- 1Shanxi Medical University, Taiyuan, Shanxi, China
- 2The First Hospital of Shanxi Medical University, Taiyuan, Shanxi, China
Objective: This study investigates the antibacterial mechanisms of medical nanosilver latex materials by conducting antibacterial experiments on nanosilver latex, observing the morphological changes in Escherichia coli after co-cultivation with nanosilver, and detecting variations in nitric oxide, malondialdehyde, protein, and DNA fragment content. The aim is to provide a theoretical foundation for the application of antibacterial materials in medical settings.
Methods: To prepare the composite latex film, nanosilver was combined with natural latex. The antibacterial efficacy of nanosilver latex were assessed using co-cultivation and colony counting methods. Electron microscopy facilitated the observation of Escherichia coli post co-cultivation with nanosilver, and subsequent changes in nitric oxide, malondialdehyde, protein, and DNA fragment content were measured.
Results: The composite film of nanosilver latex demonstrated antibacterial properties, which were proportional to its concentration and inversely proportional to the particle size. Exposure to nanosilver resulted in bacterial cell membranes, leading to increased levels of nitric oxide, malondialdehyde, protein, and DNA content within bacteria.
Conclusion: Nanosilver particles, especially those of 10 nm, were found to be most effective in antibacterial activity. The antibacterial effects were primarily due to the disruption of bacterial cell membranes and walls, altering intra and extracellular osmotic pressure, inducing lipid peroxidation of bacterial cell membranes, triggering of oxidative stress, and damage to DNA.
1 Introduction
With the rapid advancement of modern medical technology, the use of human body implants has become increasingly common and essential for disease diagnosis and treatment, significantly enhancing treatment outcomes. However, these implanted devices, as foreign objects, are prone to causing infections due to improper handling or prolonged placement. Catheter-related bloodstream infections, catheter-related urinary tract infections, urinary tract infections, artificial heart valve infections, and orthopedic prosthetic joint infections represent approximately 45% of hospital-acquired infections (Yang et al., 2003). Addressing these infections is challenging, especially with recurrent cases, as prolonged implantation offers a substrate for bacterial colonization and biofilm formation (Bjarnsholt et al., 2018; Xu et al., 2019; Yan et al., 2023; Bjarnsholt et al., 2018; Xu et al., 2019; Yan et al., 2023). This complicates antibiotic treatment, increases antibiotic usage, and raises the risk of bacterial resistance. The improper utilization of implanted medical devices adversely affects patient prognosis and escalates healthcare costs. To mitigate infections associated with implanted devices, extensive research is being conducted on developing antibacterial materials to minimize bacterial colonization and proliferation, thus reducing infection rates.
In the pursuit of novel antibacterial materials, significant strides have been made in nanotechnology and nanomaterials. Owing to their unique surface and interface effects, small size effects, quantum size effects, and macroscopic quantum tunneling effects, nanomaterials enable precise control over physical properties such as size, surface chemistry, and shape, making them widely applicable (Wu et al., 2020). In medicine, nanometallic elements and their oxides are extensively utilized for their antibacterial and antiviral properties—for example, nano silver, nano copper oxide, and nano zinc oxide (Ma and Hou, 2022; Yang et al., 2022; Xiang and Zheng, 2009; Cai et al., 2020). Nanosilver (Ag NPs), in particular, garners attention due to its lower minimum inhibitory concentration compared to conventional antibiotics (Maomao et al., 2023) and its eco-friendly synthesis while being capable of interfacing with various metals to combat bacteria (Peng, 2020; Rather et al., 2021; Mueez et al., 2022). Considering the wide array of implanted devices, catheters, which frequently contact the external environment, are a focus of this study, particularly ureteral stent materials. We prepare natural latex mixed with nanosilver as a medical antibacterial material and test its efficacy. Co-cultivation of nanosilver with bacteria facilitates observations of bacterial morphology, alterations in cell membranes, internal alterations, and the measurement of nitric oxide, malondialdehyde, protein, and DNA fragment content. These experiments aim to enhance the understanding of the antibacterial mechanism of nanosilver and provide critical theoretical insights for the development of medical antibacterial materials based on nanomaterials, thereby offering innovative solutions for preventing and controlling hospital-acquired infections, such as catheter-related infections.
2 Materials and methods
2.1 Experimental materials and instruments
This section details the materials and instruments utilized in the experiment: Nanosilver, Natural latex, Artificial urine (YY0325-2016), Bacteria (Escherichia coli, Pseudomonas aeruginosa, Klebsiella pneumoniae, Staphylococcus aureus, Enterococcus faecalis, and Staphylococcus epidermidis), UV-VIS spectrophotometer (UV-1800), Nitric oxide test reagent (S0021M), Lipid peroxidation (MDA) test reagent kit (S0131M), Bradford protein concentration assay reagent kit (P0006), Diphenylamine DNA quantitative test reagent kit (HL20014).
2.2 Preparation of nanosilver latex film
The preparation process for nanosilver latex film began by filtering natural latex through a 120 mesh sieve, then pouring it into a three-necked flask. It was consistently stirred at 400 rpm on a magnetic stirrer and de-ammoniated under closed conditions using nitrogen. Distinct sizes of nanosilver particles (10 nm, 15 nm, and 20 nm) were separately suspended in 2 mL of deionized water at varying concentrations (4 mg, 8 mg, 16 mg, and 32 mg) and sonicated for 1 h at 40 kHz and 40°C, resulting in 12 groups of nanosilver colloids. This produced 12 groups of nanosilver colloids. 8 mL of de-ammoniated natural latex was added to the prepared nanosilver colloid and stirred at a constant rate of 300 rpm for 10 min to obtain nanosilver latex. 3 mL of nanosilver latex was added to 60 mm culture dishes and ventilated for 72 h to obtain nanosilver latex composite films, approximately 1 mm thick, generating 12 experimental film groups (i.e., , 10 nm, 2 mg/mL group; 10 nm, 4 mg/mL group; 10 nm, 8 mg/mL group; 10 nm, 16 mg/mL group; 15 nm, 2 mg/mL group; 15 nm, 4 mg/mL group; 15 nm, 8 mg/mL group; 15 nm, 16 mg/mL group; 20 nm, 2 mg/mL group; 20 nm, 4 mg/mL group; 20 nm, 8 mg/mL group; 20 nm, 16 mg/mL group) denoted as A1, A2, A3, A4, B1, B2, B3, B4, C1, C2, C3, C4 groups. An equal volume of deionized water added to natural latex served as the control group, denoted as X. Dried films were cut into 8 mm circular pieces using a perforator, totaling 13 groups. Preparation process is shown in Figure 1.
