- 1Department of Otolaryngology, Head and Neck Surgery, the Affiliated Hospital of Qingdao University, Qingdao, Shandong, China
- 2Department of Genetics and Cell Biology, Basic Medical College, Qingdao University, Qingdao, Shandong, China
- 3Department of Blood Purification, The Affiliated Yantai Yuhuangding Hospital of Qingdao University, Yantai, Shandong, China
- 4Department of Traditional Chinese Medicine, Qingdao Eighth People’s Hospital, Qingdao, Shandong, China
- 5Department of Reproductive Medicine, the Affiliated Hospital of Qingdao University, Qingdao, Shandong, China
- 6Department of Pathology, School of Basic Medicine, Qingdao University, Qingdao, Shandong, China
- 7Department of Hematology, Qingdao Eighth People’s Hospital, Qingdao, Shandong, China
Objective: Acute kidney injury (AKI), a syndrome with high morbidity and mortality worldwide, frequently arises from renal ischemia-reperfusion (I/R) injury, particularly in surgical contexts. Despite extensive research, effective therapies for both AKI and its progression to renal interstitial fibrosis remain elusive. This study investigates the potential therapeutic efficacy of glucosamine (GS), an endogenous amino sugar, in alleviating I/R-induced AKI.
Methods: A murine I/R injury model was utilized to evaluate the protective effects of GS. Mice were treated with GS prior to I/R injury, and renal tissues were harvested for biochemical, histological, and molecular analyses. Key markers of oxidative stress, mitochondrial integrity, and endoplasmic reticulum (ER) stress were measured. Additionally, inflammatory responses in proximal convoluted tubular epithelial cells exposed to TPHP, an environmental toxin, were assessed in vitro.
Results: GS administration markedly reduced oxidative stress levels, preserved mitochondrial structure, and mitigated ER stress in renal tissues following I/R injury. Moreover, GS significantly attenuated TPHP-induced inflammatory responses in proximal tubular epithelial cells, suggesting a targeted anti-inflammatory action.
Conclusion: These findings highlight glucosamine’s potential as a therapeutic agent for AKI, offering protection through the modulation of oxidative, mitochondrial, and inflammatory pathways. This study provides foundational evidence for GS as a promising candidate for AKI intervention and opens avenues for further exploration of glucosamine in kidney disease therapeutics.
Introduction
Acute kidney injury (AKI) is characterized by a rapid decline in renal excretory function and is a significant global clinical syndrome associated with high morbidity and mortality rates (Franzin et al., 2020). Renal ischemia-reperfusion (I/R) injury is a major contributor to AKI, frequently occurring during partial nephrectomy, kidney transplantation, and other complex surgical procedures (Saadat-Gilani et al., 2020; Krasinski et al., 2020). However, the precise pathological mechanisms underlying the development of AKI remain poorly understood. Consequently, there is a notable absence of effective preventive and therapeutic strategies in clinic for AKI. While dialysis and kidney transplantation are standard treatments, their long-term costs and potential side effects highlight the urgent need for new therapeutic approaches. Ideally, these approaches should be naturally nontoxic, have minimal adverse reactions, and offer potent curative effects for treating renal I/R injury.
Glucosamine (GS) is an amino sugar characterized by the replacement of the hydroxyl group of glucose with an amino group. (Ma and Gao, 2019). Naturally occurring in the human body, GS plays a pivotal role in the biosynthesis of glycoproteins and glycosaminoglycans (Dalirfardouei et al., 2016). GS is ubiquitously distributed across human tissues, with particularly high concentrations observed in connective tissues and cartilage (Riegger et al., 2021). While clinical trials predominantly explore its efficacy in managing knee osteoarthritis, GS has shown promise in safeguarding cardiomyocytes by enhancing N-acetylglucosamine-modified proteins and mitigating NF-κB activation (Allison et al., 2012; Dong et al., 2022). Furthermore, GS facilitates myocardial repair post-myocardial infarction by modulating macrophage phenotypes towards repair and pro-regression states (Wysoczynski et al., 2022). With recognized anti-inflammatory properties, GS may primarily exert its anticancer effects through the inhibition of pro-inflammatory mediator synthesis (Li et al., 2022; Zahedipour et al., 2017). Therefore, GS offers several unique advantages over other compounds, particularly in the context of treating inflammatory and degenerative conditions. Significantly, recent studies have unveiled the potential of glucosamine hydrochloride in mitigating unilateral ureteral obstruction-induced in vivo and TGF-β1-induced in vitro renal fibrosis, respectively (Park et al., 2013). However, its effects on AKI remain to be elucidated.
In this study, employing both in vivo and in vitro experiments, we elucidate that administration of GS notably mitigates the development of AKI. The protective efficacy of GS is attributed to its ability to inhibit oxidative stress, mitigate mitochondrial damage, and alleviate excessive endoplasmic reticulum (ER) stress. These beneficial effects are mediated through the blockade of the inflammatory response in damaged kidney proximal tubular cells.
Materials and methods
Reagents and antibodies
Antibodies targeting GRP78 and CHOP were procured from Cell Signaling Technology (Danvers, MA, United States), while the antibody against KIM-1 was obtained from R&D Systems (Minneapolis, MN, United States). Proteintech (Chicago, IL, United States) provided the antibodies targeting GAPDH, Bioss (Beijing, China) supplied antibodies against ATF-4, and Elabscience (Wuhan, China) furnished antibodies targeting β-tubulin. Invitrogen (Carlsbad, CA, United States) supplied the secondary antibodies and DAPI. N-acetylcysteine (NAC) was sourced from Yeasen (Shanghai, China), while tert-Butyl hydroperoxide (TBHP) was procured from Macklin (Shanghai, China). Fetal bovine serum (FBS) was acquired from Suero fetal bovine ester, Natocor-Industria Biologica (Cordoba, Argentina), and bovine serum albumin (BSA) from Biosharp (Anhui, China). Triton X-100 was obtained from SolarBio (Beijing, China), and BeyoECL Plus reagent was sourced from Beyotime Biotechnology (Shanghai, China). GS was prepared, purified (purity≥99%) and characterized in our lab (Zhang et al., 2006).
Animals
Male C57BL/6J mice aged 8–10 weeks were procured from Vital River Laboratory Animal Technology Co., Ltd. (Beijing, China). The mice were accommodated in a specific pathogen-free (SPF) animal facility, maintaining a 12-hour light-dark cycle, temperature ranging from 20°C to 24°C, and humidity between 40% and 70%. They were provided with ad libitum access to a standard diet and distilled water.
Recreating mice models of renal I/R injury
Mice were anesthetized with an intraperitoneal injection of 25 mg/kg pentobarbital sodium during the procedure. Abdominal skin and muscle were incised along the midline to expose the renal artery carefully. Mice were randomly divided into 5 groups (n = 12 for each group). In the sham group, the left kidney was exposed without undergoing renal I/R and contralateral nephrectomy. In the I/R group, mice underwent renal artery clamping for 35 min in the left kidney with contralateral nephrectomy. For the I/R-GS150 and I/R-GS300 groups, GS (150 mg/kg or 300 mg/kg) was administered daily by gavage starting from 3 days before the renal injury until sacrifice. In the I/R-NAC group, 100 mg/kg NAC was administered following the procedure. After sacrifice 2 days post-surgery, kidneys tissues were fixed in 10% neutral formalin for further analysis. All animal procedures were conducted in accordance with the guidelines approved by the Institutional Animal Ethics and Use Committee of Qingdao University (Ref: QYFY WZLL 28603).
