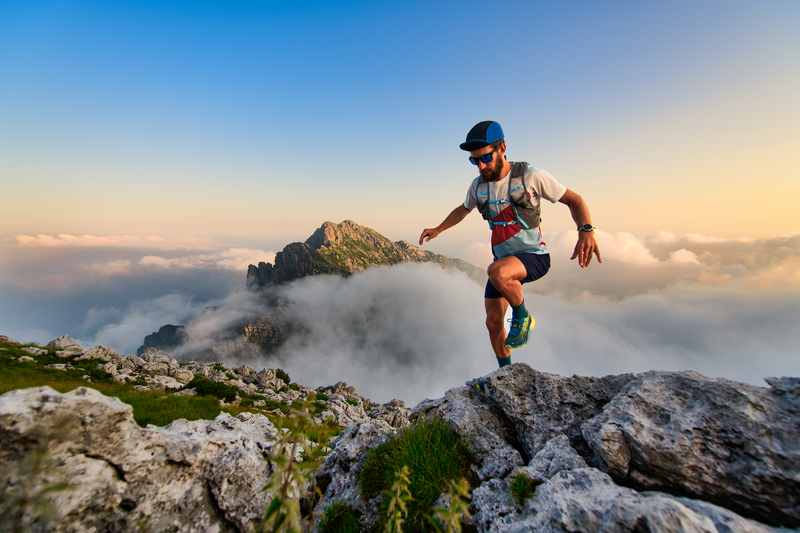
94% of researchers rate our articles as excellent or good
Learn more about the work of our research integrity team to safeguard the quality of each article we publish.
Find out more
PERSPECTIVE article
Front. Mater. , 23 February 2024
Sec. Biomaterials and Bio-Inspired Materials
Volume 11 - 2024 | https://doi.org/10.3389/fmats.2024.1362141
This article is part of the Research Topic Women In Science: Materials 2023 View all 10 articles
Sensorineural hearing loss (SNHL) occurs when the sound transduction mechanism in the inner ear is compromised, because of impairments affecting the sensory hair cells—the actual biological transducers (90% of cases)—or the neurons. SNHL results in a broad spectrum of developmental, cognitive and psycho-social damages. To date, only cochlear implants (CIs) can offer a therapeutic solution to patients. They are multi-component electronic devices, surgically implanted, which capture, elaborate and convert the sound into electric stimuli delivered to the cochlea. Due to inherent limitations of the current electronic-based CIs, a new class of devices has been envisioned, which is based on piezoelectric materials. However, using piezoelectric membranes, the obtained sensitivity was not enough. The new frontiers for piezoelectric material-based CI aim at synergizing micro/nanofabrication aided by multiscale materials modeling with an in vivo tissue engineering approach to provide an implantable biomaterial-based system for SNHL, acting as a next-generation CI. Specifically, the envisioned device will move forward the primitive concept of bulk-structured piezoelectric CIs by designing a nanostructured material (e.g., based on nanofibers) to be precisely delivered and be intimately and efficiently integrated with the cochlear microenvironment. Piezoelectric material-based CIs are indeed hypothesized to have a much higher resolution of electrical stimulation with more than hundreds of channels, compared to maximum 22 stimulating elements present in electronic-based CIs. Moreover, the stimulation site will be closest to peripheral nerve fiber endings for maximal resolution. This would be the first sensory implant with a feedback mechanism on a micrometer scale.
