocanal, J., Yu, G., et al. (2023). Reversible metal-insulator transition in SrIrO3 ultrathin layers by field effect control of inversion symmetry breaking. Commun. Mater. 4 (1), 36. doi:10.1038/s43246-023-00362-7
Gallego, F., Trier, F., Mallik, S., Bréhin, J., Varotto, S., Moreno Vicente-Arche, L., et al. (2024). All-electrical detection of the spin-charge conversion in nanodevices based on SrTiO3 2-D electron gases. Adv. Funct. Mater. 34 (3), 2307474. doi:10.1002/adfm.202307474
Gan, Y., Yang, F., Kong, L., Chen, X., Xu, H., Zhao, J., et al. (2023). Light-induced giant Rashba spin–orbit coupling at superconducting KTaO3(110) heterointerfaces. Adv. Mater. 35 (25), 2300582. doi:10.1002/adma.202300582
Gomonay, O., Jungwirth, T., and Sinova, J. (2016). High antiferromagnetic domain wall velocity induced by néel spin-orbit torques. Phys. Rev. Lett. 117 (1), 017202. doi:10.1103/PhysRevLett.117.017202
González-Hernández, R., Šmejkal, L., Výborný, K., Yahagi, Y., Sinova, J., Jungwirth, T., et al. (2021). Efficient electrical spin splitter based on nonrelativistic collinear antiferromagnetism. Phys. Rev. Lett. 126 (12), 127701. doi:10.1103/PhysRevLett.126.127701
Grezes, C., Kandazoglou, A., Cosset-Cheneau, M., Arche, L. M. V., Noël, P., Sgarro, P., et al. (2023). Non-volatile electric control of spin-orbit torques in an oxide two-dimensional electron gas. Nat. Commun. 14 (1), 2590. doi:10.1038/s41467-023-37866-2
Grigera, S. A., Gegenwart, P., Borzi, R. A., Weickert, F., Schofield, A. J., Perry, R. S., et al. (2004). Disorder-sensitive phase formation linked to metamagnetic quantum criticality. Science 306 (5699), 1154–1157. doi:10.1126/science.1104306
Haidar, S. M., Shiomi, Y., Lustikova, J., and Saitoh, E. (2015). Enhanced inverse spin Hall contribution at high microwave power levels in La0.67Sr0.33MnO3/SrRuO3 epitaxial bilayers. Appl. Phys. Lett. 107 (15). doi:10.1063/1.4933379
He, P., Walker, S. M., Zhang, S. S. L., Bruno, F., Bahramy, M., Lee, J. M., et al. (2018a). Observation of out-of-plane spin texture in a SrTiO3(111) two-dimensional electron gas. Phys. Rev. Lett. 120 (26), 266802. doi:10.1103/PhysRevLett.120.266802
He, P., Zhang, S. S. L., Zhu, D., Liu, Y., Wang, Y., Yu, J., et al. (2018b). Bilinear magnetoelectric resistance as a probe of three-dimensional spin texture in topological surface states. Nat. Phys. 14 (5), 495–499. doi:10.1038/s41567-017-0039-y
He, P., Zhang, S. S. L., Zhu, D., Shi, S., Heinonen, O. G., Vignale, G., et al. (2019). Nonlinear planar Hall effect. Phys. Rev. Lett. 123 (1), 016801. doi:10.1103/PhysRevLett.123.016801
Huang, X., Sayed, S., Mittelstaedt, J., Susarla, S., Karimeddiny, S., Caretta, L., et al. (2021). Novel spin–orbit torque generation at room temperature in an all-oxide epitaxial La0.7Sr0.3MnO3/SrIrO3 system. Adv. Mater. 33 (24), e2008269. doi:10.1002/adma.202008269
Hwang, H. Y., Iwasa, Y., Kawasaki, M., Keimer, B., Nagaosa, N., and Tokura, Y. (2012). Emergent phenomena at oxide interfaces. Nat. Mater. 11 (2), 103–113. doi:10.1038/nmat3223
Imada, M., Fujimori, A., and Tokura, Y. (1998). Metal-insulator transitions. Rev. Mod. Phys. 70 (4), 1039–1263. doi:10.1103/RevModPhys.70.1039
Itoh, S., Endoh, Y., Yokoo, T., Ibuka, S., Park, J. G., Kaneko, Y., et al. (2016). Weyl fermions and spin dynamics of metallic ferromagnet SrRuO3. Nat. Commun. 7 (1), 11788. doi:10.1038/ncomms11788