2.3 Antibacterial performance experiment of nanosilver latex film
For the antibacterial experiment, six pathogenic bacteria commonly associated with catheter-related infections were selected (Huang, 2023). Following the research group’s previous (Zixiang et al., 2019a),8 mm film pieces from each group were placed into a 48-well plate. Each well was inoculated with 50 μL of bacterial suspension at a standardized McFarland turbidity standard of 0.5. The plates were incubated at 37°C for 12–18 h. Colony counts were performed post incubation by diluting the bacteria with PBS, and antibacterial rates were calculated. This experiment was replicated three times under the same conditions at different time intervals.
Antibacterial rate (%) = (number of colonies in control group - number of colonies in experimental group)/number of colonies in control group x 100%
2.4 Stability experiment of nanosilver latex film
Latex films from the experimental group were placed in centrifuge tubes containing 4 mL of artificial urine and stored at 37°C for 15 days. UV-VIS spectrophotometry was employed to detect the detachment of metal ions or oxides detachment from the highest concentration group. Metal ion release was assessed using heavy metal ion reagents. Blank artificial urine served as the control.
2.5 Observation of bacterial morphology, cell membrane, internal changes, and determination of bacterial internal substance content after co-cultivation of nanosilver with bacteria
Optimal particle size of silver nanoparticles showing antibacterial activity was cultured with 1 ± 0.5 mg/mL concentration and 0.5 McFarland standard turbidity Escherichia coli as the experimental group, and Escherichia coli as the control group.
2.6.1: After 12 h of shaking incubation at 37°C, the co-cultures were centrifuged and observed using electron microscopy.
2.6.2: After 24 h of incubation, the supernatant was extracted from both the experimental and control groups by ultrasonic disruption, followed by refrigerated centrifugation. A UV spectrophotometer was then used to determine differences in substance content, specifically nitric oxide (NO), malondialdehyde (MDA), protein, and DNA fragment content.
2.6.3: After 4 h of shock culture, cell membrane integrity was assessed using propidium iodide/acridine orange (PI/AO).
2.6 Statistical analysis
Data were analyzed using SPSS 27.0 software for statistical description and analysis. Descriptive statistics or quartiles were used to represent metric data based on normal distribution.
3 Results
3.1 Antibacterial performance experiment of nanosilver latex film
Table 1 outlines the antibacterial experiment results of the nanosilver latex composite film. The results demonstrate that the composite film exhibits notable antibacterial properties, particularly against Escherichia coli and Klebsiella pneumoniae. A graphical representation of the antibacterial effects of nanosilver latex composite film shows an increase in antibacterial activity with rising concentration (Figure 2A) and a decrease with increasing particle size (Figure 2B).
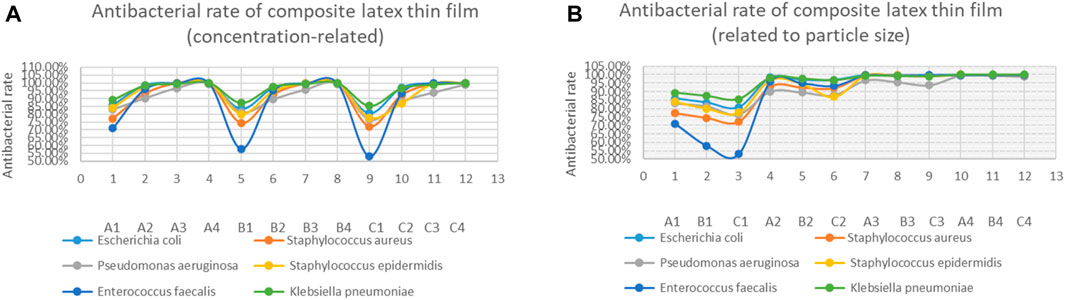
FIGURE 2. Antibacterial results statistics of composite latex film. (A) illustrates concentration-related antibacterial rates, and (B) depicts antibacterial rates related to the particle size.
3.2 Stability experiment results of nanosilver latex film
The colorimetric reaction of the heavy metal test reagent with metal ions indicated metal ion release. Positive results appeared red (Control Group 2), while negative results appeared yellow (Control Group 1). All composite films showed negative results after storage in artificial urine under simulated body conditions (Figure 3). UV-VIS spectrophotometry revealed no detachment of pure nanosilver from the composite latex film (Figure 4).

FIGURE 3. Heavy metal ion detection reagent results in latex film soaking solution; control group 1: artificial urine group; control group 2: nano-silver + artificial urine group.