Cell culture and in vitro experiments
The proximal convoluted tubular epithelial cell line HK-2 cells were cultured in DMEM/F12 medium supplemented with 10% FBS at 37°C in a humidified atmosphere containing 95% air and 5% CO2. Cultured HK-2 cells were randomly assigned to one of four groups: (i) Control group: HK-2 cells were subjected to starvation treatment in DMEM/F12 supplemented with 2% FBS for 24 h, followed by culture in DMEM/F12 supplemented with 10% FBS for an additional 24 h (ii) TBHP group: After starvation, HK-2 cells were cultured in DMEM/F12 supplemented with 10% FBS and 50 μmol/L t-BHP for an additional 24 h (iii) TBHP + NAC group: During starvation treatment, 5 mmol/L NAC was added to the culture medium, followed by exposure to 50 μmol/L t-BHP for 24 h (iv) TBHP + GS group: During starvation treatment, 50 μg/ml GS was added to the culture medium, followed by exposure to 50 μmol/L t-BHP for 24 h. All in vitro experiments with HK-2 cells were conducted in triplicate.
Renal histopathological assessment
Kidney tissues from each experimental group were fixed in 10% neutral buffered formalin solution and embedded in paraffin. Sections of 5 μm thickness were prepared from paraffin-embedded tissues and stained with hematoxylin and eosin (H&E). Images of the stained sections were captured using a light microscope (Nikon ECLPSE 80i, Japan) and evaluated in a blinded manner by a single pathologist. The extent of renal tubular injury was assessed using a previously established 5-point scoring system based on H&E staining, as described in our previous study (Tan et al., 2020). All assessments were conducted by pathologists who were blinded to the experimental group allocations.
Immunohistochemistry
Paraffin-embedded sections were deparaffinized and rehydrated using a graded series of ethanol solutions. Antigen retrieval was performed by incubating the slides in citrate buffer (pH 6.0) using a microwave method, followed by blocking of endogenous peroxidase activity with H2O2 solution for 15 min. The sections were then incubated with primary antibodies against KIM-1 (1:300) overnight at 4°C. Subsequently, the sections were incubated with the corresponding secondary antibodies for 1 h at room temperature. Immunoreactivity was visualized using diaminobenzidine tetrahydrochloride (DAB) staining, and the nuclei were counterstained with hematoxylin. Images of the immunohistochemically stained sections were captured using a light microscope (Nikon ECLPSE 80i, Japan).
Western blot analysis
Cells were harvested, and total proteins were extracted using RIPA buffer. The protein concentrations were determined using a BCA protein assay kit (Beyotime Biotechnology, Shanghai, China). Equal amounts of total protein were resolved by Sodium Dodecyl Sulfate Polyacrylamide Gel Electrophoresis (SDS-PAGE) and subsequently transferred to a polyvinylidene fluoride (PVDF) membrane. Primary antibodies against GRP78 (1:1,000), CHOP (1:1,000), and ATF4 (1:1,000) were employed to assess ER stress levels, with GAPDH antibodies serving as loading SDS controls. Following incubation with corresponding horseradish peroxidase (HRP)-conjugated secondary antibodies, protein bands were imaged using the Syngene GeneGnome XRQ (Syngene) and the intensity of the protein bands was analyzed using SynGene GeneTools software (Syngene) following the manufacturer’s instructions.
Reactive oxygen species (ROS) detection
ROS levels were assessed using a Reactive Oxygen Species Assay Kit (Beyotime Biotechnology, Shanghai, China) following the manufacturer’s instructions. HK-2 cells were treated with DCFH-DA and incubated at 37°C for 20 min, followed by three washes with PBS. DAPI staining was performed simultaneously to visualize the cell nuclei. Fluorescence images were acquired with a fluorescence microscope (Nikon, Japan).
Quantitative measurement of mitochondrial membrane potential
The Mitochondrial Membrane Potential (MMP) of HK-2 cells was assessed using a JC-1 Mitochondrial Membrane Potential Assay Kit (YEASEN, Shanghai, China) following the manufacturer’s instructions. Briefly, HK-2 cells were treated with JC-1 solution and incubated at 37°C for 20 min, followed by washing with 1 × JC-1 staining buffer. Fluorescence images were acquired using a fluorescence microscope (Nikon, Japan), and the red-to-green fluorescence ratio was quantified using ImageJ software.
RNA sequencing
RNA-seq libraries were prepared using the VAHTS® Universal V8 RNA-Seq Library Prep Kit for MGI and VAHTS® RNA Adapters Set 8 for MGI (Vazyme, Nanjing, China). Sequencing was performed using the MGI-SEQ 2000 platform (BGI Technology Co. LTD., Shenzhen, China). Demultiplexed reads were mapped to the human genome using HISAT2 (Pertea et al., 2016), and transcripts were quantified using featureCounts (Liao et al., 2014). Differentiation expression for all groups was determined using DESeq2 (Love et al., 2014). Gene ontology (GO) and KEGG pathway enrichment analysis were performed using DAVID (https://david.ncifcrf.gov/).
Statistical analysis
Data from independent experiments were presented as mean ± standard error of the mean (SEM). Prism software was used for statistical analysis. Statistical significance was determined using one-way analysis of variance (ANOVA). Results with *p < 0.05 were considered statistically significant.
Results
GS mitigates I/R-induced renal damage
To investigate the protective role of GS on acute kidney damage induced by I/R, we utilized a mouse I/R injury model illustrated in Figure 1A. Renal histopathology was assessed using H&E staining 2 days after reperfusion (Figure 1B). No pathological damage was observed in the kidney samples from the sham group. Conversely, the samples from the I/R group exhibited typical AKI characteristics, including lumen obstruction, swelling of renal tubular epithelial cells, intraluminal necrotic cellular debris, and proteinaceous cast formation. Notably, administration of NAC, an ROS inhibitor, markedly attenuated renal pathological damage, serving as a positive control. More importantly, treatment with GS (both 150 mg/kg and 300 mg/kg) resulted in histological improvements comparable to those of the NAC group, reversing the pathological changes induced by I/R injury. Renal tubular injury scores, rated based on H&E staining, revealed that GS (both 150 mg/kg and 300 mg/kg) or NAC treatment strikingly ameliorated the damage of the kidney tissue after I/R injury (Figure 1C), indicating that administration of GS significantly reduced kidney pathological damage 2 days after reperfusion.