Jadaun, P., Register, L. F., and Banerjee, S. K. (2020). Rational design principles for giant spin Hall effect in 5d -transition metal oxides. Proc. Natl. Acad. Sci. 117 (22), 11878–11886. doi:10.1073/pnas.1922556117
Jin, M.-J., Moon, S. Y., Park, J., Modepalli, V., Jo, J., Kim, S. I., et al. (2017). Nonlocal spin diffusion driven by giant spin Hall effect at oxide heterointerfaces. Nano Lett. 17 (1), 36–43. doi:10.1021/acs.nanolett.6b03050
Kaneta-Takada, S., Kitamura, M., Arai, S., Arai, T., Okano, R., Anh, L. D., et al. (2022). Giant spin-to-charge conversion at an all-epitaxial single-crystal-oxide Rashba interface with a strongly correlated metal interlayer. Nat. Commun. 13 (1), 5631. doi:10.1038/s41467-022-33350-5
Katine, J. A., Albert, F. J., Buhrman, R. A., Myers, E. B., and Ralph, D. C. (2000). Current-driven magnetization reversal and spin-wave excitations in Co/Cu/Co pillars. Phys. Rev. Lett. 84 (14), 3149–3152. doi:10.1103/PhysRevLett.84.3149
Kim, B. J., Jin, H., Moon, S. J., Kim, J.-Y., Park, B. G., Leem, C. S., et al. (2008). Novel eff=1/2 Mott state induced by relativistic spin-orbit coupling in Sr2IrO4. Phys. Rev. Lett. 101 (7), 076402. doi:10.1103/PhysRevLett.101.076402
Kim, H.-S., Chen, Y., and Kee, H.-Y. (2015). Surface states of perovskite iridates AIrO3: signatures of a topological crystalline metal with nontrivial Z2 index. Phys. Rev. B 91 (23), 235103. doi:10.1103/PhysRevB.91.235103
King, P. D. C., He, R. H., Eknapakul, T., Buaphet, P., Mo, S. K., Kaneko, Y., et al. (2012). Subband structure of a two-dimensional electron gas formed at the polar surface of the strong spin-orbit perovskite KTaO3. Phys. Rev. Lett. 108 (11), 117602. doi:10.1103/PhysRevLett.108.117602
King, P. D. C., McKeown Walker, S., Tamai, A., de la Torre, A., Eknapakul, T., Buaphet, P., et al. (2014). Quasiparticle dynamics and spin–orbital texture of the SrTiO3 two-dimensional electron gas. Nat. Commun. 5 (1), 3414. doi:10.1038/ncomms4414
Koster, G., Klein, L., Siemons, W., Rijnders, G., Dodge, J. S., Eom, C. B., et al. (2012). Structure, physical properties, and applications of SrRuO3 thin films. Rev. Mod. Phys. 84 (1), 253–298. doi:10.1103/RevModPhys.84.253
Kozuka, Y., Isogami, S., Masuda, K., Miura, Y., Das, S., Fujioka, J., et al. (2021). Observation of nonlinear spin-charge conversion in the thin film of nominally centrosymmetric Dirac semimetal SrIrO3 at room temperature. Phys. Rev. Lett. 126 (23), 236801. doi:10.1103/PhysRevLett.126.236801
Kumar, D., Hsu, C.-H., Sharma, R., Chang, T. R., Yu, P., Wang, J., et al. (2021). Room-temperature nonlinear Hall effect and wireless radiofrequency rectification in Weyl semimetal TaIrTe4. Nat. Nanotechnol. 16 (4), 421–425. doi:10.1038/s41565-020-00839-3
Lao, B., Liu, P., Zheng, X., Lu, Z., Li, S., Zhao, K., et al. (2022). Anisotropic linear and nonlinear charge-spin conversion in topological semimetal SrIrO3. Phys. Rev. B 106 (22), L220409. doi:10.1103/PhysRevB.106.L220409
Lee, J. H., Harada, T., Trier, F., Marcano, L., Godel, F., Valencia, S., et al. (2021). Nonreciprocal transport in a Rashba ferromagnet, delafossite PdCoO2. Nano Lett. 21 (20), 8687–8692. doi:10.1021/acs.nanolett.1c02756