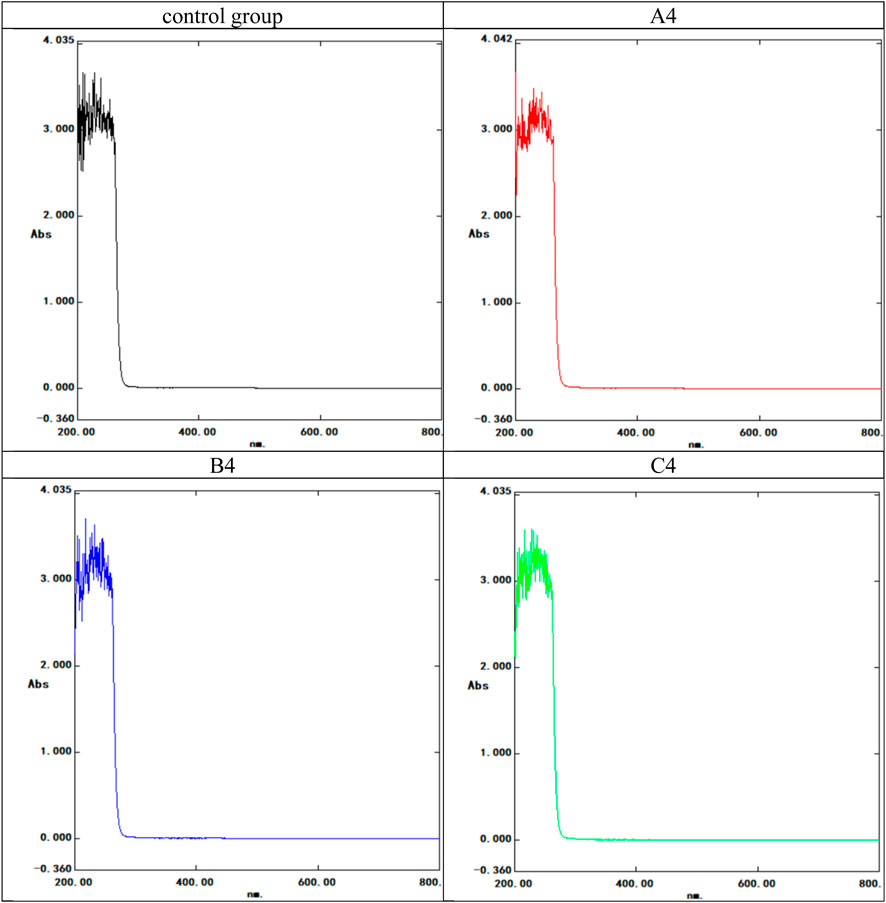
FIGURE 4. UV spectrophotometer spectral curve of the composite latex film soaking solution. The control group used artificial urine; the experimental groups included the three highest concentration. A4: 10 nm, 16 mg/mL group; B4: 15 nm, 16 mg/mL group; C4: 20 nm, 16 mg/mL group. Horizontal coordinate: nm; Ordinate: Absorbance (Abs).
3.3 Observation of bacterial morphology, cell membrane, internal changes, and determination of bacterial internal substance content after co-cultivation of nanosilver with bacteria
Considering the antibacterial results, nanosilver with 10 nm diameter exhibited the most effective antibacterial performance. Consequently, 10 nm nanosilver was selected for this segment of the experiment. The bacteria chosen were Escherichia coli, commonly found in urinary system infections.
3.3.1 Electron microscope result
Scanning Electron Microscopy (SEM) demonstrated swollen and expanded bacteria, shallower surface patterns, fissures, and direct rupture in bacterial cells in the experimental group, in contrast to the control group (Figure 5: 1. 2. 3. 4). Ransmission Electron Microscopy (TEM) revealed dispersed bacterial nuclei lacking highly folded regions in the experimental group, unlike the denser nuclei in the control group. This indicated restricted bacterial growth in the experimental group (Figure 5: 5. 6. 7. 8).
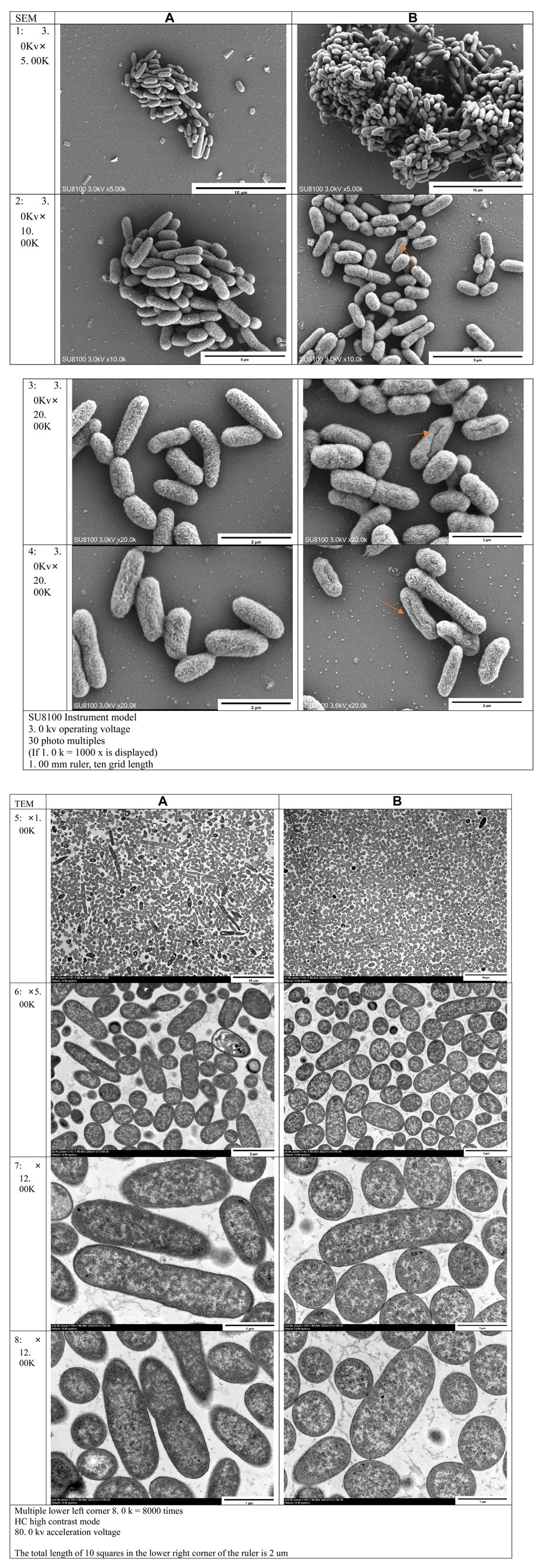
FIGURE 5. Electron microscope observation results: (A) Control group; (B) Experimental group 1–4 were the results of scanning electron microscopy (SEM), five to eight were the results of transmission electron microscopy (TEM)
3.3.2 UV-VIS spectrophotometry results
UV-VIS spectrophotometry analysis indicated differences in substance content between the experimental and control groups, particularly at 280 nm (Figure 6), suggesting implications for subsequent experiments. Control group: 280 nm absorbance 0.0831; Experimental group: 280 nm absorbance 0.1370.