Ear consists of three sequential compartments: outer for collection, middle for conduction and inner for mechanic-into-electric signal conversion of sound waves. In the latter compartment, the proper sensory function takes place by means of the hair cells that work as in-liquid mechano-electrical transducers; thus, the auditory neurons transmit the electric signal to the brain, where the hearing sense is finally elaborated (Figure 1A) (Fettiplace and Hackney, 2006). Over 5% of the world population—about 466 million people—suffer from disabling hearing loss (WHO website, 2023). When the transduction mechanism, namely, sensory cells and/or neurons, is damaged, sensorineural hearing loss (SNHL) occurs (Liu et al., 2023), which impacts the developmental, cognitive and psycho-social abilities. In about 90% of cases, SNHL affects the inner ear because of damaged sensory epithelium and most rarely involving the underlying auditory neurons. The ear sensory cells, namely, hair cells, act as biological transducers and reside in the organ of Corti, the core structure of the cochlea. Here, they convert the mechanical vibration of the basilar membrane—a thin membrane dividing the cochlea into fluid filled compartments—into stimulation of the underlying spiral ganglion neurons via neurotransmitters (Figures 1B–D) (Fettiplace and Hackney, 2006). Unfortunately, hair cells do not self-renovate over time and are available in predefined and small numbers with respect to other senses (Grandori and Martini, 1995). Although ageing or external factors, such as traumas, exposure to noise and ototoxic effects of certain drugs, can affect the viability and function of the hair cells, in most cases (>60%) SNHL is associated to inherited genetic conditions (Ciorba et al., 2009). SNHL results in a broad spectrum of developmental, cognitive and psycho-social damages, which include linguistic deficit in deaf-born children, who are thus early implanted (Forli et al., 2011). Restoring SNHL is much more complicated than conductive hearing loss, the latter (as a mechanical impairment) performed by replacement prostheses and reconstructive surgery, and only complex electronic implants, namely, cochlear implants (CIs), can offer a therapeutic solution to patients to bypass sensory cells and directly stimulate the auditory nerve (Carlyonet al., 2022). The CI is a multi-component electronic device implanted through a surgical procedure of the temporal bone, which replaces the whole ear function. Despite of efforts towards new biological and pharmaceutical strategies, nowadays, the only successful treatment for SNHL still relies on the CI implant. In fact, as today CIs represent the only option for deaf people affected by profound or severe SNHL, while mild-to-moderate forms are only treated with hearing aids due to the costs (e.g., ⁓ a hundred thousand euro for a public national health system) and risks associated to CIs (Berrettini et al., 2011; Tanna et al., 2024). Although conventional CIs can bring patients back to an acceptable hearing, they suffer from some important disadvantages impacting the quality of life and hearing after implantation, such as water unsuitability, battery recharge dependency and hearing sound differently, and for such reasons a new class of CIs is highly desirable.
FIGURE 1. The human cochlea: (A) Schematic of the ear and hearing (adapted from Wikimedia under Creative Commons Attribution-Share Alike 4.0); (B) Schematic of a cross-section of the cochlea indicating the several anatomic structures (Adapted from OpenStax under the CC BY license); (C) Scanning electron microscopy micrograph of a cross-sectioned cochlea, displaying the thin basilar membrane and other decellularized cochlear structures (original image of the authors); (D) Schematic of a portion of the organ of Corti, showing a couple of hair cells with the tectorial membrane (Adapted from OpenStax under the CC BY license).
Piezoelectric material-based CIs would theoretically represent an innovative and smart solution in this field: they work in a biomimetic fashion, are self-powered and fully implantable (i.e., better water-compliant), less expensive (as material-based instead of electronic-based) and a-magnetic, leading to improved magnetic resonance imaging (MRI) compatibility than current bionic CIs (Inaoka et al., 2011). By exploiting the mechanical tonotopy still present in deaf cochleae, piezoelectric materials in contact with the basilar membrane could potentially enable a fine-tuning process of the sound vibrations, thus providing a better quality of hearing versus discretized electrodes in electronic CIs. However, several issues have still to be solved before reaching the clinical practice, which demand a visionary and integrated research. On the materials side, piezoelectric CIs in the form of solid slabs have shown insufficient sensitivity (Inaoka et al., 2011). The research efforts are still variegated, instead, synergistic multi-disciplinary integrated approaches must be put in place for hearing restoration (Latif et al., 2021).
