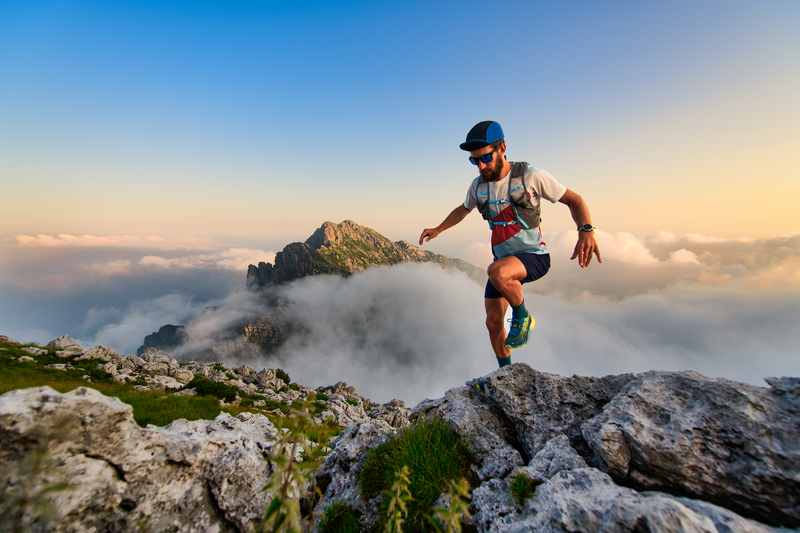
94% of researchers rate our articles as excellent or good
Learn more about the work of our research integrity team to safeguard the quality of each article we publish.
Find out more
REVIEW article
Front. Mater. , 16 February 2024
Sec. Biomaterials and Bio-Inspired Materials
Volume 11 - 2024 | https://doi.org/10.3389/fmats.2024.1296399
Natural polymers have many uses, and Tragacanth gum is just one of them. Many people are interested in natural gums because of their many attractive characteristics, such as being ‘green’ bio-based renewable materials, being easily accessible, inexpensive, and structurally diverse. One class of naturally occurring polysaccharides is called gum because of its tendency to create a gel or a thick solution. Among the many plant-based raw materials, these polysaccharide gums are abundant. Hydrogels, which are three-dimensional polymeric webs that can imitate live tissues, have demonstrated remarkable potential as adjustable biomaterials in numerous regenerative techniques due to their high water or biological exudate absorption capacities. Natural polysaccharides, often known as gums, are present in many different types of trees and possess many desirable properties, such as being renewable, biocompatible, biodegradable, non-toxic, and amenable to chemical modification. Many people are curious about certain parts of the food, water, energy, biotech, environmental, and healthcare sectors as of now. Gum, a type of very important and unique food ingredient, has many vital uses in the food business. Cosmetics, coating, photosensitive resin, fertilizer, casting, pharmaceuticals, and tobacco are just a few of the non-food businesses that make use of their strong water-affinity and structural plasticity. There are a lot of benefits to hydrogels made from natural gums as opposed to those made from synthetic sources. Synthesis hydrogel polymers have been the center of interest among these non-food applications because of their extensive use in the pharmaceutical and medical fields. The Tragacanth gum hydrogels used for medication delivery and tissue engineering have been the focus of this study. We also paid close attention to drug delivery, physical-chemical properties, and the extraction of Tragacanth gum. Our research has a wide range of biomedical applications, including tissue engineering for bone, skin, fixation of bone, periodontal, and cartilage. Possible futures based on hydrogels made of Tragacanth gum were likewise our primary focus.
Food gum, a form of very significant and distinctive food additive, serves several crucial purposes in the food industry. Due to their excellent structural adaptability and high water affinity are also used in non-food industries such as cosmetics, coating, photosensitive resin, fertilizer, casting, tobacco, and pharmaceuticals. Synthesis hydrogel polymers have drawn the most attention among these non-food uses because of their ubiquitous use in the pharmaceutical and medical fields, such as delivery systems for medications, cells, genes, or proteins, and as scaffolds for tissue engineering (Xie et al., 2017). The ophrastus first wrote about Tragacanth gum, an ancient exudate gum, in the third century BC. The Greek terms “tragos” and “akantha,” which mean “goat” and “horn,” respectively, are the origin of the word “Tragacanth.” Tragacanth gum may be known by this term due to its readily available, curled ribbon-like shape. Exudate gum from Astragalus gummifer or other species is known as Tragacanth gum (Verbeken et al., 2003; Barak et al., 2020). Food gum, a type of crucial food additive, is used extensively in the frozen food, dairy products, beverages, condiments, cakes, starch, candy, wine, food, and frozen food industries (Nishinari and Zhang, 2004; Funami, 2011). It has many important functions on food applications, including thickening, gelation, emulsification, suspension, stabilisation, and clarification Hydrogels are three-dimensional polymeric webs with high water or biological exudate absorption capacities that can resemble live tissues; they have so far showed significant promise as tuneable biomaterials in a range of regenerative techniques. Natural polysaccharides, or gums, are amazing substances that are renewable, biocompatible, biodegradable, non-toxic, and readily chemically changeable. They are present in a variety of tree genera. Recently, there has been a lot of interest in topics related to the food, water, energy, biotechnology, environment, and medical industries. In the food industry, food gum, a type of very important and distinctive food additive, provides a number of essential functions (Dickinson, 2003; Sawai et al., 2008). Tragacanth gum has recently shown significant potential as a therapeutic ingredient in tissue engineering and regenerative medicine, which is in line with the growing interest of researchers in the use of naturally occurring materials in biomedicine. Tragacanth gum may be readily isolated from the stems and branches