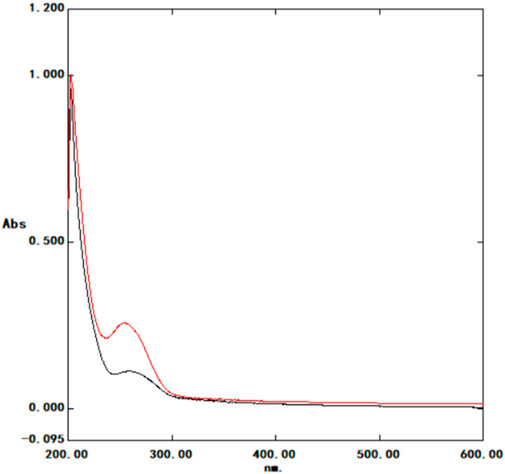
FIGURE 6. U Ultraviolet spectrophotometer spectral curve Black: control group; red: experimental group; control group: 280 nm absorbance 0.0831; experimental group: 280 nm absorbance 0.1370. Horizontal coordinate: nm; Ordinate: Absorbance (Abs).
3.3.3 Biochemical test results
The biochemical test results showed statistically significant differences (p < 0.05) in nitric oxide, malondialdehyde, DNA, and protein content between the experimental and control groups (Table 2).
The table shows the Optical density (OD)value.
3.3.4 PI/AO Result
The PI/AO results are displayed in Figure 7. The experimental group (Figure B) exhibited a significant increase in the number of red bacteria compared to the control group (Figure A), indicating bacterial death and compromised membrane integrity.
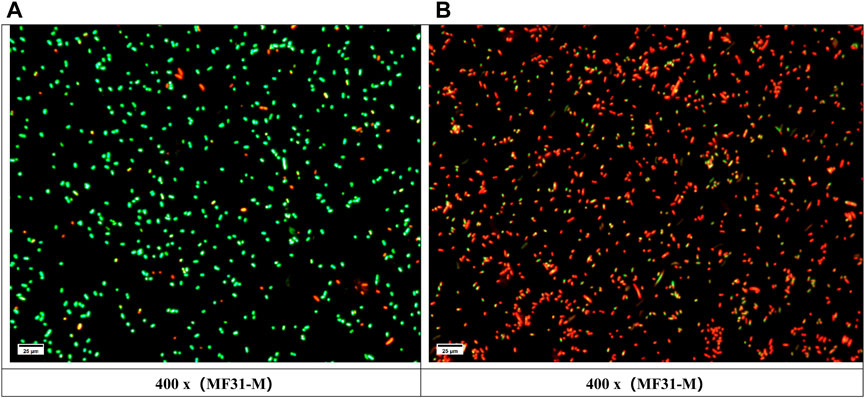
FIGURE 7. PI/AO test results: Green represents living cells, red represents dead cells; (A) Control group; (B) Experimental group.
4 Discussion
The use of medical implants and interventions has been instrumental in advancing medical treatments, improving diagnostic and therapeutic outcomes, alleviating patient suffering, enhancing quality of life, and furthering the development of medical technologies and disease management. However, the placement of implants or interventions within the human body, due to surgical procedures and patient conditions, poses a risk of bacterial infection at the interface between the implanted or intervened material and the surrounding tissues. Such infections can be localized or extensive, potentially leading systemic infections. Implant and intervention-related infections present a significant healthcare challenge due to their difficult treatment, time-consuming nature, and high costs. Severe cases may necessitate surgical intervention, implant removal, or prolonged antibiotic treatments (O’cearbhaill, 2019; Dachi, 2022; Fairweather et al., 2020). Significantly increasing the economic burden on both patients and healthcare systems. Furthermore, increased antibiotic use may lead to the emergence of resistant bacterial strains, complicating treatments, escalating costs, and posing additional risks to patient health.
In response to these challenges, alongside stringent aseptic procedures and meticulous management of implant-related infections, the use of antimicrobial implants has become a crucial preventive strategy. Currently, research focuses on six main modalities: ①: antimicrobial coating technology; ②: nanomaterials; ③: antimicrobial biomaterials; ④: electroactive materials; ⑤: antimicrobial drug delivery systems; and⑥: antimicrobial surface texture design. Among these, antimicrobial nanomaterials, particularly nano-silver, have garnered widespread attention due to their high efficiency, safety, stability, and versatility. Researchers highlight that nanosilver, in addition to typical nanomaterial properties, offers significant surface area, strong penetrative ability, and resistance to oxidation, (Liu et al., 2023a), aids in tissue repair, and accelerates wound healing, leading to its application in treating burns, ulcers, surgical incisions, and more (Zhou et al., 2019; Liu et al., 2023b; Prabhu et al., 2023; Zhang, 2023). Nanosilver also possesses anti-inflammatory properties, reducing inflammation, alleviating swelling and pain, andcontributing to the treatment of inflammatory conditions such as arthritis and dermatitis (Andleeb et al., 2023; Kurup et al., 2023).