The hearing frequency range in humans is in 20–20.000 Hz (in ⁓ 2½ rounds of the spiral), wherein speech frequencies are in 500–5.000 Hz (Figure 1A). The capability of decoding the sound waves into frequencies, each one resonating locally at a specific length of the membrane is in fact a characteristic of the cochlea as a physical system discussed by the Nobel prize laureate G. von Békésy in his “travelling wave theory”, which provides the basics of cochlear organ function (von Békésy, 1970). As the sound waves are transmitted across these ramps, they induce vibration of the basilar membrane. At the core of inner ear physiology, hair cells convert mechanical into electrical signals to excite sensory neurons and ultimately work as mechano-electric transducers. Thanks to their stereocilia, hair cells sense the local movement of the tectorial membrane induced by the underlying basilar membrane and respond by converting this displacement into ion-activated neurotransmitters that finally trigger the afferent nerve receptors (Fettiplace and Hackney, 2006). The central axis of the cochlea contains the auditory neurons which distribute their dendrites radially across the spiral to reach each segment of the basilar membrane (Figure 1B). The mechanism by which the hair cells stimulate the neurons is complex and relies on environmental factors generating the endocochlear potential (+80 mV) in the endolymphatic spaces of the scala media, which combines with the resting potentials of hair cells by giving rise to a massive 110–150 mV battery across hair cell membranes, which generates the highest electrochemical driving force in the body (Javel, 2003). In addition, the hair cells induce sound amplification (Le Masurier et al., 2005). According to mechanical tonotopy, high frequencies stimulate cochlear hair cells in the basal portion, and low frequencies in the apical portion of the cochlea (Figure 2A). This is due to the geometrical and mechanical characteristics of the basilar membrane, shorter (average section: 100 μm × 4.5 µm) and stiffer (E = 50 MPa) at the base, longer (average section: 500 μm × 1.5 µm) and softer (E = 3 MPa) at the apex of the cochlea, for a 28–40 mm total length (Figure 2B) (Liu et al., 2015). As a consequence of biomechanical cues, a sound wave, initially travelling at ≈100 m∙s−1 at the base of the cochlea, slows down as the mechanical impedance of the cochlea decreases and increases in amplitude. Near the resonance, where the stiffness and the mass compensate, the wavelength becomes short and the amplitude is maximum. All energy is spent to excite the hair cells and the wave breaks up. Basing on the sound stimuli (0–120 dB range), the basilar membrane can show displacements up to 100 nm.
FIGURE 2. (A) Schematic of an unrolled cochlea working at different frequency ranges (i.e., tonotopy); (B) 3 view design of a basilar membrane in its spiral conformation and size variation; (C,D) Schematics of a cochlear slice with application of a piezoelectric material in the form of: (C) a bulky film, and (D) a nanostructured device.
Hair cells are present in a predefined number at birth, about 12.000 outer and 3.500 inner hair cells, and unfortunately are not able to self-renew (Grandori and Martini, 1995). Such cells are terminally differentiated, and endogenous stem cells lack regenerative capacity (Simoni et al., 2017). Absence, dysfunction, impairment or death of hair cells represent the major causes of SNHL. This disease widely occurs due to inherited genetic conditions (60%). However, not all patients are eligible for or can truly benefit from treatments (Liu et al., 2023). In the current CI technology, the conversion of sound stimuli into electric signals is performed by a speech processor and a transmitter as an external device powered by a battery in which the sound is captured, processed and finally transmitted to a subcutaneous receiver; the latter is connected to the electrode array implanted inside the cochlea, which directly stimulates the auditory nerve (Copeland and Pillsbury, 2004). Since the seventies, different electrode arrays have been developed, with a variable number of electrodes (currently, 12-22) and configurations; however, recognition of speech in noisy environments or music appreciation remain the pinnacle of CI-implanted patients (Park, 2015). The battery to be daily charged is able to provide the electrode with an electric potential in the order of magnitude of volts, thus, much higher than the endocochlear potential. Owing to the extensive surgery, the permanent implantation of an electronic device, the health costs and the possible complications, CIs are implanted in severe-to-profound SNHL and often only unilaterally. Shortcomings of current CIs experienced by patients include loss of hearing frequencies (e.g., hearing sounds differently, hearing noise, losing of residual hearing, incomplete language understanding, difficulty in music perception, perceiving interactions with static electricity, magnetic fields, mobile phones, metal detectors and hearing aids), battery charge dependency, aesthetic problems and water unsuitability. Even if clinical benefits of CIs have remarkably improved over the past 50 years, hearing is still far from natural (Le Masurier et al., 2005). At research level, regenerative therapies (Park, 2015), as well as otoprotective drugs (Ciorba et al., 2008), are being investigated, but no clinically exploitable results have been found until now.