Legrand, W., Ramaswamy, R., Mishra, R., and Yang, H. (2015). Coherent subnanosecond switching of perpendicular magnetization by the fieldlike spin-orbit torque without an external magnetic field. Phys. Rev. Appl. 3 (6), 064012. doi:10.1103/PhysRevApplied.3.064012
Lesne, E., Fu, Y., Oyarzun, S., Rojas-Sánchez, J. C., Vaz, D. C., Naganuma, H., et al. (2016). Highly efficient and tunable spin-to-charge conversion through Rashba coupling at oxide interfaces. Nat. Mater. 15 (12), 1261–1266. doi:10.1038/nmat4726
Li, J., Wang, J., Wuttig, M., Ramesh, R., Wang, N., Ruette, B., et al. (2004). Dramatically enhanced polarization in (001), (101), and (111) BiFeO3 thin films due to epitiaxial-induced transitions. Appl. Phys. Lett. 84 (25), 5261–5263. doi:10.1063/1.1764944
Li, P., Wu, W., Wen, Y., Zhang, C., Zhang, J., Zhang, S., et al. (2018). Spin-momentum locking and spin-orbit torques in magnetic nano-heterojunctions composed of Weyl semimetal WTe2. Nat. Commun. 9 (1), 3990. doi:10.1038/s41467-018-06518-1
Li, S., Lao, B., Lu, Z., Zheng, X., Zhao, K., Gong, L., et al. (2023). Room temperature spin-orbit torque efficiency and magnetization switching in SrRuO3-based heterostructures. Phys. Rev. Mater. 7 (2), 024418. doi:10.1103/PhysRevMaterials.7.024418
Lin, W., Liu, L., Liu, Q., Li, L., Shu, X., Li, C., et al. (2021). Electric field control of the magnetic Weyl fermion in an epitaxial SrRuO3 (111) thin film. Adv. Mater. 33 (36), e2101316. doi:10.1002/adma.202101316
Liu, C., Yan, X., Jin, D., Ma, Y., Hsiao, H. W., Lin, Y., et al. (2021b). Two-dimensional superconductivity and anisotropic transport at KTaO3 (111) interfaces. Science 371 (6530), 716–721. doi:10.1126/science.aba5511
Liu, J., Kriegner, D., Horak, L., Puggioni, D., Rayan Serrao, C., Chen, R., et al. (2016b). Strain-induced nonsymmorphic symmetry breaking and removal of Dirac semimetallic nodal line in an orthoperovskite iridate. Phys. Rev. B 93 (8), 085118. doi:10.1103/PhysRevB.93.085118
Liu, L., Qin, Q., Lin, W., Li, C., Xie, Q., He, S., et al. (2019). Current-induced magnetization switching in all-oxide heterostructures. Nat. Nanotechnol. 14 (10), 939–944. doi:10.1038/s41565-019-0534-7
Liu, L., Zhou, G., Shu, X., Li, C., Lin, W., Ren, L., et al. (2022). Room-temperature spin-orbit torque switching in a manganite-based heterostructure. Phys. Rev. B. 105 (14), 144419. doi:10.1103/PhysRevB.105.144419
Liu, Y.-T., Huang, C.-C., Chen, K.-H., Huang, Y. H., Tsai, C. C., Chang, T. Y., et al. (2021a). Anatomy of type-x spin-orbit-torque switching. Phys. Rev. Appl. 16 (2), 024021. doi:10.1103/PhysRevApplied.16.024021
Liu, Z. T., Li, M. Y., Li, Q. F., Liu, J. S., Li, W., Yang, H. F., et al. (2016a). Direct observation of the Dirac nodes lifting in semimetallic perovskite SrIrO3 thin films. Sci. Rep. 6 (1), 30309. doi:10.1038/srep30309
Luke, G. M., Fudamoto, Y., Kojima, K. M., Larkin, M. I., Merrin, J., Nachumi, B., et al. (1998). Time-reversal symmetry-breaking superconductivity in Sr2RuO4. Nature 394 (6693), 558–561. doi:10.1038/29038
Ma, Q., Xu, S.-Y., Shen, H., MacNeill, D., Fatemi, V., Chang, T. R., et al. (2018). Observation of the nonlinear Hall effect under time-reversal-symmetric conditions. Nature 565 (7739), 337–342. doi:10.1038/s41586-018-0807-6
Manchon, A., Koo, H. C., Nitta, J., Frolov, S. M., and Duine, R. A. (2015). New perspectives for Rashba spin–orbit coupling. Nat. Mater. 14 (9), 871–882. doi:10.1038/nmat4360