Due to the cytotoxic effects of nanosilver (Azizi et al., 2023), it requires combination with other materials and processes like oxidation-reduction to mitigate cytotoxicity (Zhai et al., 2011; Zixiang et al., 2019b). This study utilizes natural latex, known for its excellent biocompatibility and processability in medical catheters, mixed with nanosilver to combat the heightened risk of catheter-related infections during implantation. Consequently, research into antibacterial latex catheters has intensified. Prior studies have documented the antibacterial properties of chitosan nanosilver latex (Xu, 2021; JIA et al., 2022; Kai, 2022) and the wound-healing enhancement of natural latex nanosilver pillow cores (Lu et al., 2017). However, limited research into the antibacterial mechanism of nanosilver latex and the determination of its optimal particle size persists, leading to ongoing debate. The nanosilver utilized this research varies from tens to hundreds of nanometers. While some studies indicate that antibacterial efficacy gradually increases with particle sizes ranging from 1 to 10 nm, it tends to decrease for sizes between 20–100 nm (Zuo et al., 2013). Conversely, other researchers advocate that the most effective antibacterial properties of nanometals and their oxides occur within the 10–20 nm range.
Results from antibacterial experiments demonstrate that nanosilver latex films exert significant antibacterial effects against six common pathogens associated with medical infections. At a concentration of 0.4 mg/100 mL, the composite film already exhibits pronounced antibacterial properties. The experimental findings suggest that the antibacterial effect of the composite film is directly proportional to the concentration of nanosilver and inversely proportional to particle size. This effect is likely attributed to the fact that smaller particle sizes have larger relative surface areas, inflicting more substantial physical damage and enhanced oxidative stress on bacteria, culminating in increased bacterial damage.
Based on these study results, outcomes, it is posited that nanosilver materials with a particle size of 10 nm are most effective in terms of antibacterial properties. Stability experiments indicate that the composite latex film does not release metal ions or shed metallic elements, underscoring the method’s stability. Such stability ensures that nanosilver remains embedded within the film, mitigating risks of toxicity or physical damage in other areas. This finding indirectly confirms the safety of the method, aligning with prior experimental results from the research group that verified the biocompatibility of latex and nanosilver, meeting medical equipment safety standards (Xu, 2022).
Various perspectives exist regarding the antibacterial mechanism of nanosilver. ①: Physical damage to bacterial cell membrane (Bahar et al., 2017; LI et al., 2017; Yao et al., 2019); ②: Chemical damage to bacterial cell components (Friedman et al., 2011; Rizzello and Pompa, 2014); ③: DNA damage (Yn et al., 2017); ④: Disruption of the respiratory chain (Tan et al., 2020); ⑤: Catalytic action for sterilization (Guo et al., 2023); ⑥: Inhibition of cell division (Sanyasi et al., 2016; Guo et al., 2019; Zhang et al., 2023). Despite ongoing debate over the exact mechanism, scanning electron microscopy (SEM) images in this experiment demonstrate that nanosilver affects the osmotic pressure inside and outside bacteria, causing swelling and potential rupture, and consequently leading to the leakage of bacterial contents. Escherichia coli, protected by its cell wall, does not exhibit swelling and rupture, suggesting that these changes are primarily due to nanosilver particles physicallydamaging the bacterial cell wall and cell membrane. Transmission electron microscope (TEM) images further show that in addition to osmotic pressure changes causing bacterial swelling, nanosilver significantly restricts bacterial growth, leading to looser nucleoids This is likely attributable to the destruction of DNA or replication pathways. Further analysis reveals a substantial increase in malondialdehyde (MDA) content in Escherichia coli after mixed cultivation with nanosilver, indicating lipid peroxidation in the bacterial membrane and suggesting a disruption of the membrane’s defense mechanism. Given that lipids are predominantly located in the bacterial cell membrane, the PI/AO results further indicate that the cell membrane was compromised. It can be inferred that nanosilver induces lipid peroxidation in the Escherichia coli membrane, likely disrupting the membrane’s defense mechanism. Additionally, nanosilver triggers oxidative stress and DNA damage within the bacteria, with an increase in protein content suggesting a rise in superoxide dismutase activity to mitigate oxidative stress. In summary, nanosilver’s antibacterial action results from damaging bacterial cell membranes and walls, altering osmotic pressure both internally and externally in bacteria, inducing lipid peroxidation in bacterial cell membranes, triggering oxidative stress, and causing DNA damage. These findings are consistent with other scholars’ research (Friedman et al., 2011; Rizzello and Pompa, 2014; Li et al., 2017; Yn et al., 2017). The noted alteration in osmotic pressure, a novel discovery in this study, may be due to nanosilver particles directly penetrating bacteria, potentially explaining the reduced antibacterial effectiveness of larger nanosilver particles. Moreover, the observation of bacterial division in SEM results implies that the role of physical damage as the predominant antibacterial mechanism is contentious. Since only a minority of bacteria display physical damage, it is plausible to assert that physical damage is not the primary antibacterial mechanism of nanosilver. When these results are viewed in conjunction with other research, oxidative stress emerges as the likely principal antibacterial mechanism of nanosilver.