Piezoelectric materials possess the unique feature of generating electric charges via intrinsic and reversible polarization under mechanical force application, thus properly acting as mechano-electrical transducers (Danti, 2016). The possible transition from an electronics-based to a material-based CI has opened the intriguing possibility for an a-magnetic, fully implantable material with no need for an external energy supply (Mukherjee et al., 2000; Shintaku et al., 2010; Inaoka et al., 2011) (Figure 2C). Based on preliminary experiments on a 40 μm thick poly (vinylidene fluoride) (PVDF) film fixed on a substrate with a trapezoidal slit and a detecting electrode array, the function of frequency selectivity was demonstrated (Nakagawa and Kawano, 2014). After implantation in guinea pigs, such a piezoelectric membrane generated biological Auditory Brainstem Responses. A totally implantable device was then tested consisting of a silicon frame and a piezoelectric PVDF bulky film. However, the vibration amplitude in the piezoelectric film measured by laser doppler vibrometry, was smaller than that of the basilar membrane, thus yielding an insufficient sensitivity (Inaoka et al., 2011). On the other hand, ceramic materials with a perovskite-like structure, like lead zirconate titanate (PZT), possess higher piezoelectric properties than those of piezopolymers, but are very rigid and difficult to process, so they failed as a self-standing basilar membrane device, even though such PZT transducers were able to generate 45–60 μV with a vibration displacement of 7–17 nm at the frequency range of 100–1,600 Hz (Dufay et al., 2018). In addition, using PZT in implanted devices poses biocompatibility concerns. Among piezoceramics, BaTiO3 owns excellent piezoelectric properties exploited in piezoelectric actuators, capacitors and recently in biomedical devices (Candito et al., 2022). Nanostructured materials have shown the ability to modulate cell activity, by smartly combining topographical, chemical and physical cues in a single platform (Lang et al., 2016). Preliminary studies have demonstrated neural guidance by aligned nanofiber patterns in PVDF scaffolds enhancing neurite sprout induced by mechanically activated piezomaterials (Corey et al., 2007). While producing ultrafine fibers (typical diameter range: 10–1,000 nm), the electrospinning process can inherently apply mechanical stretching and a poling effect, which results to enhance the piezoelectric properties of piezopolymers. Nanocomposites based on piezoelectric ceramic nanoparticles as a filler and a piezopolymer as a matrix represent a strategy to increase the final piezoelectric properties (Magnani et al., 2022). By electrospinning 20% (w/w%) BaTiO3/PVDF and LiNbO3/PVDF-trifuoroethylene (PVDF-TrFE) nanocomposites, the piezoelectric coefficients and the electric voltage outputs were doubled with respect to those of the plain polymers (Mota et al., 2017; Danti et al., 2020). In 20/80 (w/w%) LiNbO3/PVDF-TrFE ultrafine fibers collected at 4,000 rpm, the resulting output voltage was 90 ± 2 mV and the thickness-normalized sensitivity was 1.06 mV/N‧µm (Danti et al., 2020). Moreover, 20/80 (w/w%) BaTiO3/PVDF nanocomposite fibers demonstrated improved viability of SH-SY5Y neural cells under mechanical motion (Mota et al., 2017), whereas LiNbO3 nanoparticles up to 50 μg/mL in the culture medium showed remarkable ability to reduce pro-inflammatory cytokines of epithelial cells and excellent otocompatibility with OC-k3 inner ear cells (Danti et al., 2020). Computational studies at different scales have described how different parameters play a role over the mechanical and electrical behaviour, although a comprehensive dissertation on electrospun fiber meshes is still missing. Scientists have also developed finite elements models to understand the macroscale behavior of the auditory apparatus, unveiling the chance to simulate the complex physics of the acoustic phenomena with different boundary conditions, however, such insight has not been yet combined with biomaterial-based approaches (Gan et al., 2007).