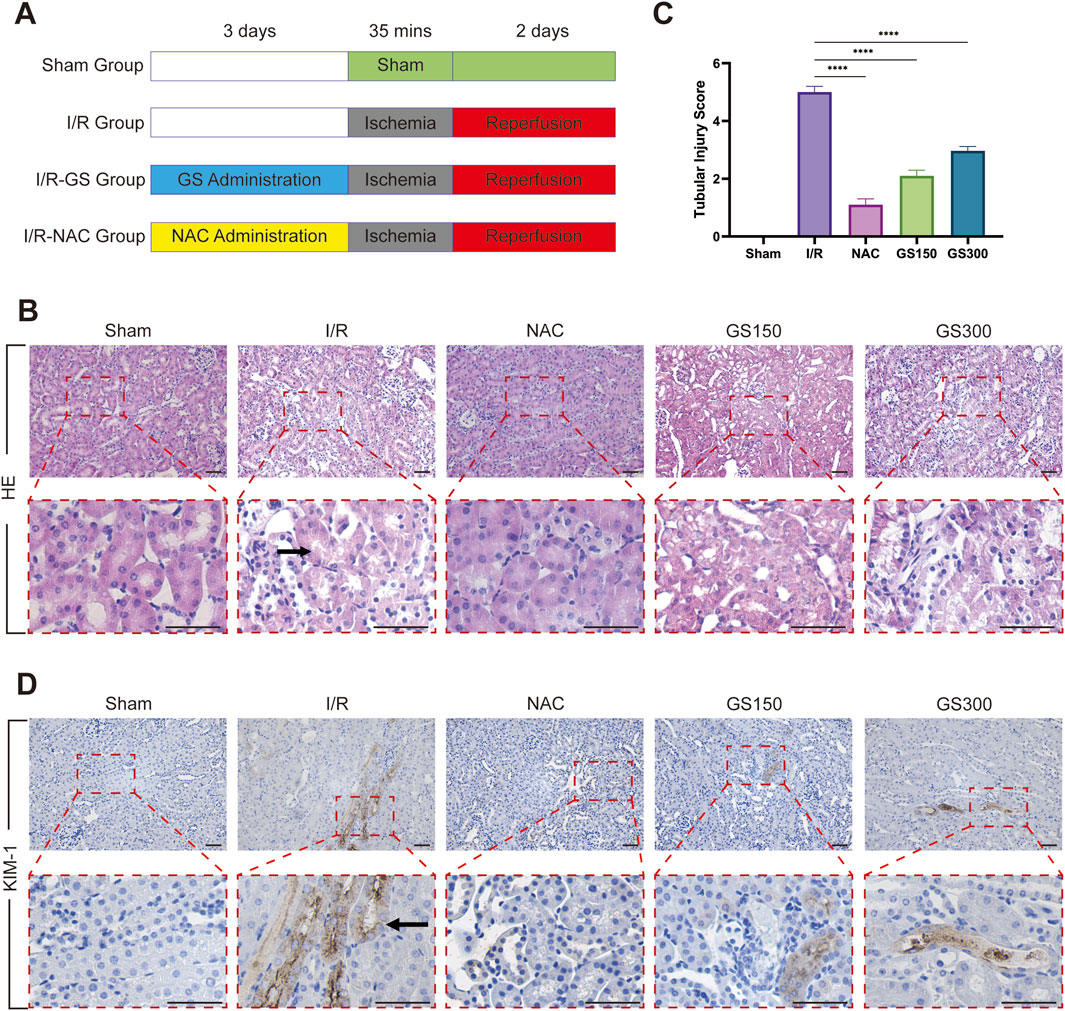
Figure 1. GS administration protects against AKI induced by renal I/R injury. (A) Flow chart for animal procedures of Sham, I/R, I/R-GS and I/R-NAC groups. (B) H&E staining was used to assess renal histological morphology at 2 days after reperfusion. The arrow points to renal tubular swelling, interstitial congestion and glomerular basement membrane thickening. Scale bar represents 50 μm. (C) Tubular injury scores were quantified and analyzed based on H&E staining. Values were mean ± SEM, results were representative of 6 animals in each group, ****P <0.0001. (D) Immunohistochemical staining of kidney sections with KIM-1 antibody on 2 days after IR. The arrow points to KIM1 positive area. Scale bar represents 50 μm.
Furthermore, we performed immunohistochemical staining of KIM-1, a marker of renal proximal tubule cell damage, on these kidney samples. Confocal analysis revealed that the number of KIM-1 positive tubular cells greatly increased in the kidneys samples from the I/R group. However, both NAC and GS treatment markedly reduced the number of KIM-1 positive tubular injury cells, demonstrating that GS treatment effectively protects renal filtration function and maintains renal morphological integrity against AKI induced by I/R (Figure 1D).
GS administration reduces maladaptive ER stress
Several lines of evidence indicates an interrelationship between ER stress and oxidative stress, both playing crucial roles in the pathogenesis of various human diseases (Zeeshan et al., 2016; Kishi et al., 2024; Bhandary et al., 2012). In addition, our previous data suggested that targeting ER stress could be a promising strategy for treating I/R injury (Yin et al., 2023). Thus, we investigated whether GS’s protective effect against I/R renal induced injury involves the regulation of ER stress. Using tBHP-induced HK-2 cells as a model of oxidative stress-induced damage, we observed a clear decrease in the number of HK-2 cells and alterations in cell morphology relative to control group, indicative of cellular damage when treated with tBHP. Notably, the degree of cellular damage was attenuated in both the NAC and GS treatment groups (Figure 2A). Western blot analysis showed a significant increase in the expression of GRP78, CHOP, and ATF-4, key effectors of ER stress, in HK-2 cells following TBHP treatment. However, administration of both NAC and GS resulted in a significant reduction in the production of these ER stress-related proteins, similar as those of control group (Figure 2B). These findings suggest that GS’s protective effect against renal I/R injury involves the inhibition of maladaptive ER stress.
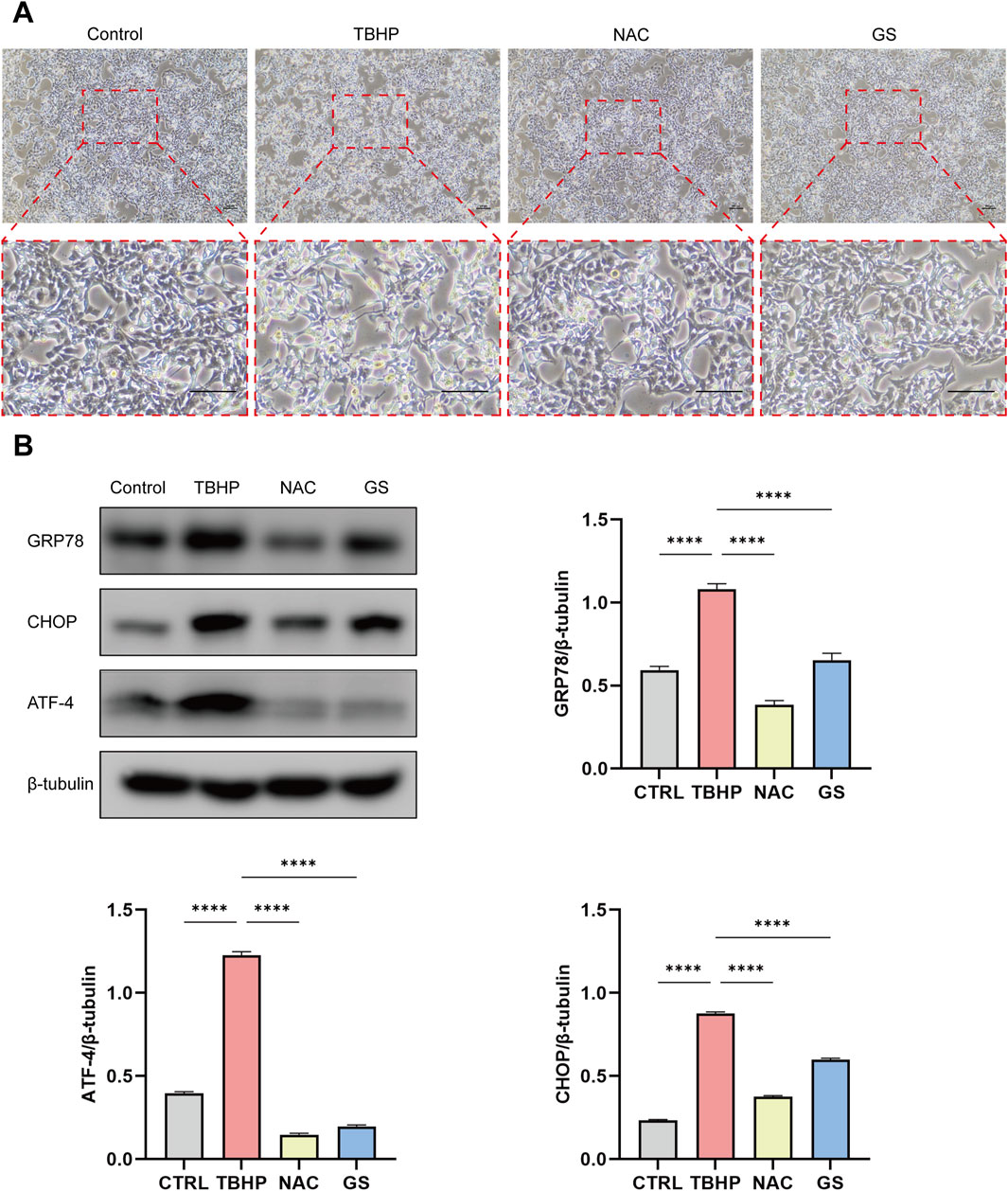
Figure 2. GS administration inhibits ER stress in HK-2 cells treated with TBHP. (A) Morphological photographs of HK-2 cells when treated with TBHP, TBHP + NAC, or TBHP + GS for 2 days. Scale bar represents 100 μm. (B) The expression of ER stress key genes GRP78, CHOP and ATF-4 in HK-2 cells when treated with TBHP, TBHP + NAC, or TBHP + GS were analyzed with Western blotting. β-tubulin was used as loading control. Values were mean ± SEM, results were representative of 8 biological repeats in each group, ****P <0.0001.