Regarding whether nanosilver and other nanometals might induce bacterial resistance akin to antibiotics, current research posits bacteria are unlikely to develop resistance to nanometals and their oxides. However, some scholars outline potential mechanisms by which bacteria could gradually adapt and develop tolerance to nanometals and their oxides: 1: Horizontal gene transfer (HGT) (Zha et al., 2022; Zhang et al., 2022); 2: Prevention of ion release (Radzig et al., 2013; Blair et al., 2015; Stabryla et al., 2021); 3: Reshaping of the cell membrane (Zhu et al., 2014; Kotchaplai et al., 2017; Wu et al., 2019). It is important to note that, while no definitive evidence currently exists that bacteria will develop tolerance to nanometals and their oxides, conclusive findings necessitate further investigation. The field continues to evolve, marked by active debates and discussions within the scientific community.
5 Conclusion
Nanosilver latex composite film has excellent antibacterial effect and stability. The antibacterial effect of 10 nm-sized nanosilver is optimal. Nanosilver achieves its antibacterial effect by damaging bacterial cell membranes and walls, altering osmotic pressure inside and outside bacteria, inducing lipid peroxidation in bacterial cell membranes, triggering oxidative stress, and damaging DNA.
Data availability statement
The datasets presented in this study can be found in online repositories. The names of the repository/repositories and accession number(s) can be found in the article/Supplementary material.
Author contributions
ZM: Writing–original draft. CL: Writing–review and editing. BF: Writing–review and editing. PY: Writing–review and editing.
Funding
The author(s) declare financial support was received for the research, authorship, and/or publication of this article. Funding source of this study: Science and Technology Department of Shanxi Province: Shanxi Province Science and Technology cooperation and exchange special project “Preparation of chitosan composite nano-silver latex ureteral stent tube and animal experimental study” project number: 202204041101047.
Conflict of interest
The authors declare that the research was conducted in the absence of any commercial or financial relationships that could be construed as a potential conflict of interest.
Publisher’s note
All claims expressed in this article are solely those of the authors and do not necessarily represent those of their affiliated organizations, or those of the publisher, the editors and the reviewers. Any product that may be evaluated in this article, or claim that may be made by its manufacturer, is not guaranteed or endorsed by the publisher.
References
Andleeb, S., Iqbal, Z., Gulzar, N., Raza, A., and Ahmad, A. (2023). Synthesis, characterization, acute dermal toxicity, anti-inflammatory, and wound healing potential of biogenic silver nanoparticles in balb C mice. Curr. Pharm. Biotechnol. 24. doi:10.2174/1389201024666230727122201
Azizi, A., Ghasemirad, M., Mortezagholi, B., Movahed, E., Aryanezhad, S. S., Makiya, A., et al. (2023). Study of cytotoxic and antibacterial activity of Ag- and mg-dual-doped ZnO nanoparticles. ChemistryOpen 13, e202300093. doi:10.1002/open.202300093
Bahar, K., Nahideh, A., Morteza, M., Soodabeh, D., Abadi, A., Abasi, E., et al. (2017). A review on potential role of silver nanoparticles and possible mechanisms of their actions on bacteria. Drug Res. (Stuttg) 67, 70–76. doi:10.1055/s-0042-113383
Bjarnsholt, T., Buhlin, K., Dufrêne, Y. F., Gomelsky, M., Moroni, A., Ramstedt, M., et al. (2018). Biofilm formation - what we can learn from recent developments. J. Intern Med. 284, 332–345. doi:10.1111/joim.12782
Blair, J. M., Webber, M. A., Baylay, A. J., Ogbolu, D. O., and Piddock, L. J. V. (2015). Molecular mechanisms of antibiotic resistance. Nat. Rev. Microbiol. 13 (1), 42–51. doi:10.1038/nrmicro3380
Cai, X., Xie, Z., Li, D., Kassymova, M., Zang, S. Q., and Jiang, H. L. (2020). Nano-sized metal-organic frameworks: synthesis and applications. Coord. Chem. Rev. 417, 213366. doi:10.1016/j.ccr.2020.213366
Dachi, F. N. C. (2022). Orthopaedic implant related infection. Int. J. Res. Publ. 93 (2708-3578). doi:10.47119/ijrp100931120222751
Fairweather, J., Findlay, M., and Isles, C. (2020). Catheter related blood stream infection. Berlin, Germany: Springer eBooks.
Friedman, A., Blecher, K., Sanchez, D., Tuckman-Vernon, C., Gialanella, P., Friedman, J. M., et al. (2011). Susceptibility of Gram-positive and -negative bacteria to novel nitric oxide-releasing nanoparticle technology. Virulence 2 (3), 217–221. doi:10.4161/viru.2.3.16161
Guo, M., Zhang, W., Niu, S., et al. (2023). Nrf2 adaptively modulates the oxidative stress-related hepatocyte damage induced by nano silver. Zhuhai, Guangdong, China: The 10th National Toxicology Congress of Chinese Society of Toxicology, 1. (in Chinese).
Guo, Qi, Guo, Z., Wu, W., et al. (2019). Research progress of FtsZ inhibitors as novel antibacterial molecules. Guangzhou Med. 54 (02), 9–17. (in Chinese). doi:10.3969/j.issn.1000-8535.2023.02.002
Huang, kunkun (2023). Correlative study on the identification of pathogenic bacteria in implant infection after fracture surgery. Adv. Clin. Med. 13 (6), 10226–10331. doi:10.12677/acm.2023.1361431
Jia, L., Wei, L., Zhang, Y., and Wang, H. (2022). Preparation and properties of chitosan-VC loaded nano-silver-doped natural latex. Prog. Nat. Sci. Mater. Int. 32 (1002-0071), 625–633. doi:10.1016/j.pnsc.2022.09.007
Kai, Z. X. (2022). Preparation of carboxymethyl chitosan silver nanoscale antibacterial latex and its antibacterial activity in vitro. Jinzhong, Shanxi, China: Shanxi Medical University. (in Chinese).