By comparing the electric output obtained by the PVDF slab device with that of CIs, a 1000-time difference has been highlighted. Therefore, four major challenges to be overcome for the future piezoelectric device have been pointed out: 1) Increase the output voltage; 2) Perform a proper fixation to the basilar membrane; 3) Increase the frequency selectivity, as it is naturally performed by outer hair cells that mechanically amplify low-level sound; and, 4) Increase the thickness to improve the piezoelectric performance (Nakagawa and Kawano, 2014). However, due to the small thickness of the basilar membrane, which varies both radially and longitudinally in 0.55–1.16 μm (Liu et al., 2015; Hrncirik et al., 2023), placing a slab even thicker than that used by Inaoka et al. (2011), i.e., 40 μm-thick PVDF film, is not realistic. In our opinion, developing thick membranes will not be an option, as this would generate a heavy device, difficult to be properly placed on site, thus hampering the basilar membrane vibration. Instead, using nanomaterials and nanotechnologies could be the way forward. On the nanoscale, many material properties can be enhanced by reaching the highest surface area to volume ratio, such as a highly distributed electric charge available for cell contact. In a larger-scale device than those ideally needed by the piezoelectric CI, PVDF nanofibers demonstrated to detect low frequencies with a sensitivity of 22.16 mV/Pa‧cm2 (Sarpeshkar et al., 1998).
In our perspective, a piezoelectric device made of nanostructured non-biodegradable biomaterials and based on the tissue engineering approach and its proper surgical implantation would promote a direct contact with spiral ganglion neurons, thus reducing the necessary electric output for neural stimulation (i.e., 3 orders-of-magnitude output voltage increase would not be necessary, few hundreds of mV would be sufficient to stimulate the neurons with a material nearly in contact with them) and finally achieving enough sensitivity. This would be reached by implanting a porous material acting as a scaffold for recruiting neural endings, not a bulky film (Figure 2D). PVDF and its copolymers are non-biodegradable and widely biocompatible, thus, they could act as a long-lasting implant (Azimi et al., 2020). The output voltage can be increased using nanoceramic/PVDF composites (Mota et al., 2017; Danti et al., 2020; Mokhtari et al., 2021). Several studies have proved the enhanced performance of piezoelectric nanocomposites in energy harvesting applications, using PVDF and nanoceramics, like reduced graphene oxide (rGO), which could also be suitable for biomedical purposes (Pusty et al., 2018; Shi et al., 2018). The optimal composition and nanostructured of piezoelectric nanocomposites should be defined by in silico models, which combine the biomaterial properties with the mechanical signals of the basilar membrane, as received in the cochlea, and the electric charged generated as a response. This would require additional efforts and multiscale modeling tools. Insertion and fixation to the basilar membrane remains a key challenge, as the best piezoelectric device placement should be in an upside-down position, i.e., via the scala tympani, as the scala media is smaller and residual hair cells should be preserved. Possibly, binding agents could be used to this purpose. Another challenge should consider that the locations of the neural endings is close to cochlear axis; thus, it is not distributed along the full area of the basilar membrane. By maximizing the piezoelectric material contact with the basilar membrane and the neurites, i.e., via a nanostructured scaffold, like electrospun fiber meshes, the frequency selectivity might be improved. Overall, the piezoelectric material-based CI would be self-powered (no need for battery) and less expensive (only 4 cm of biomaterial is needed, no software, no electronics), fully implantable with a less invasive surgery (since no device part will be implanted in the skull bone, as in conventional CIs, but only the electrode part inside the cochlea), preserve and even boost residual sensory function. This configuration would make hearing ability available for a broader range of patients, including those who do not meet the medical and audiological criteria for current CI implantation, e.g., the elderly affected by presbycusis, who could better fight social isolation and/or slow cognitive decline (Amieva et al., 2015; Lin et al., 2018). Today, receiving a CI depends very much on local health system regulations and socio-economic circumstances. As such, we hope to stimulate research in this field (Gfeller et al., 2002).
The original contributions presented in the study are included in the article/supplementary material, further inquiries can be directed to the corresponding author.
SD: Conceptualization, Data curation, Formal Analysis, Investigation, Project administration, Visualization, Writing–original draft, Writing–review and editing. SB: Conceptualization, Funding acquisition, Supervision, Writing–review and editing.
The author(s) declare financial support was received for the research, authorship, and/or publication of this article. Funded by European Union–Next-Generation EU via the Italian Ministry of University and Research (MUR), PRIN 2022 program (PROMISE project, CUP 153D23004700006).
Authors acknowledge the MIT-UNIPI collaboration for piezoelectric nanofiber modeling (NANOSPARK project, 2017). The Center for Instrumentation Sharing—University of Pisa (CISUP) is acknowledged for SEM analysis. Dr. Delfo D’Alessandro and Dr. Mario Milazzo (University of Pisa) are acknowledged for their support to basilar membrane images.