Manchon, A., Železný, J., Miron, I. M., Jungwirth, T., Sinova, J., Thiaville, A., et al. (2019). Current-induced spin-orbit torques in ferromagnetic and antiferromagnetic systems. Rev. Mod. Phys. 91 (3), 035004. doi:10.1103/RevModPhys.91.035004
Manipatruni, S., Nikonov, D. E., and Young, I. A. (2018). Beyond CMOS computing with spin and polarization. Nat. Phys. 14 (4), 338–343. doi:10.1038/s41567-018-0101-4
Martínez, E. A., Dai, J., Tallarida, M., Nemes, N. M., and Bruno, F. Y (2023). Anisotropic electronic structure of the 2D electron gas at the AlOx/KTaO3(110) interface. Adv. Electron. Mater. 9 (10), 2300267. doi:10.1002/aelm.202300267
Mathieu, R., Asamitsu, A., Yamada, H., Takahashi, K. S., Kawasaki, M., Fang, Z., et al. (2004). Scaling of the anomalous Hall effect in Sr1−xCaxRuO3. Phys. Rev. Lett. 93 (1), 016602. doi:10.1103/PhysRevLett.93.016602
Mellnik, A. R., Lee, J. S., Richardella, A., Grab, J. L., Mintun, P. J., Fischer, M. H., et al. (2014). Spin-transfer torque generated by a topological insulator. Nature 511(7510): 449–451. doi:10.1038/nature13534
Moon, S. J., Jin, H., Kim, K. W., Choi, W. S., Lee, Y. S., Yu, J., et al. (2008). Dimensionality-controlled insulator-metal transition and correlated metallic state in 5d transition metal oxides Srn+1IrnO3n+1(n=1, 2, and ∞). Phys. Rev. Lett. 101 (22), 226402. doi:10.1103/PhysRevLett.101.226402
Mosendz, O., Pearson, J. E., Fradin, F. Y., Bauer, G. E. W., Bader, S. D., and Hoffmann, A. (2010). Quantifying spin Hall angles from spin pumping: experiments and theory. Phys. Rev. Lett. 104 (4), 046601. doi:10.1103/PhysRevLett.104.046601
Nakamura, H., and Kimura, T. (2009). Electric field tuning of spin-orbit coupling in KTaO3 field-effect transistors. Phys. Rev. B 80 (12), 121308. doi:10.1103/PhysRevB.80.121308
Nan, T., Anderson, T. J., Gibbons, J., Hwang, K., Campbell, N., Zhou, H., et al. (2019). Anisotropic spin-orbit torque generation in epitaxial SrIrO3 by symmetry design. Proc. Natl. Acad. Sci. 116 (33), 16186–16191. doi:10.1073/pnas.1812822116
Nie, Y. F., King, P. D. C., Kim, C. H., Uchida, M., Wei, H., Faeth, B., et al. (2015). Interplay of spin-orbit interactions, dimensionality, and octahedral rotations in semimetallic SrIrO3. Phys. Rev. Lett. 114 (1), 016401. doi:10.1103/PhysRevLett.114.016401
Noël, P., Trier, F., Vicente Arche, L. M., Bréhin, J., Vaz, D. C., Garcia, V., et al. (2020). Non-volatile electric control of spin–charge conversion in a SrTiO3 Rashba system. Nature 580 (7804), 483–486. doi:10.1038/s41586-020-2197-9
Oh, Y.-W., Chris Baek, S.-h., Kim, Y. M., Lee, H. Y., Lee, K. D., Yang, C. G., et al. (2016). Field-free switching of perpendicular magnetization through spin–orbit torque in antiferromagnet/ferromagnet/oxide structures. Nat. Nanotechnol. 11 (10), 878–884. doi:10.1038/nnano.2016.109
Ohtomo, A., and Hwang, H. Y. (2004). A high-mobility electron gas at the LaAlO3/SrTiO3 heterointerface. Nature 427 (6973), 423–426. doi:10.1038/nature02308
Ou, Y., Wang, Z., Chang, C. S., Nair, H. P., Paik, H., Reynolds, N., et al. (2019). Exceptionally high, strongly temperature dependent, spin Hall conductivity of SrRuO3. Nano Lett. 19 (6), 3663–3670. doi:10.1021/acs.nanolett.9b00729