Kotchaplai, P., Khan, E., and Vangnai, A. S. (2017). Membrane alterations in Pseudomonas putida F1 exposed to nanoscale zerovalent iron: effects of short-term and repetitive nZVI exposure. Environ. Sci. Technol. 51 (14), 7804–7813. doi:10.1021/acs.est.7b00736
Kurup, M., Kumar, M., Ramanathan, S., and Chandra, M. (2023). The biogenetic synthesis of metallic nanoparticles and the role they play in anti-inflammatory drug treatment. Curr. Drug Discov. Technol. 20. doi:10.2174/1570163820666230718123544
Li, W. R., Sun, T. L., Zhou, S. L., Ma, Y. K., Shi, Q. S., Xie, X. B., et al. (2017). A comparative analysis of antibacterial activity, dynamics, and effects of silver ions and silver nanoparticles against four bacterial strains. Int. Biodeterior. Biodegrad. 123, 304–310. doi:10.1016/j.ibiod.2017.07.015
Liu, Z., Xu, Y., Zhu, R., et al. (2023a). Research progress on preparation and application of nano-silver. J. Tianjin Univ. Sci. Technol. 38 (02), 75–80. (in Chinese). doi:10.13364/j.issn.1672-6510.20220158
Liu, C., Ling, J., Yang, L. Y., Ouyang, X. k., and Wang, N. (2023b). Chitosan-based carbon nitride-polydopamine-silver composite dressing with antibacterial properties for wound healing. Carbohydr. Polym. 303, 120436. doi:10.1016/j.carbpol.2022.120436
Lu, X., Ma, Y., Lu, Y., et al. (2017). Clinical study of natural latex nano silver pillow for head, face and neck burn patients. PLA Nurs. J. 34 (24), 59–62. (in Chinese). doi:10.3969/j.issn.1008-9993.2017.24.014
Maomao, X., Meng, G., Yang, Y., Martin, M., Rotello, V. M., Zboril, R., et al. (2023). Antibacterial nanomaterials: mechanisms, impacts on antimicrobial resistance and design principles. A J. Ger. Chem. Soc. 62, e202217345. doi:10.1002/anie.202217345
Ma, Y., and Hou, J. (2022). Preparation and antibacterial activity of nano copper oxide- loaded zeolite 10X. Int. J. Mol. Sci. 23 (1422-0067), 8421. doi:10.3390/ijms23158421
Mueez, A., Hussain, S., Ahmad, M., Raza, A., Ahmed, I., and Amjad, M. (2022). Green synthesis of nanosilver particles from plants extract. Int. J. Agric. Environ. bioresearch 07 (2456-8643), 96–122. doi:10.35410/ijaeb.2022.5703
O’Cearbhaill, E. D. (2019). Shedding light on implant-associated infection. Sci. Transl. Med. 11, 1946–6234. doi:10.1126/scitranslmed.aaz3709
Peng, X. (2020). Antibacterial effect of silver-titanium bimetallic nano-materials. Integr. Ferroelectr. 209 (1026-7530), 150–161. doi:10.1080/10584587.2020.1728825
Prabhu, A., Baliga, V., Bhat, S., Thenkondar, S. T., Nayak, Y., et al. (2023). Transforming wound management: nanomaterials and their clinical impact. Pharmaceutics 15 (5), 1560. doi:10.3390/pharmaceutics15051560
Radzig, M. A., Nadtochenko, V. A., Koksharova, O. A., Kiwi, J., Lipasova, V., and Khmel, I. (2013). Antibacterial effects of silver nanoparticles on gram-negative bacteria: influence on the growth and biofilms formation, mechanisms of action. Colloids Surf. B Biointerfaces 102, 300–306. doi:10.1016/j.colsurfb.2012.07.039
Rather, G. A., Hassan, S., Pal, S., et al. (2021). Antimicrobial efficacy of biogenic silver and zinc nanocrystals/nanoparticles to combat the drug resistance in human pathogens, 0. London, UK: IntechOpen eBooks, 0.
Rizzello, L., and Pompa, P. P. (2014). Nanosilver-based antibacterial drugs and devices: mechanisms, methodological drawbacks, and guidelines. Chem. Soc. Rev. 43 (5), 1501–1518. doi:10.1039/c3cs60218d
Sanyasi, S., Majhi, R. K., Kumar, S., Mishra, M., Ghosh, A., Suar, M., et al. (2016). Polysaccharide-capped silver Nanoparticles inhibit biofilm formation and eliminate multi-drug-resistant bacteria by disrupting bacterial cytoskeleton with reduced cytotoxicity towards mammalian cells. Sci. Rep. 6, 24929. doi:10.1038/srep24929
Stabryla, L. M., Johnston, K. A., Diemler, N. A., Cooper, V. S., Millstone, J. E., Haig, S. J., et al. (2021). Role of bacterial motility in differential resistance mechanisms of silver nanoparticles and silver ions. Nat. Nanotechnol. 16 (9), 996–1003. doi:10.1038/s41565-021-00929-w
Tan, J., Liu, Z., Wang, D., Zhang, X., Qian, S., and Liu, X. (2020). A facile and universal strategy to endow implant materials with antibacterial ability via alkalinity disturbing bacterial respiration. Biomater. Sci. 8 (7), 1815–1829. doi:10.1039/c9bm01793c
Wu, J. K., Zhan, M. J., Chang, Y., Gao, H., Ye, J., Yu, R., et al. (2019). Mechanistic insights into stress response and metabolic activity resilience of Nitrosomonas europaea cultures to long-term CeO2 nanoparticle exposure. Environ. Science-Nano 6 (7), 2215–2227. doi:10.1039/c9en00346k
Wu, Q., Miao, W. S., Zhang, Y. D., Gao, H. j., and Hui, D. (2020). Mechanical properties of nanomaterials: a review. Nanotechnol. Rev. 9 (1), 259–273. doi:10.1515/ntrev-2020-0021
Xiang, D., and Zheng, C. (2009). Research progress of antiviral effect of nano-silver. J. Dalian Med. Univ. 31 (06), 716–719. (in Chinese).