The authors declare that the research was conducted in the absence of any commercial or financial relationships that could be construed as a potential conflict of interest.
The author(s) declared that they were an editorial board member of Frontiers, at the time of submission. This had no impact on the peer review process and the final decision.
All claims expressed in this article are solely those of the authors and do not necessarily represent those of their affiliated organizations, or those of the publisher, the editors and the reviewers. Any product that may be evaluated in this article, or claim that may be made by its manufacturer, is not guaranteed or endorsed by the publisher.
Amieva, H., Ouvrard, C., Giulioli, C., Meillon, C., Rullier, L., and Dartigues, J. F. (2015). Self-reported hearing loss, hearing aids, and cognitive decline in elderly adults: a 25-year study. J. Am. Geriatr. Soc. 63 (10), 2099–2104. doi:10.1111/jgs.13649
Azimi, B., Sorayani Bafqi, M. S., Fusco, A., Ricci, C., Gallone, G., Bagherzadeh, R., et al. (2020). Electrospun ZnO/Poly(Vinylidene fluoride-trifluoroethylene) scaffolds for lung tissue engineering. Tissue Eng. A 26 (23-24), 1312–1331. doi:10.1089/ten.TEA.2020.0172
Berrettini, S., Arslan, E., Baggiani, A., Burdo, S., Cassandro, E., Cuda, D., et al. (2011). Analysis of the impact of professional involvement in evidence generation for the HTA Process, subproject "cochlear implants": methodology, results and recommendations. Acta Otorhinolaryngol. Ital. 31 (5), 273–280.
Candito, M., Simoni, E., Gentilin, E., Martini, A., Marioni, G., Danti, S., et al. (2022). Neuron compatibility and antioxidant activity of barium titanate and lithium niobate nanoparticles. Int. J. Mol. Sci. 23 (3), 1761. doi:10.3390/ijms23031761
Carlyon, R. P., and Goehring, T. (2021). Cochlear implant research and development in the twenty-first century: a critical update. JARO 22, 481–508. doi:10.1007/s10162-021-00811-5
Ciorba, A., Astolfi, L., Jolly, C., and Martini, A. (2009). Review paper: cochlear implants and inner ear based therapy. Eur. J. Nanomed. 2 (2), 25–28. doi:10.1515/ejnm.2009.2.2.25
Ciorba, A., Astolfi, L., and Martini, A. (2008). Otoprotection and inner ear regeneration. Audiol. Med. 6 (3), 170–175. doi:10.1080/16513860802410806
Copeland, B. J., and Pillsbury, H. C. (2004). Cochlear implantation for the treatment of deafness. Annu. Rev. Med. 55, 157–167. doi:10.1146/annurev.med.55.091902.105251
Corey, J. M., Lin, D. Y., Mycek, K. B., Chen, Q., Samuel, S., Feldman, E. L., et al. (2007). Aligned electrospun nanofibers specify the direction of dorsal root ganglia neurite growth. J. Biomed. Mat. Res. A 83 (3), 636–645. doi:10.1002/jbm.a.31285
Danti, S. (2016). “Boron nitride nanotubes as nanotransducers,” in Boron nitride nanotubes in nanomedicine. Editors G. Ciofani, and V. Mattoli (Elsevier Book, Amsterdam, Netherlands), 123–138. doi:10.1016/C2014-0-04018-X
Danti, S., Azimi, B., Candito, M., Fusco, A., Sorayani Bafqi, M. S., Ricci, C., et al. (2020). Lithium niobate nanoparticles as biofunctional interface material for inner ear devices. Biointerphases 15 (3), 031004. doi:10.1116/6.0000067
Dufay, T., Guiffard, B., Seveno, R., and Thomas, J. C. (2018). Energy harvesting using a lead zirconate titanate (PZT) thin film on a polymer substrate. Energy Technol. 6 (5), 917–921. doi:10.1002/ente.201700732
Fettiplace, R., and Hackney, C. M. (2006). The sensory and motor roles of auditory hair cells. Nat. Rev. Neurosci. 7 (1), 19–29. doi:10.1038/nrn1828
Forli, F., Arslan, E., Bellelli, S., Burdo, S., Mancini, P., Martini, A., et al. (2011). Systematic review of the literature on the clinical effectiveness of the cochlear implant procedure in paediatric patients. Acta Otorhinolaryngol. Ital. 31 (5), 281–298.