GS treatment inhibits mitochondrial oxidative damage
It is well documented that ER stress and oxidative stress are intricately linked and contribute significantly to the pathogenesis of various human diseases, including renal diseases (Ma et al., 2022; Correia de Sousa et al., 2023). To explore whether the protective effect of GS against renal I/R injury involves the mediation of oxidative stress, we investigated its impact on ROS production and mitochondrial damage. Our previous findings have established that excessive ROS generation is central to oxidative stress-induced cellular damage and is associated with mitochondrial dysfunction (Tan et al., 2013). Using an in vitro oxidative stress model, we observed that TBHP treatment resulted in a increase in ROS production by 2 fold in HK-2 cells, whereas both NAC and GS treatments markedly reduced ROS overproduction, similar as those of control group (Figures 3A, B). Additionally, we evaluated Mitochondrial Membrane Potential (MMP) using the JC-1 Assay in HK-2 cells. We observed that TBHP treatment resulted in a notable decrease in the red-to-green fluorescence ratio, indicating the occurrence of mitochondrial depolarization. Remarkably, both NAC and GS treatments effectively preserved MMP in HK-2 cells, as evidenced by a higher red-to-green fluorescence ratio compared to TBHP-treated cells (Figures 3C, D). These findings strongly suggest that the presence of GS mitigates oxidative damage by attenuating ROS overproduction and preserving MMP in HK-2 cells.
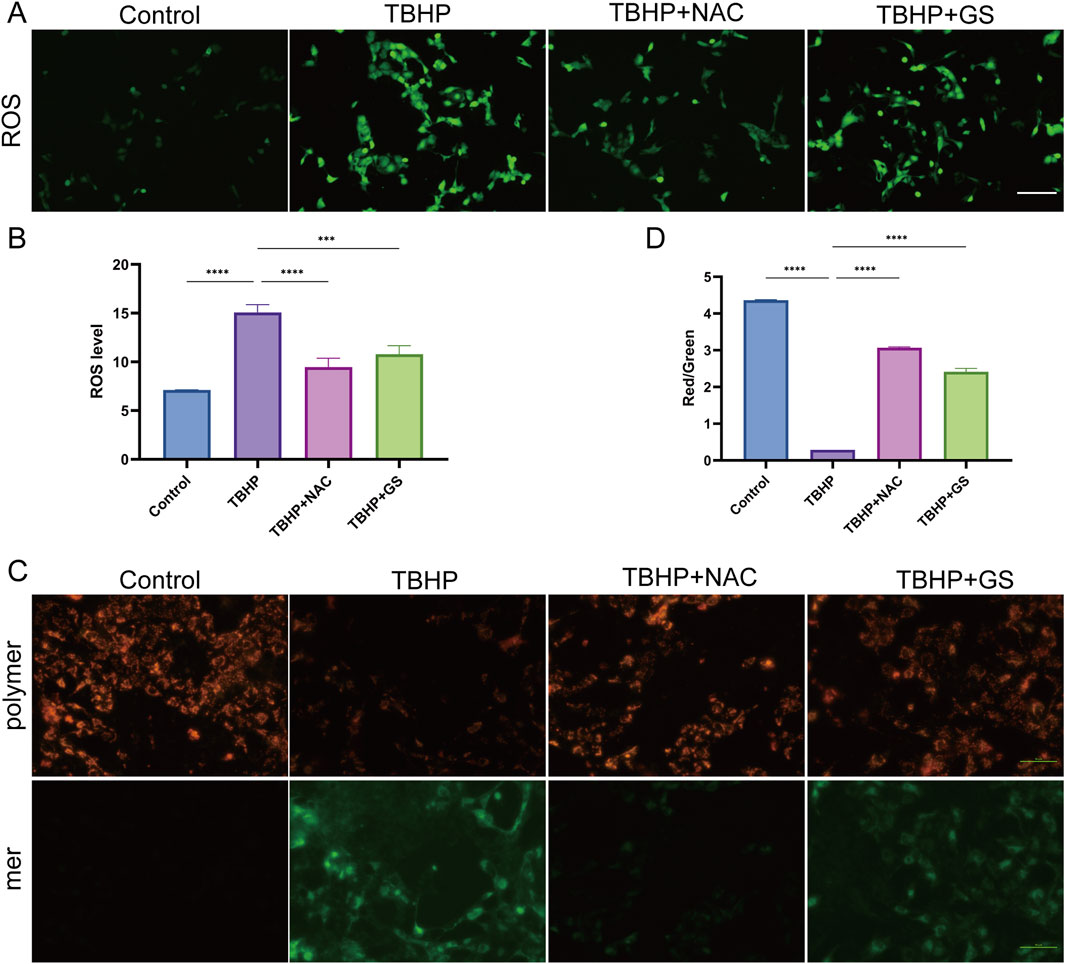
Figure 3. GS administration alleviates oxidative damage of HK-2 cells induced by TBHP. (A) Fluorescence microscopy detection of ROS generation by DCFH-DA in HK-2 cells when treated with TBHP, TBHP + NAC, or TBHP + GS for 2 days. Scale bar represents 50 μm. (B) ROS levels in TCMK-1 cells were quantified as mean ± SEM, results were representative of 8 biological repeats in each group, ***P<0.001, ****P<0.0001. (C) MMP was detected using a JC-1 MMP assay Kit and images was captured using fluorescent microscopy. Scale bar represents 50 μm. (D) The red to green fluorescence ratio was analyzed by image J as mean ± SEM, results were representative of 8 biological repeats in each group, ****P <0.0001.
GS suppresses tubular epithelial cell inflammatory response induced by TPHP
To gain molecular insights into the protective effects of GS, we conducted RNA-seq analysis to elucidate the underlying signaling pathways and key genes implicated in this protective mechanism. Comparative analysis revealed that, in the TBHP-treated group, 1,073 genes were upregulated while 1710 genes were downregulated compared to the control group. However, following GS administration, a significant reversal was observed, with 148 upregulated genes and 172 downregulated genes showing notable changes (Figure 4A).