Pai, Y.-Y., Tylan-Tyler, A., Irvin, P., and Levy, J. (2018). Physics of SrTiO3-based heterostructures and nanostructures: a review. Rep. Prog. Phys. 81 (3), 036503. doi:10.1088/1361-6633/aa892d
Patri, A. S., Hwang, K., Lee, H.-W., and Kim, Y. B. (2018). Theory of large intrinsic spin Hall effect in iridate semimetals. Sci. Rep. 8 (1), 8052. doi:10.1038/s41598-018-26355-y
Ramesh, R., and Schlom, D. G. (2019). Creating emergent phenomena in oxide superlattices. Nat. Rev. Mater. 4 (4), 257–268. doi:10.1038/s41578-019-0095-2
Rao, W., Zhou, Y.-L., Wu, Y.-j., Duan, H. J., Deng, M. X., and Wang, R. Q. (2021). Theory for linear and nonlinear planar Hall effect in topological insulator thin films. Phys. Rev. B 103 (15), 155415. doi:10.1103/PhysRevB.103.155415
Ren, Z., Lao, B., Zheng, X., Liao, L., Lu, Z., Li, S., et al. (2022). Emergence of insulating ferrimagnetism and perpendicular magnetic anisotropy in 3d–5d perovskite oxide composite films for insulator spintronics. ACS Appl. Mater. and Interfaces 14 (13), 15407–15414. doi:10.1021/acsami.2c01849
Reyren, N., Thiel, S., Caviglia, A. D., Kourkoutis, L. F., Hammerl, G., Richter, C., et al. (2007). Superconducting interfaces between insulating oxides. Science 317 (5842), 1196–1199. doi:10.1126/science.1146006
Richter, T., Paleschke, M., Wahler, M., Heyroth, F., Deniz, H., Hesse, D., et al. (2017). Spin pumping and inverse spin Hall effect in ultrathin SrRuO3 films around the percolation limit. Phys. Rev. B 96 (18), 184407. doi:10.1103/PhysRevB.96.184407
Santander-Syro, A. F., Bareille, C., Fortuna, F., Copie, O., Gabay, M., Bertran, F., et al. (2012). Orbital symmetry reconstruction and strong mass renormalization in the two-dimensional electron gas at the surface of KTaO3. Phys. Rev. B 86 (12), 121107. doi:10.1103/PhysRevB.86.121107
Schilling, A., Cantoni, M., Guo, J. D., and Ott, H. R. (1993). Superconductivity above 130 K in the Hg–Ba–Ca–Cu–O system. Nature 363 (6424), 56–58. doi:10.1038/363056a0
Sinova, J., Culcer, D., Niu, Q., Sinitsyn, N. A., Jungwirth, T., and MacDonald, A. H. (2004). Universal intrinsic spin Hall effect. Phys. Rev. Lett. 92 (12), 126603. doi:10.1103/PhysRevLett.92.126603
Sinova, J., Valenzuela, S. O., Wunderlich, J., Back, C., and Jungwirth, T. (2015). Spin Hall effects. Rev. Mod. Phys. 87 (4), 1213–1260. doi:10.1103/RevModPhys.87.1213
Slonczewski, J. C. (1996). Current-driven excitation of magnetic multilayers. J. Magnetism Magnetic Mater. 159 (1), L1–L7. doi:10.1016/0304-8853(96)00062-5
Sodemann, I., and Fu, L. (2015). Quantum nonlinear Hall effect induced by Berry curvature dipole in time-reversal invariant materials. Phys. Rev. Lett. 115 (21), 216806. doi:10.1103/PhysRevLett.115.216806
Stemmer, S., and James Allen, S. (2014). Two-dimensional electron gases at complex oxide interfaces. Annu. Rev. Mater. Res. 44 (1), 151–171. doi:10.1146/annurev-matsci-070813-113552
Takiguchi, K., Wakabayashi, Y. K., Irie, H., Krockenberger, Y., Otsuka, T., Sawada, H., et al. (2020). Quantum transport evidence of Weyl fermions in an epitaxial ferromagnetic oxide. Nat. Commun. 11 (1), 4969. doi:10.1038/s41467-020-18646-8
Tang, A., Xu, T., Liu, S., Liang, Y., Chen, H., Yan, D., et al. (2022). Implementing complex oxides for efficient room-temperature spin–orbit torque switching. Adv. Electron. Mater. 8 (11), 2200514. doi:10.1002/aelm.202200514