Gan, R. Z., Reeves, B. P., and Wang, X. (2007). Modeling of sound transmission from ear canal to cochlea. Ann. Biomed. Eng. 35 (12), 2180–2195. doi:10.1007/s10439-007-9366-y
Gfeller, K., Turner, C., Mehr, M., Woodworth, G., Fearn, R., Knutson, J. F., et al. (2002). Recognition of familiar melodies by adult cochlear implant recipients and normal-hearing adults. Cochlear Implants Int. 3 (1), 29–53. doi:10.1002/cii.50
Grandori, F., and Martini, A. (1995). Potenziali evocati uditivi. Basi teoriche e applicazioni cliniche. Padua, Italy: Piccin Nuova Libraria. ISBN: 8829910724.
Hrncirik, F., Roberts, I., Sevgili, I., Swords, C., and Bance, M. (2023). Models of cochlea used in cochlear implant research: a review. Ann. Biomed. Eng. 51 (7), 1390–1407. doi:10.1007/s10439-023-03192-3
Inaoka, T., Shintaku, H., Nakagawa, T., Kawano, S., Ogita, H., Sakamoto, T., et al. (2011). Piezoelectric materials mimic the function of the cochlear sensory epithelium. Proc. Natl. Acad. Sci. U. S. A. 108 (45), 18390–18395. doi:10.1073/pnas.1110036108
Javel, E. (2003). “Auditory system, peripheral,” in Encyclopedia of the neurological sciences. Editor(s): M. J. Aminoff, and R. B. Daroff (Academic Press, Cambridge, MA, USA), 305–311. doi:10.1016/B0-12-226870-9/00529-3
Lang, C., Fang, J., Shao, H., Ding, X., and Lin, T. (2016). High-sensitivity acoustic sensors from nanofibre webs. Nat. Commun. 7 (1), 11108. doi:10.1038/ncomms11108
Latif, R., Noor, M. M., Yunas, J., and Hamzah, A. A. (2021). Mechanical energy sensing and harvesting in micromachined polymer-based piezoelectric transducers for fully implanted hearing systems: a review. Polym. (Basel) 13 (14), 2276. doi:10.3390/polym13142276
Le Masurier, M., and Gillespie, P. G. (2005). Hair-cell mechanotransduction and cochlear amplification. Neuron 48 (3), 403–415. doi:10.1016/j.neuron.2005.10.017
Lin, H. W., Mahboubi, H., and Bhattacharyya, N. (2018). Self-reported hearing difficulty and risk of accidental injury in US adults, 2007 to 2015. JAMA Otolaryngol. Head. Neck Surg. 144 (5), 413–417. doi:10.1001/jamaoto.2018.0039
Liu, F., Han, B., Zhou, X., Huang, S., and Huang, J. (2023). Research progress on the treatment and nursing of sensorineural hearing loss. Front. Neurosci. 17, 1199946. doi:10.3389/fnins.2023.1199946
Liu, W., Atturo, F., Aldaya, R., Santi, P., Cureoglu, S., Obwegeser, S., et al. (2015). Macromolecular organization and fine structure of the human basilar membrane - relevance for cochlear implantation. Cell. Tissue Res. 360 (2), 245–262. doi:10.1007/s00441-014-2098-z
Magnani, A., Capaccioli, S., Azimi, B., Danti, S., and Labardi, M. (2022). Local piezoelectric response of polymer/ceramic nanocomposite fibers. Polym. (Basel) 14 (24), 5379. doi:10.3390/polym14245379
Mokhtari, F., Azimi, B., Salehi, M., Hashemikia, S., and Danti, S. (2021). Recent advances of polymer-based piezoelectric composites for biomedical applications. J. Mech. Behav. Biomed. Mat. 122, 104669. doi:10.1016/j.jmbbm.2021.104669
Mota, C., Labardi, M., Trombi, L., Astolfi, L., D’Acunto, M., Puppi, D., et al. (2017). Design, fabrication and characterization of composite piezoelectric ultrafine fibers for cochlear stimulation. Mater. Des. 122, 206–219. doi:10.1016/j.matdes.2017.03.013
Mukherjee, N., Roseman, R. D., and Willging, J. P. (2000). The piezoelectric cochlear implant: concept, feasibility, challenges, and issues. J. Biomed. Mat. Res. 53 (2), 181–187. doi:10.1002/(sici)1097-4636(2000)53:2<181::aid-jbm8>3.0.co;2-t