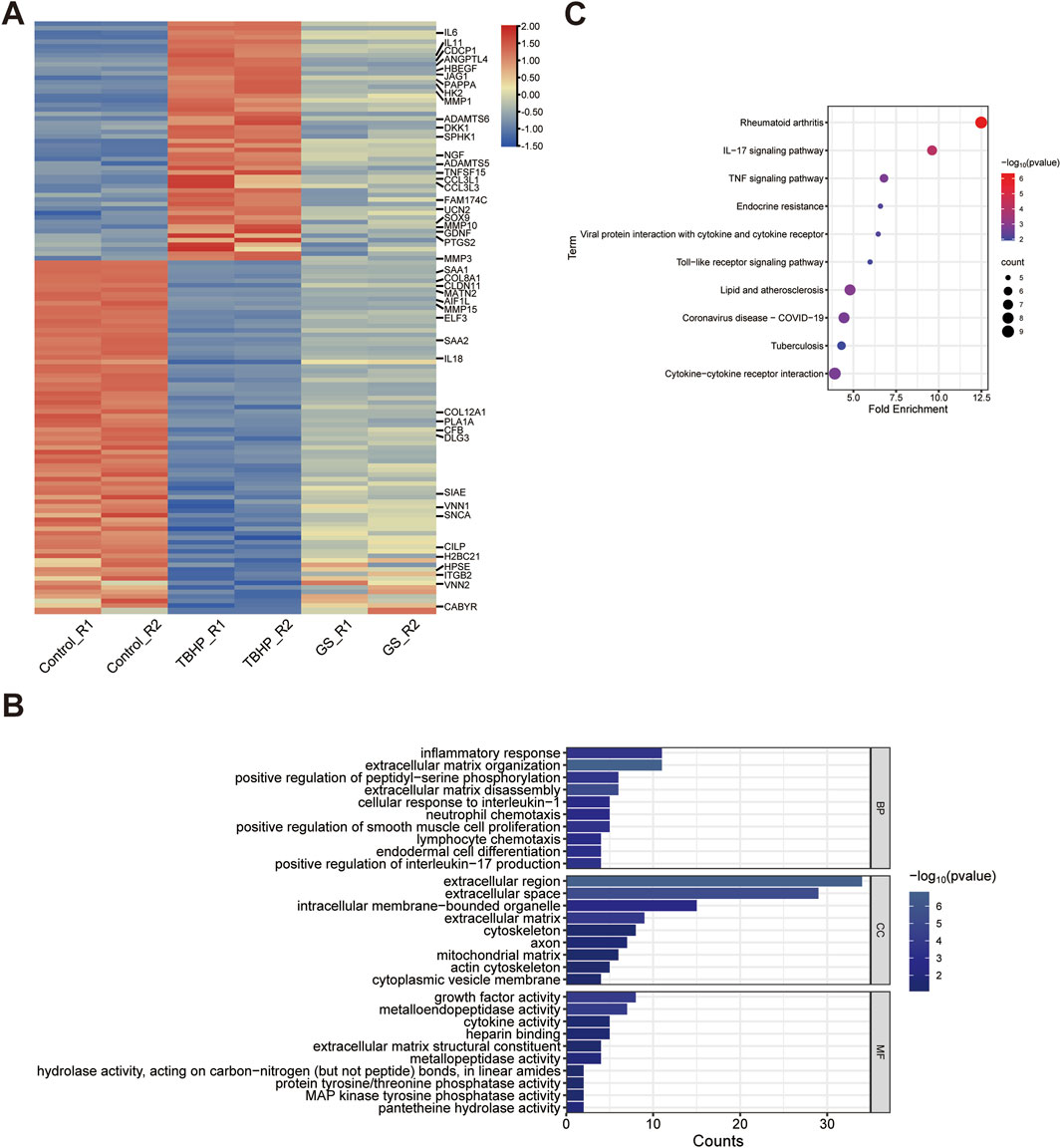
Figure 4. RNA-Seq uncovers the mechanism of the protective effect of GS on I/R-induced AKI. (A) Heatmap shows differential gene expression profiles in RNA-Seq analysis of HK-2 cell when treated with PBS, TBHP, or TBHP + GS for 2 days. The data from biological repeat are shown as fold change greater than 2 and p values less than 0.05 were considered differentially expressed, with upregulation in red, downregulation in blue. (B) GO analysis classifies differential expressed genes (DEGs) into top ranked biological process (BP), cellular component (CC), and molecular function (MF) group. The bar chart was constructed through the bioinformatics platform. According to the comprehensive sorting results of P-value, FDR and Fold Enrichment, the top 10 with the highest degree of enrichment are selected for mapping. (C) KEGG pathway analysis of DEGs. Fold Enrichment refers to the ratio of the proportion of genes detected in a KEGG pathway to the proportion of genes associated with that pathway in the whole genome. KEGG, Kyoto Encyclopedia of Genes and Genomes.
Subsequently, GO function analysis of the protein-coding differentially expressed genes (DEGs) in biological significance mostly associated with inflammatory response, including IL6, VNN1, CCL3L1, ELF3, GPER1, CCL3L3, SPHK1, ITGB2, IL18, HPSE, PTGS2, and extracellular matrix organization, including ADAMTS5, ELF3, MMP15, MMP1, ITGB2, MMP3, COL8A1, SOX9, ADAMTS6, MMP10, MATN2, suggesting that rescue of these genes following IR is the major consequence of GS treatment. Likewise, the cellular component module revealed that these genes were mainly enriched in extracellular region, extracellular space and extracellular matrix, including matrix metalloproteinases (MMP1, MMP3, MMP10, MMP15), cytokine and chemokine families (CSF2, IL11, IL18, IL6, CCL3L1, CCL3L3), collagen (COL12A1 and COL8A1), implying that GS may mediate the extracellular components and structure to regulate the immune response in IR induced AKI. Further molecular factors analysis suggests these immune and extracellular matrix regulation were controlled by growth factor and cytokine activity and MMP activity (Figure 4B). Similarly, KEGG analysis of these DEGs unveiled that GS confers protection against renal injury by inhibiting pathways linked to IL-17 signaling pathway, including MAPK10, CSF2, MMP1 and FOSB, and TNF signaling pathway which were associated with MAPK10, IL6, JAG1, MMP3, PTGS2 (Figure 4C).
Taken together, we concluded that NAG incorporated immune response, extracellular matrix as well as several keys signaling pathways to block IR-induced kidney injury.
Discussion
There are currently no established therapeutics for I/R-induced acute kidney injury (AKI), although several emerging approaches are under investigation, including antioxidant therapy, anti-inflammatory strategies, renoprotective agents, and cell-based treatments. However, these strategies each present distinct limitations that compromise their efficacy and clinical translatability (Li et al., 2023). Antioxidant therapies such as N-acetylcysteine and MitoQ have shown limited efficacy in clinical trials, often failing to replicate the protective effects observed in preclinical models (Tenório et al., 2021; Sulaimon et al., 2022). Anti-inflammatory approaches (e.g., corticosteroids, TNF-α inhibitors) are frequently hindered by systemic side effects, including immunosuppression, hyperglycemia, and an increased susceptibility to infections (Rabb et al., 2016). Renoprotective agents (e.g., fenoldopam, SGLT-2 inhibitors) show efficacy largely dependent on stable hemodynamics, which can be challenging to manage in patients experiencing AKI (Messina et al., 2024). Cell-based therapies, while promising, are restricted by regulatory challenges, high costs, and logistical complexities related to cell processing and preservation, which limit their broader clinical use (Li et al., 2023; Rota et al., 2019). Therefore, the pursuit of preventive measures utilizing health substances with potent therapeutic effects and minimal adverse side effects is imperative. Additionally, the high prevalence of severe renal diseases underscores the urgent need for effective drugs to treat and prevent kidney conditions. Here, we elucidate the renoprotective effects of GS through a combination of in vivo and in vitro experiments. Administration of GS demonstrates a profound ability to ameliorate renal tubular injury and preserve glomerular filtration. This protective effect is attributed to the inhibition of oxidative stress, mitochondrial damage, and ER stress.