Taniguchi, T., Mitani, S., and Hayashi, M. (2015). Critical current destabilizing perpendicular magnetization by the spin Hall effect. Phys. Rev. B 92 (2), 024428. doi:10.1103/PhysRevB.92.024428
Tian, D., Liu, Z., Shen, S., Li, Z., Zhou, Y., Liu, H., et al. (2021). Manipulating Berry curvature of SrRuO3 thin films via epitaxial strain. Proc. Natl. Acad. Sci. 118 (18), e2101946118. doi:10.1073/pnas.2101946118
To, D. Q., Dang, T. H., Vila, L., Attané, J. P., Bibes, M., and Jaffrès, H. (2021). Spin to charge conversion at Rashba-split SrTiO3 interfaces from resonant tunneling. Phys. Rev. Res. 3 (4), 043170. doi:10.1103/PhysRevResearch.3.043170
Tomioka, Y., Asamitsu, A., Moritomo, Y., and Tokura, Y. (1995). Anomalous magnetotransport properties of Pr1-xCaxMnO3. J. Phys. Soc. Jpn. 64 (10), 3626–3630. doi:10.1143/JPSJ.64.3626
Trier, F., Noël, P., Kim, J.-V., Attané, J. P., Vila, L., and Bibes, M. (2021). Oxide spin-orbitronics: spin–charge interconversion and topological spin textures. Nat. Rev. Mater. 7 (4), 258–274. doi:10.1038/s41578-021-00395-9
Trier, F., Prawiroatmodjo, G. E. D. K., Zhong, Z., Christensen, D. V., von Soosten, M., Bhowmik, A., et al. (2016). Quantization of Hall resistance at the metallic interface between an oxide insulator and SrTiO3. Phys. Rev. Lett. 117 (9), 096804. doi:10.1103/PhysRevLett.117.096804
Trier, F., Vaz, D. C., Bruneel, P., Noël, P., Fert, A., Vila, L., et al. (2020). Electric-field control of spin current generation and detection in ferromagnet-free SrTiO3-based nanodevices. Nano Lett. 20 (1), 395–401. doi:10.1021/acs.nanolett.9b04079
van Benthem, K., Elsässer, C., and French, R. H. (2001). Bulk electronic structure of SrTiO3: experiment and theory. J. Appl. Phys. 90 (12), 6156–6164. doi:10.1063/1.1415766
Varotto, S., Johansson, A., Göbel, B., Vicente-Arche, L. M., Mallik, S., Bréhin, J., et al. (2022). Direct visualization of Rashba-split bands and spin/orbital-charge interconversion at KTaO3 interfaces. Nat. Commun. 13 (1), 6165. doi:10.1038/s41467-022-33621-1
Vaz, D. C., Noël, P., Johansson, A., Göbel, B., Bruno, F. Y., Singh, G., et al. (2019). Mapping spin–charge conversion to the band structure in a topological oxide two-dimensional electron gas. Nat. Mater. 18 (11), 1187–1193. doi:10.1038/s41563-019-0467-4
Vicente-Arche, L. M., Bréhin, J., Varotto, S., Cosset-Cheneau, M., Mallik, S., Salazar, R., et al. (2021). Spin–charge interconversion in KTaO3 2D electron gases. Adv. Mater. 33 (43), e2102102. doi:10.1002/adma.202102102
Vrejoiu, I., Le Rhun, G., Pintilie, L., Hesse, D., Alexe, M., and Gösele, U. (2006). Intrinsic ferroelectric properties of strained tetragonal PbZr0.2Ti0.8O3 obtained on layer–by–layer grown, defect–free single–crystalline films. Adv. Mater. 18 (13), 1657–1661. doi:10.1002/adma.200502711
Wadehra, N., Tomar, R., Varma, R. M., Gopal, R. K., Singh, Y., Dattagupta, S., et al. (2020). Planar Hall effect and anisotropic magnetoresistance in polar-polar interface of LaVO3-KTaO3 with strong spin-orbit coupling. Nat. Commun. 11 (1), 874. doi:10.1038/s41467-020-14689-z
Wahler, M., Homonnay, N., Richter, T., Müller, A., Eisenschmidt, C., Fuhrmann, B., et al. (2016). Inverse spin Hall effect in a complex ferromagnetic oxide heterostructure. Sci. Rep. 6 (1), 28727. doi:10.1038/srep28727