Nakagawa, T., and Kawano, S. (2014). “Artificial cochlear epithelium,” in Regenerative medicine for the inner ear. Editor J. Ito (Springer, Berlin,Germany), 157–164. doi:10.1007/978-4-431-54862-1_17
Park, Y.-H. (2015). Stem cell therapy for sensorineural hearing loss, still alive? J. Audiol. Otol. 19 (2), 63–67. doi:10.7874/jao.2015.19.2.63
Pusty, M., Sinha, L., and Shirage, P. M. (2019). A flexible self-poled piezoelectric nanogenerator based on a rGO–Ag/PVDF nanocomposite. New J. Chem. 43 (1), 284–294. doi:10.1039/C8NJ04751K
Sarpeshkar, R., Lyon, R. F., and Mead, C. (1998). “A low-power wide-dynamic-range analog VLSI cochlea,” in Neuromorphic systems engineering: neural networks in silicon (Boston, MA, USA: Kluwer Academic), 49–103.
Shi, K., Sun, B., Huang, X., and Jiang, P. (2018). Synergistic effect of graphene nanosheet and BaTiO3 nanoparticles on performance enhancement of electrospun PVDF nanofiber mat for flexible piezoelectric nanogenerators. Nano Energy 52, 153–162. doi:10.1016/j.nanoen.2018.07.053
Shintaku, H., Nakagawa, T., Kitagawa, D., Tanujaya, H., Kawano, S., and Ito, J. (2010). Development of piezoelectric acoustic sensor with frequency selectivity for artificial cochlea. Sens. Actuators A Phys. 158 (2), 183–192. doi:10.1016/j.sna.2009.12.021
Simoni, E., Orsini, G., Chicca, M., Bettini, S., Franceschini, V., Martini, A., et al. (2017). Regenerative medicine in hearing recovery. Cytotherapy 9 (8), 909–915. doi:10.1016/j.jcyt.2017.04.008
Tanna, R. J., Lin, W. J., and De Jesus, O. (2024). Sensorineural hearing loss. Treasure Island, FL, USA: StatPearls Publishing. https://www.ncbi.nlm.nih.gov/books/NBK565860/.
Von Bekesy, G. (1970). Travelling waves as frequency analysers in the cochlea. Nature 225 (5239), 1207–1209. doi:10.1038/2251207a0
WHO Website (2023). Deafness and hearing loss. https://www.who.int/news-room/fact-sheets/detail/deafness-and-hearing-loss (accessed on January 17, 2024).
Keywords: sensorineural hearing loss, hair cells, electrospinning, piezoelectric polymers, nanocomposites
Citation: Danti S and Berrettini S (2024) New perspectives for piezoelectric material-based cochlear implants: getting to nano. Front. Mater. 11:1362141. doi: 10.3389/fmats.2024.1362141
Received: 27 December 2023; Accepted: 05 February 2024;
Published: 23 February 2024.
Edited by:
Giulia Masi, University of Bologna, ItalyReviewed by:
Dimitrios Kontziampasis, University of Dundee, United KingdomCopyright © 2024 Danti and Berrettini. This is an open-access article distributed under the terms of the Creative Commons Attribution License (CC BY). The use, distribution or reproduction in other forums is permitted, provided the original author(s) and the copyright owner(s) are credited and that the original publication in this journal is cited, in accordance with accepted academic practice. No use, distribution or reproduction is permitted which does not comply with these terms.
*Correspondence: Serena Danti, c2VyZW5hLmRhbnRpQHVuaXBpLml0
Disclaimer: All claims expressed in this article are solely those of the authors and do not necessarily represent those of their affiliated organizations, or those of the publisher, the editors and the reviewers. Any product that may be evaluated in this article or claim that may be made by its manufacturer is not guaranteed or endorsed by the publisher.
Research integrity at Frontiers
Learn more about the work of our research integrity team to safeguard the quality of each article we publish.