The ER is an organelle essential for protein synthesis, folding, maturation, and assembly. It is particularly vulnerable to hypoxia-ischemia-induced stress, which triggers a maladaptive unfolded protein response (UPR) pathway (Oakes and Papa, 2015; Coleman and Haller, 2019). This pathway significantly influences cell fate and is implicated in various human diseases. Our investigation into the association between GS treatment and ER stress revealed that GS effectively alleviates excessive ER stress, thereby contributing to its renoprotective effect (Marciniak et al., 2022). Emerging evidence highlights the interconnectedness of oxidative stress, mitochondrial dysfunction, and ER stress in kidney diseases (Bhargava and Schnellmann, 2017; Cybulsky, 2017; Zhang et al., 2023). Reperfusion injury exacerbates ROS production in mitochondria, intensifying mitochondrial damage and cellular oxidative stress. This oxidative stress amplifies ER stress, leading to a vicious cycle of ROS production and mitochondrial dysfunction, crucial in the pathogenesis of numerous diseases (Saaoud et al., 2023; Verfaillie et al., 2012; Ratliff et al., 2016). MMP is pivotal for mitochondrial oxidative phosphorylation and adenosine triphosphate (ATP) production (Gorospe et al., 2023; Zaib et al., 2022). Accordingly, ROS overproduction and MMP decline serve as early indicators of I/R injury (Sun et al., 2005; Xiao et al., 2022; Chen et al., 2023; Su et al., 2023). Our findings demonstrate that GS treatment mitigates ROS overproduction, thereby safeguarding mitochondria against oxidative damage.
We identified GS exhibit mitochondrial protection mechanisms in the AKI model. One possible mechanism involves GS’s ability to improve mitochondrial respiration efficiency and increase ATP production, thereby supporting cellular energy demands (Shen et al., 2024). Additionally, it may attenuate the generation of ROS, mitigating mitochondrial damage linked to oxidative stress (Ma et al., 2020). Furthermore, GS can activate the hexosamine biosynthesis pathway, which has been implicated in promoting mitochondrial autophagy (mitophagy)—the selective removal of damaged mitochondria. By facilitating mitophagy, GS helps preserve mitochondrial integrity and maintain energy homeostasis (Paneque et al., 2023; Caramés et al., 2013).
Our findings indicate that GS treatment effectively suppresses inflammation-related pathways, thereby attenuating inflammatory responses and providing protection against AKI. Upon injury, not only tissue-resident immune cells, such as dendritic cells and macrophages, but also native renal cells, express receptors or cytokines to sense damage (Duthie et al., 2016; Jiang et al., 2022). Subsequently, intrarenal immune cells respond to these stimuli, and initiated to actively secrete cytokines such as interleukins (ILs) and chemokines. These cytokines then migrate to local blood vessels and recruit leukocytes, including subsequent macrophages (Duthie et al., 2016). Indeed, upon GS treatment, several ILs, such as IL11, IL6, and IL18, as well as CCL3L1 and CCL3L3, were significantly inhibited, accompanied by the downregulation of TNFRSF10A. These findings are consistent with GS’s role in immune regulation and apoptosis protection in renal tissue, highlighting the ways in which GS mediates AKI protection through a novel and safe mechanism.
While our data are promising, it is important to consider the variability in IR injury models and potential differences in GS’s efficacy across different species or patient populations (Shiva et al., 2020). Further research is needed to validate these findings in clinical settings. Glucosamine, widely available as a dietary supplement, is considered safe based on pharmacokinetic analysis. Upon oral administration, glucosamine is rapidly absorbed, though its bioavailability remains relatively low at 20%–30% due to first-pass liver metabolism. Peak plasma concentrations are reached within 2–4 h, and it primarily accumulates in cartilage, supporting joint health. Glucosamine undergoes liver metabolism through pathways akin to glucose, contributing to glycosaminoglycan synthesis, which is crucial for cartilage repair. Excretion occurs predominantly through the kidneys, with a half-life of about 15 h. Clinical studies have shown that glucosamine is generally well-tolerated both short- and long-term at the recommended daily dose of 1,500 mg. Overall, the pharmacokinetic analysis supports the safety of glucosamine. While it is also crucial to consider the potential adverse effects of glucosamine administration (Anderson et al., 2005). Although oral glucosamine, commonly used for joint health and osteoarthritis, is generally well-tolerated, it can have adverse effects such as gastrointestinal issues, allergic reactions, blood sugar complications, and interactions with other medications, as well as isolated incidents of renal injury (Dahmer and Schiller, 2008). The integration of glucosamine as a therapeutic agent for AKI not only supports the development of novel treatment strategies but also has the potential to improve patient outcomes by reducing the incidence and severity of renal injury (Hu et al., 2017). Therefore, a comparative analysis of this minimally invasive approach with more invasive strategies reported in the literature is recommended.
Conclusion
In conclusion, our study underscores the antioxidant and anti-inflammatory properties of GS and highlights its therapeutic potential for I/R-induced AKI. These findings offer insights into the development of novel preventive and therapeutic strategies for AKI management.
Data availability statement
The original contributions presented in the study are included in the article/Supplementary Material. Further inquiries can be directed to the corresponding authors.
Ethics statement
The animal study was approved by Our study has been approved by the Ethics Committee of the Affiliated Hospital of Qingdao University (Ethical approval no. QYFY WZLL27937). The study was conducted in accordance with the local legislation and institutional requirements.
Author contributions
GZ: Software, Writing–original draft, Methodology, Supervision. SJ: Conceptualization, Investigation, Writing–original draft. XF: Writing–original draft, Data curation, Software. JQ: Writing–review and editing, Visualization, Software. JL: Methodology, Supervision, Writing–original draft, Formal Analysis. SY: Formal Analysis, Supervision, Writing–original draft, Data curation. YC: Formal Analysis, Writing–original draft, Validation. YD: Writing–review and editing, Methodology, Validation, Visualization. XD: Validation, Writing–original draft, Resources, Software. ZW: Funding acquisition, Project administration, Writing–review and editing. XT: Funding acquisition, Resources, Visualization, Writing–review and editing. SY: Project administration, Resources, Visualization, Writing–review and editing.
Funding
The author(s) declare that financial support was received for the research, authorship, and/or publication of this article. This work was funded by grants from the Natural Science Foundation of Shandong Province (ZR2020MC083 to ZW and ZR2020MH077 to XT), National Natural Science Foundation of China (32070859 to ZW), Taishan Scholars Program of Shandong Province (TS20190931 to ZW), and China Postdoctoral Science Foundation (316116 to XT).
Acknowledgments
The authors thank the participants and staff of this study for their time and commitment.
Conflict of interest
The authors declare that the research was conducted in the absence of any commercial or financial relationships that could be construed as a potential conflict of interest.
Publisher’s note
All claims expressed in this article are solely those of the authors and do not necessarily represent those of their affiliated organizations, or those of the publisher, the editors and the reviewers. Any product that may be evaluated in this article, or claim that may be made by its manufacturer, is not guaranteed or endorsed by the publisher.