Wang, H., Meng, K.-Y., Zhang, P., Hou, J. T., Finley, J., Han, J., et al. (2019). Large spin-orbit torque observed in epitaxial SrIrO3 thin films. Appl. Phys. Lett. 114 (23). doi:10.1063/1.5097699
Wang, Y., Ramaswamy, R., Motapothula, M., Narayanapillai, K., Zhu, D., Yu, J., et al. (2017). Room-temperature giant charge-to-spin conversion at the SrTiO3–LaAlO3 oxide interface. Nano Lett. 17 (12), 7659–7664. doi:10.1021/acs.nanolett.7b03714
Wang, Z., Zhong, Z., Hao, X., Gerhold, S., Stöger, B., Schmid, M., et al. (2014). Anisotropic two-dimensional electron gas at SrTiO3(110). Proc. Natl. Acad. Sci. 111 (11), 3933–3937. doi:10.1073/pnas.1318304111
Wei, J., Zhong, H., Liu, J., Wang, X., Meng, F., Xu, H., et al. (2021). Enhancement of spin–orbit torque by strain engineering in SrRuO3 films. Adv. Funct. Mater. 31 (40). doi:10.1002/adfm.202100380
Witczak-Krempa, W., Chen, G., Kim, Y. B., and Balents, L. (2014). Correlated quantum phenomena in the strong spin-orbit regime. Annu. Rev. Condens. Matter Phys. 5 (1), 57–82. doi:10.1146/annurev-conmatphys-020911-125138
Xiao, D., Chang, M.-C., and Niu, Q. (2010). Berry phase effects on electronic properties. Rev. Mod. Phys. 82 (3), 1959–2007. doi:10.1103/RevModPhys.82.1959
Yan, B., and Felser, C. (2017). Topological materials: Weyl semimetals. Annu. Rev. Condens. Matter Phys. 8 (1), 337–354. doi:10.1146/annurev-conmatphys-031016-025458
Yasuda, K., Tsukazaki, A., Yoshimi, R., Kondou, K., Takahashi, K., Otani, Y., et al. (2017). Current-nonlinear Hall effect and spin-orbit torque magnetization switching in a magnetic topological insulator. Phys. Rev. Lett. 119 (13), 137204. doi:10.1103/PhysRevLett.119.137204
Yoon, J., Lee, S.-W., Kwon, J. H., Lee, J. M., Son, J., Qiu, X., et al. (2017). Anomalous spin-orbit torque switching due to field-like torque–assisted domain wall reflection. Sci. Adv. 3 (4), e1603099. doi:10.1126/sciadv.1603099
Yu, G., Upadhyaya, P., Fan, Y., Alzate, J. G., Jiang, W., Wong, K. L., et al. (2014). Switching of perpendicular magnetization by spin–orbit torques in the absence of external magnetic fields. Nat. Nanotechnol. 9 (7), 548–554. doi:10.1038/nnano.2014.94
Yu, X.-Q., Zhu, Z.-G., and Su, G. (2021). Hexagonal warping induced nonlinear planar Nernst effect in nonmagnetic topological insulators. Phys. Rev. B 103 (3), 035410. doi:10.1103/PhysRevB.103.035410
Zhang, H., Ma, Y., Zhang, H., Chen, X., Wang, S., Li, G., et al. (2019b). Thermal spin injection and inverse Edelstein effect of the two-dimensional electron gas at EuO–KTaO3 interfaces. Nano Lett. 19 (3), 1605–1612. doi:10.1021/acs.nanolett.8b04509
Zhang, H., Yan, X., Zhang, X., Wang, S., Xiong, C., Zhang, H., et al. (2019a). Unusual electric and optical tuning of KTaO3-based two-dimensional electron gases with 5d orbitals. ACS Nano 13 (1), 609–615. doi:10.1021/acsnano.8b07622
Zhang, H., Yun, Y., Zhang, X., Zhang, H., Ma, Y., Yan, X., et al. (2018). High-mobility spin-polarized two-dimensional electron gases at EuO/KTaO3 interfaces. Phys. Rev. Lett. 121 (11), 116803. doi:10.1103/PhysRevLett.121.116803
Zhang, H., Zhang, H., Yan, X., Zhang, X., Zhang, Q., Zhang, J., et al. (2017). Highly mobile two-dimensional electron gases with a strong gating effect at the amorphous LaAlO3/KTaO3 interface. ACS Appl. Mater. and Interfaces 9 (41), 36456–36461. doi:10.1021/acsami.7b12814