Xu, B. (2021). Study on biosafety of chitosan composite nano-silver latex ureteral stent tube. Jinzhong, China: Shanxi Medical University. (in Chinese).
Xu, B. (2022). Study on biosafety of chitosan composite nano-silver latex ureteral stent tube. Jinzhong, China: Shanxi Medical University. (in Chinese).
Xu, B., Wang, Z., and Chun, L. (2019). Research progress of urinary tract infections associated with ureteral stents and antimicrobial biomaterials. J. Clin. Urology 36 (05), 406–411. (in Chinese). doi:10.13201/j.issn.1001-1420.2021.05.016
Yan, X.-A., Mchirume, W., Yi-xiang, L., et al. (2023). Research progress on novel treatment of bacterial biofilm infection. West China Med. 38 (08), 1276–1280. (in Chinese).
Yang, Y., Wu, S., and Zou, Y. (2003). Research progress of implant infection. Chongqing Med. 32 (7), 926–928. (in Chinese). doi:10.3969/j.issn.1671-8348.2003.07.068
Yang, K., Huang, M., He, X., An, Z., and Qin, H. (2022). Synergistic antibacterial effect of zinc oxide nanoparticles and polymorphonuclear neutrophils. J. Funct. Biomaterials 13 (2079-4983), 35. doi:10.3390/jfb13020035
Yao, X., Tang, X., Wang, X., et al. (2019). Research progress on antibacterial mechanism of inorganic antibacterial materials. Mater. Rev. 35 (01), 1105–1111. (in Chinese). doi:10.11896/cldb.19090190
Yn, S., Uo, H., Häfeli, U. O., and Bach, H. (2017). Metal nanoparticles: understanding the mechanisms behind antibacterial activity. J. Nanobiotechnology 15, 65. doi:10.1186/s12951-017-0308-z
Zhai, J., Guo, Y., Chun, L., et al. (2011). Evaluation of cytotoxicity of nano-Ag-sio2 catheters. Chin. J. Tissue Eng. Res. Clin. Rehabilitation 15 (29), 5371–5374. (in Chinese). doi:10.3969/j.issn.1673-8225.2011.29.013
Zhang, J. (2023). Clinical observation of nano-silver antibacterial gel promoting wound healing after anal fistula operation. Clin. Pract. Integr. Chin. West. Med. 20 (03), 84–85. (in Chinese).
Zhang, G., Chen, J., and Li, W. (2022). Conjugative antibiotic-resistant plasmids promote bacterial colonization of microplastics in water environments. J. Hazard Mater 430, 128443. doi:10.1016/j.jhazmat.2022.128443
Zhang, H., Chen, Y., Zhang, Y., Qiao, L., Chi, X., et al. (2023). Identification of anti-Mycobacterium tuberculosis agents targeting the interaction of bacterial division proteins FtsZ and SepFe. Acta Pharm. Sin. B 13 (05), 2056–2070. doi:10.1016/j.apsb.2023.01.022
Zha, Y., Li, Z., Zhong, Z., Ruan, Y., Sun, L., Zuo, F., et al. (2022). Size-dependent enhancement on conjugative transfer of antibiotic resistance genes by micro/nanoplastics. J. Hazard Mater 431, 128561. doi:10.1016/j.jhazmat.2022.128561
Zhou, Y., Zhang, C., Zou, H., et al. (2019). Efficacy and safety analysis of nano-silver composite activated carbon fiber sterile hemostatic dressing for promoting burn wound healing. Chin. Med. 15 (10), 1633–1637. (in Chinese). doi:10.3760/j.issn.1673-4777.2020.10.034
Zhu, B., Xia, X., Xia, N., Zhang, S., and Guo, X. (2014). Modification of Fatty acids in membranes of bacteria: implication for an adaptive mechanism to the toxicity of carbon nanotubes. Environ. Sci. Technol. 48 (7), 4086–4095. doi:10.1021/es404359v
Zixiang, K., Chun, L., Liu, Y., et al. (2019a). Preparation of novel antibacterial latex and its antibacterial effect on Common pathogens of urinary system. J. Biomed. Eng. Clin. Sci. 25 (1), 1–5. (in Chinese). doi:10.13339/j.cnki.sglc.20201221.023
Zixiang, K., Chun, L., Liu, Y., et al. (2019b). Preparation and bacteriostatic activity of Carboxymethyl chitosan composite Silver and copper oxide nanoparticles. J. Biomed. Eng. 40 (01), 38–42. (in Chinese). doi:10.19529/j.cnki.1672-6278.2021.01.07
Keywords: nanosilver, natural latex, oxidative stress, antibacterial mechanism, cell membrane destruction
Citation: Meng Z, Fan B, Yan P and Liu C (2024) Research on the antibacterial mechanism of medical nanosilver latex composite materials. Front. Mater. 11:1349870. doi: 10.3389/fmats.2024.1349870
Received: 05 December 2023; Accepted: 05 January 2024;
Published: 24 January 2024.
Edited by:
Joanna Rydz, Polish Academy of Sciences, PolandReviewed by:
Marimuthu Karunakaran, Alagappa Government Arts College, IndiaAwadh Bihari Yadav, Allahabad University, India
Copyright © 2024 Meng, Fan, Yan and Liu. This is an open-access article distributed under the terms of the Creative Commons Attribution License (CC BY). The use, distribution or reproduction in other forums is permitted, provided the original author(s) and the copyright owner(s) are credited and that the original publication in this journal is cited, in accordance with accepted academic practice. No use, distribution or reproduction is permitted which does not comply with these terms.
*Correspondence: Chun Liu, c3h0eWxpdWNodW5AMTI2LmNvbQ==