References
Allison, D. F., Wamsley, J. J., Kumar, M., Li, D., Gray, L. G., Hart, G. W., et al. (2012). Modification of RelA by O-linked N-acetylglucosamine links glucose metabolism to NF-κB acetylation and transcription. Proc. Natl. Acad. Sci. U. S. A. 109 (42), 16888–16893. doi:10.1073/pnas.1208468109
Anderson, J. W., Nicolosi, R. J., and Borzelleca, J. F. (2005). Glucosamine effects in humans: a review of effects on glucose metabolism, side effects, safety considerations and efficacy. Food Chem. Toxicol. 43 (2), 187–201. doi:10.1016/j.fct.2004.11.006
Bhandary, B., Marahatta, A., Kim, H. R., and Chae, H. J. (2012). An involvement of oxidative stress in endoplasmic reticulum stress and its associated diseases. Int. J. Mol. Sci. 14 (1), 434–456. doi:10.3390/ijms14010434
Bhargava, P., and Schnellmann, R. G. (2017). Mitochondrial energetics in the kidney. Nat. Rev. Nephrol. 13 (10), 629–646. doi:10.1038/nrneph.2017.107
Caramés, B., Kiosses, W. B., Akasaki, Y., Brinson, D. C., Eap, W., Koziol, J., et al. (2013). Glucosamine activates autophagy in vitro and in vivo. Arthritis Rheum. 65 (7), 1843–1852. doi:10.1002/art.37977
Chen, R. Y., Li, D. W., Xie, H., Liu, X. W., Zhuang, S. Y., Wu, H. Y., et al. (2023). Gene signature and prediction model of the mitophagy-associated immune microenvironment in renal ischemia-reperfusion injury. Front. Immunol. 14, 1117297. doi:10.3389/fimmu.2023.1117297
Coleman, O. I., and Haller, D. (2019). ER stress and the UPR in shaping intestinal tissue homeostasis and immunity. Front. Immunol. 10, 2825. doi:10.3389/fimmu.2019.02825
Correia de Sousa, M., Delangre, E., Türkal, M., Foti, M., and Gjorgjieva, M. (2023). Endoplasmic reticulum stress in renal cell carcinoma. Int. J. Mol. Sci. 24 (5), 4914. doi:10.3390/ijms24054914
Cybulsky, A. V. (2017). Endoplasmic reticulum stress, the unfolded protein response and autophagy in kidney diseases. Nat. Rev. Nephrol. 13 (11), 681–696. doi:10.1038/nrneph.2017.129
Dalirfardouei, R., Karimi, G., and Jamialahmadi, K. (2016). Molecular mechanisms and biomedical applications of glucosamine as a potential multifunctional therapeutic agent. Life Sci. 152, 21–29. doi:10.1016/j.lfs.2016.03.028
Dong, H., Liu, Z., and Wen, H. (2022). Protein O-GlcNAcylation regulates innate immune cell function. Front. Immunol. 13, 805018. doi:10.3389/fimmu.2022.805018
Duthie, F., O’Sullivan, E. D., and Hughes, J. (2016). ISN forefronts symposium 2015: the diverse function of macrophages in renal disease. Kidney Int. Rep. 1 (3), 204–209. doi:10.1016/j.ekir.2016.08.004
Franzin, R., Stasi, A., Fiorentino, M., Stallone, G., Cantaluppi, V., Gesualdo, L., et al. (2020). Inflammaging and complement system: a link between acute kidney injury and chronic graft damage. Front. Immunol. 11, 734. doi:10.3389/fimmu.2020.00734
Gorospe, C. M., Carvalho, G., Herrera Curbelo, A., Marchhart, L., Mendes, I. C., Niedźwiecka, K., et al. (2023). Mitochondrial membrane potential acts as a retrograde signal to regulate cell cycle progression. Life Sci. Alliance 6 (12), e202302091. doi:10.26508/lsa.202302091
Hu, J., Chen, R., Jia, P., Fang, Y., Liu, T., Song, N., et al. (2017). Augmented O-GlcNAc signaling via glucosamine attenuates oxidative stress and apoptosis following contrast-induced acute kidney injury in rats. Free Radic. Biol. Med. 103, 121–132. doi:10.1016/j.freeradbiomed.2016.12.032
Jiang, W. J., Xu, C. t., Du, C. l., Dong, J. h., Xu, S. b., Hu, B. f., et al. (2022). Tubular epithelial cell-to-macrophage communication forms a negative feedback loop via extracellular vesicle transfer to promote renal inflammation and apoptosis in diabetic nephropathy. Theranostics 12 (1), 324–339. doi:10.7150/thno.63735
Kishi, S., Nagasu, H., Kidokoro, K., and Kashihara, N. (2024). Oxidative stress and the role of redox signalling in chronic kidney disease. Nat. Rev. Nephrol. 20 (2), 101–119. doi:10.1038/s41581-023-00775-0
Krasinski, Z., Krasińska, B., Olszewska, M., and Pawlaczyk, K. (2020). Acute renal failure/acute kidney injury (AKI) associated with endovascular procedures. Diagn. (Basel) 10 (5), 274. doi:10.3390/diagnostics10050274
Li, G., Zhang, X., Liu, Y., Zhang, J., Li, L., Huang, X., et al. (2022). Relationship between glucosamine use and the risk of lung cancer: data from a nationwide prospective cohort study. Eur. Respir. J. 59 (3), 2101399. doi:10.1183/13993003.01399-2021
Li, N., Han, L., Wang, X., Qiao, O., Zhang, L., and Gong, Y. (2023). Biotherapy of experimental acute kidney injury: emerging novel therapeutic strategies. Transl. Res. 261, 69–85. doi:10.1016/j.trsl.2023.06.002
Liao, Y., Smyth, G. K., and Shi, W. (2014). featureCounts: an efficient general purpose program for assigning sequence reads to genomic features. Bioinformatics 30 (7), 923–930. doi:10.1093/bioinformatics/btt656
Love, M. I., Huber, W., and Anders, S. (2014). Moderated estimation of fold change and dispersion for RNA-seq data with DESeq2. Genome Biol. 15 (12), 550. doi:10.1186/s13059-014-0550-8
Ma, H., Li, X., Zhou, T., Sun, D., Liang, Z., Li, Y., et al. (2020). Glucosamine use, inflammation, and genetic susceptibility, and incidence of type 2 diabetes: a prospective study in UK biobank. Diabetes Care 43 (4), 719–725. doi:10.2337/dc19-1836
Ma, Q., and Gao, X. (2019). Categories and biomanufacturing methods of glucosamine. Appl. Microbiol. Biotechnol. 103 (19), 7883–7889. doi:10.1007/s00253-019-10084-x
Ma, Y., Su, Q., Yue, C., Zou, H., Zhu, J., Zhao, H., et al. (2022). The effect of oxidative stress-induced autophagy by cadmium exposure in kidney, liver, and bone damage, and neurotoxicity. Int. J. Mol. Sci. 23 (21), 13491. doi:10.3390/ijms232113491
Marciniak, S. J., Chambers, J. E., and Ron, D. (2022). Pharmacological targeting of endoplasmic reticulum stress in disease. Nat. Rev. Drug Discov. 21 (2), 115–140. doi:10.1038/s41573-021-00320-3
Messina, A., Calatroni, M., Castellani, G., De Rosa, S., Ostermann, M., and Cecconi, M. (2024). Understanding fluid dynamics and renal perfusion in acute kidney injury management. J. Clin. Monit. Comput. doi:10.1007/s10877-024-01209-3
Oakes, S. A., and Papa, F. R. (2015). The role of endoplasmic reticulum stress in human pathology. Annu. Rev. Pathol. 10, 173–194. doi:10.1146/annurev-pathol-012513-104649
Paneque, A., Fortus, H., Zheng, J., Werlen, G., and Jacinto, E. (2023). The hexosamine biosynthesis pathway: regulation and function. Genes. (Basel) 14 (4), 933. doi:10.3390/genes14040933
Park, J., Lee, S. Y., Ooshima, A., Yang, K. M., Kang, J. M., Kim, Y. W., et al. (2013). Glucosamine hydrochloride exerts a protective effect against unilateral ureteral obstruction-induced renal fibrosis by attenuating TGF-β signaling. J. Mol. Med. Berl. 91 (11), 1273–1284. doi:10.1007/s00109-013-1086-1