Zhang, H., Zhu, Z., Zhu, Y., Chen, X., Jiang, Q., Wei, J., et al. (2023). Fermi-level-dependent charge-to-spin conversion of the two-dimensional electron gas at the γ-Al2O3/KTaO3 interface. Phys. Rev. Appl. 19 (3), 034045. doi:10.1103/PhysRevApplied.19.034045
Zhang, J., Zhang, J., Chi, X., Hao, R., Chen, W., Yang, H., et al. (2022). Giant efficiency for charge-to-spin conversion via the electron gas at the LaTiO3+δ/SrTiO3 interface. Phys. Rev. B 105 (19), 195110. doi:10.1103/PhysRevB.105.195110
Zhang, Q., Shi, S., Zheng, Z., Zhou, H., Shao, D. F., Zhao, T., et al. (2024a). Highly energy-efficient spin current generation in SrIrO3 by manipulating the octahedral rotation. ACS Appl. Mater. and Interfaces 16 (1), 1129–1136. doi:10.1021/acsami.3c15514
Zhang, S., Levy, P. M., and Fert, A. (2002). Mechanisms of spin-polarized current-driven magnetization switching. Phys. Rev. Lett. 88 (23), 236601. doi:10.1103/PhysRevLett.88.236601
Zhang, X., Zhu, T., Zhang, S., Chen, Z., Song, A., Zhang, C., et al. (2024b). Light-induced giant enhancement of nonreciprocal transport at KTaO3-based interfaces. Nat. Commun. 15 (1), 2992. doi:10.1038/s41467-024-47231-6
Zhao, K., Li, S., Lu, Z., Lao, B., Zheng, X., Li, R. W., et al. (2024). Crystal orientation regulation of spin-orbit torque efficiency and magnetization switching in SrRuO3 thin films. Acta Phys. Sin. 0 (0), 117701. doi:10.7498/aps.73.20240367
Zheng, Z., Zhang, Y., Lopez-Dominguez, V., Sánchez-Tejerina, L., Shi, J., Feng, X., et al. (2021). Field-free spin-orbit torque-induced switching of perpendicular magnetization in a ferrimagnetic layer with a vertical composition gradient. Nat. Commun. 12 (1), 4555. doi:10.1038/s41467-021-24854-7
Zhou, J., Shu, X., Lin, W., Shao, D. F., Chen, S., Liu, L., et al. (2021). Modulation of spin–orbit torque from SrRuO3 by epitaxial-strain-induced octahedral rotation. Adv. Mater. 33 (30), e2007114. doi:10.1002/adma.202007114
Zhu, D., and Zhao, W. (2020). Threshold current density for perpendicular magnetization switching through spin-orbit torque. Phys. Rev. Appl. 13 (4), 044078. doi:10.1103/PhysRevApplied.13.044078
Keywords: transition metal oxides, electronic structure, charge-spin interconversion, heterostructure engineering, spintronic devices
Citation: Han Y, Lao B, Zheng X, Li S, Li R-W and Wang Z (2024) Transition metal oxides: a new frontier in spintronics driven by novel quantum states and efficient charge-spin interconversion. Front. Mater. 11:1444769. doi: 10.3389/fmats.2024.1444769
Received: 06 June 2024; Accepted: 13 August 2024;
Published: 26 August 2024.
Edited by:
Milan Radovic, Paul Scherrer Institut (PSI), SwitzerlandReviewed by:
Jacobo Santamaria, Complutense University of Madrid, SpainShijing Gong, East China Normal University, China
Copyright © 2024 Han, Lao, Zheng, Li, Li and Wang. This is an open-access article distributed under the terms of the Creative Commons Attribution License (CC BY). The use, distribution or reproduction in other forums is permitted, provided the original author(s) and the copyright owner(s) are credited and that the original publication in this journal is cited, in accordance with accepted academic practice. No use, distribution or reproduction is permitted which does not comply with these terms.
*Correspondence: Zhiming Wang, emhpbWluZy53YW5nQG5pbXRlLmFjLmNu