Pertea, M., Kim, D., Pertea, G. M., Leek, J. T., and Salzberg, S. L. (2016). Transcript-level expression analysis of RNA-seq experiments with HISAT, StringTie and Ballgown. Nat. Protoc. 11 (9), 1650–1667. doi:10.1038/nprot.2016.095
Rabb, H., Griffin, M. D., McKay, D. B., Swaminathan, S., Pickkers, P., Rosner, M. H., et al. (2016). Inflammation in AKI: current understanding, key questions, and knowledge gaps. J. Am. Soc. Nephrol. 27 (2), 371–379. doi:10.1681/asn.2015030261
Ratliff, B. B., Abdulmahdi, W., Pawar, R., and Wolin, M. S. (2016). Oxidant mechanisms in renal injury and disease. Antioxid. Redox Signal 25 (3), 119–146. doi:10.1089/ars.2016.6665
Riegger, J., Baumert, J., Zaucke, F., and Brenner, R. E. (2021). The hexosamine biosynthetic pathway as a therapeutic target after cartilage trauma: modification of chondrocyte survival and metabolism by glucosamine derivatives and PUGNAc in an ex vivo model. Int. J. Mol. Sci. 22 (14), 7247. doi:10.3390/ijms22147247
Rota, C., Morigi, M., and Imberti, B. (2019). Stem cell therapies in kidney diseases: progress and challenges. Int. J. Mol. Sci. 20 (11), 2790. doi:10.3390/ijms20112790
Saadat-Gilani, K., Zarbock, A., and Meersch, M. (2020). Perioperative renoprotection: clinical implications. Anesth. Analg. 131 (6), 1667–1678. doi:10.1213/ane.0000000000004995
Saaoud, F., Liu, L., Xu, K., Cueto, R., Shao, Y., Lu, Y., et al. (2023). Aorta- and liver-generated TMAO enhances trained immunity for increased inflammation via ER stress/mitochondrial ROS/glycolysis pathways. JCI Insight 8 (1), e158183. doi:10.1172/jci.insight.158183
Shen, Y., Dinh, H. V., Cruz, E. R., Chen, Z., Bartman, C. R., Xiao, T., et al. (2024). Mitochondrial ATP generation is more proteome efficient than glycolysis. Nat. Chem. Biol. 20 (9), 1123–1132. doi:10.1038/s41589-024-01571-y
Shiva, N., Sharma, N., Kulkarni, Y. A., Mulay, S. R., and Gaikwad, A. B. (2020). Renal ischemia/reperfusion injury: an insight on in vitro and in vivo models. Life Sci. 256, 117860. doi:10.1016/j.lfs.2020.117860
Su, L., Zhang, J., Gomez, H., Kellum, J. A., and Peng, Z. (2023). Mitochondria ROS and mitophagy in acute kidney injury. Autophagy 19 (2), 401–414. doi:10.1080/15548627.2022.2084862
Sulaimon, L. A., Afolabi, L. O., Adisa, R. A., Ayankojo, A. G., Afolabi, M. O., Adewolu, A. M., et al. (2022). Pharmacological significance of MitoQ in ameliorating mitochondria-related diseases. Adv. Redox Res. 5, 100037. doi:10.1016/j.arres.2022.100037
Sun, C. K., Zhang, X., Sheard, P., Mabuchi, A., and Wheatley, A. (2005). Change in mitochondrial membrane potential is the key mechanism in early warm hepatic ischemia-reperfusion injury. Microvasc. Res. 70 (1-2), 102–110. doi:10.1016/j.mvr.2005.04.003
Tan, X., Yu, L., Yang, R., Tao, Q., Xiang, L., Xiao, J., et al. (2020). Fibroblast growth factor 10 attenuates renal damage by regulating endoplasmic reticulum stress after ischemia-reperfusion injury. Front. Pharmacol. 11, 39. doi:10.3389/fphar.2020.00039
Tan, X., Zhang, L., Jiang, Y., Yang, Y., Zhang, W., Li, Y., et al. (2013). Postconditioning ameliorates mitochondrial DNA damage and deletion after renal ischemic injury. Nephrol. Dial. Transpl. 28 (11), 2754–2765. doi:10.1093/ndt/gft278
Tenório, M., Graciliano, N. G., Moura, F. A., Oliveira, A. C. M. d., and Goulart, M. O. F. (2021). N-acetylcysteine (NAC): impacts on human health. Antioxidants (Basel) 10 (6), 967. doi:10.3390/antiox10060967
Verfaillie, T., Rubio, N., Garg, A. D., Bultynck, G., Rizzuto, R., Decuypere, J. P., et al. (2012). PERK is required at the ER-mitochondrial contact sites to convey apoptosis after ROS-based ER stress. Cell. Death Differ. 19 (11), 1880–1891. doi:10.1038/cdd.2012.74
Wysoczynski, M., Stephan, J., and Uchida, S. (2022). Glucosamine regulates macrophage function in heart failure. Clin. Transl. Med. 12 (4), e819. doi:10.1002/ctm2.819
Xiao, J. J., Liu, Q., Li, Y., Peng, F. F., Wang, S., Zhang, Z., et al. (2022). Regulator of calcineurin 1 deletion attenuates mitochondrial dysfunction and apoptosis in acute kidney injury through JNK/Mff signaling pathway. Cell. Death Dis. 13 (9), 774. doi:10.1038/s41419-022-05220-x
Yin, S., Liu, J., Zhao, X., Dong, H., Cao, Y., Zhang, S., et al. (2023). Chitosan oligosaccharide attenuates acute kidney injury and renal interstitial fibrosis induced by ischemia-reperfusion. Ren. Fail 45 (1), 2238831. doi:10.1080/0886022x.2023.2238831
Zahedipour, F., Dalirfardouei, R., Karimi, G., and Jamialahmadi, K. (2017). Molecular mechanisms of anticancer effects of Glucosamine. Biomed. Pharmacother. 95, 1051–1058. doi:10.1016/j.biopha.2017.08.122
Zaib, S., Khan, I., Hayyat, A., Ali, N., Gul, A., and Naveed, M. (2022). Role of mitochondrial membrane potential and lactate dehydrogenase A in apoptosis. Anticancer Agents Med. Chem. 22 (11), 2048–2062. doi:10.2174/1871520621666211126090906
Zeeshan, H. M., Lee, G., Kim, H. R., and Chae, H. J. (2016). Endoplasmic reticulum stress and associated ROS. Int. J. Mol. Sci. 17 (3), 327. doi:10.3390/ijms17030327
Zhang, L., Liu, W. s., Han, B. q., Peng, Y. f., and Wang, D. f. (2006). Antitumor activities of D-glucosamine and its derivatives. J. Zhejiang Univ. Sci. B 7 (8), 608–614. doi:10.1631/jzus.2006.b0608
Keywords: acute kidney injury, ischemia-reperfusion, glucosamine, tubular epithelial cells, inflammatory response
Citation: Zhang G, Jin S, Fan X, Qi J, Liu J, Yin S, Cao Y, Du Y, Dong X, Wang Z, Tan X and Yan S (2024) Glucosamine mitigates ischemia-reperfusion-induced acute kidney injury through anti-inflammatory mechanisms. Front. Mater. 11:1438610. doi: 10.3389/fmats.2024.1438610
Received: 26 May 2024; Accepted: 04 November 2024;
Published: 26 November 2024.
Edited by:
Rajesh R. Naik, Minded XAI, United StatesReviewed by:
Shanshan Shi, University of South Carolina, United StatesXiaodong Zou, The Chinese University of Hong Kong, Shenzhen, China
Xianhao Zhou, University of Rhode Island, United States
Copyright © 2024 Zhang, Jin, Fan, Qi, Liu, Yin, Cao, Du, Dong, Wang, Tan and Yan. This is an open-access article distributed under the terms of the Creative Commons Attribution License (CC BY). The use, distribution or reproduction in other forums is permitted, provided the original author(s) and the copyright owner(s) are credited and that the original publication in this journal is cited, in accordance with accepted academic practice. No use, distribution or reproduction is permitted which does not comply with these terms.
*Correspondence: Zheng Wang, emhlbmcud2FuZ0BxZHUuZWR1LmNu; Xiaohua Tan, dGFueGhAcWR1LmVkdS5jbg==; Shu Yan, eWFuc2h1QHFkdWhvc3BpdGFsLmNu
†ORCID: Zheng Wang, orcid.org/0000-0003-4471-5983
‡These authors have contributed equally to this work