of many astragalus plants because it is a polysaccharide. This anionic polymer is a well-known biodegradable, non-toxic, non-carcinogenic substance. Tragacanth gum is a desirable material not just in industrial settings (such as food packaging) but also in biological techniques (such as medication administration) due to its resilience against microbial, heat, and acid degradation. In the area of green chemistry, Tragacanth gum has been demonstrated to be a beneficial reagent in the synthesis and stabilisation of metal nanoparticles over time (TaghavizadehYazdi et al., 2021a). Because of chemical or physical crosslinking, hydrogels are polymeric materials with a three-dimensional network structure that have the capacity to absorb large amounts of water while maintaining their structural integrity (Wang et al., 2008). The widespread applications of polysaccharide-based materials in the pharmaceutical industry are facilitated by their favourable characteristics, such as large structural plasticity, high water-affinity, and simple methods (Yu et al., 2016). One of the natural polymers with a wide range of applications is Tragacanth gum. Natural gums have attracted a lot of interest because of their availability, affordability, structural variation, and excellent capabilities as “green” bio-based renewable materials. The term “gum” refers to a class of naturally occurring polysaccharides that can either form gel or a viscous fluid. These polysaccharide gums are one of the several basic materials obtained from flora. Due to their biodegradability, biocompatibility, high water content, and cellular integrations, polysaccharide-based hydrogels are extremely promising in tissue engineering and biomedical applications because they provide a favourable milieu for cell differentiation and proliferation (Diolosà et al., 2014; Xie et al., 2017).
Tragacanth gum is a natural hydrophilic polysaccharide that is nonmutagenic, nonallergenic, noncarcinogenic, nonteratogenic, and non-toxic. Afavorable environment for cell development. It is a complex polysaccharide that contains galacturonic acid, xylose, arabinose, galactose, and fucose and is highly branched, heterogeneous, and complicated. Ragacanth gum. Tragacanth gum molecules have primary and secondary hydroxyl and carboxylic acid groups, which offer locations for reactions with monomers and crosslinking agents (Größl et al., 2005). For instance, it has been reported that a Tragacanth gum-based sterile hydrogel was generated using radiation, and the hydrogel polymer might be employed for both medication administration and wound dressing. The outcomes demonstrated that the hydrogel swelling was impacted by the hydrogel polymer’s (Kiani et al., 2012). These produced hydrogel wound dressings were discovered to be blood compatible and non-thrombogenic in nature after absorbing simulated wound fluid. Additionally, for 24 h without any abrupt releases, these hydrogels contained and released the model drug moxifloxacin in a regulated manner (Xie et al., 2017). Due to their controlled medication release, high swelling debridement properties, and capacity to maintain an ideal wound healing environment to speed up the healing process, these hydrogels can be considered an acceptable material for wound dressing application (Singh et al., 2016). According to recent reports, Tragacanth gum has the ability to naturally speed up the healing of wounds (Moghbel et al., 2005). Tragacanth is also efficient during the periods of wound remodelling and proliferation. Its antioxidant action aids in the healing process for wounds. Antibacterial nanofiber scaffolds based on Tragacanth gum and polyvinyl alcohol (PVA) have been employed for wound dressing applications (Ranjbar-Mohammadi et al., 2013). There have also been reports of human fibroblast cells adhering to nanofibers and proliferating over them. The naturally occurring hetero-polysaccharide known as Tragacanth gum comes from the Astragalus gummifer (Singh et al., 2016; Singh et al., 2017a). The exudate from the Astragalus gummifer bark contains the naturally occurring polymer known as Tragacanthgum. The exudate from the Astragalus gummifer bark contains the naturally occurring polymer known as Tragacanth gum (Tischer et al., 2002) additionally, it includes There are two important fractions: i) a small amount of water-soluble Tragacanthin, and ii) swellableBassorin. Tragacanth gum has It has a backbone of (1,4)-D-galacturonic acid and demonstrates non-mutagenic, non-carcinogenic, non-toxic, and non-teratogenic qualities in addition to being biocompatible and environmentally friendly. It also contains -COOH functional groups to boost pH sensitivity (Aspinall and Baillie, 1963; Alam et al., 2014). As It has a wide range of applications, including emulsifier, thickening, stabiliser, moisture-retention, binding, freezing, gelling, and adhesive in the cosmetic, leather, pharmaceutical, textile, and food industries (Nejatian et al., 2020a; Nagaraja et al., 2021). Due to their possible temperature-responsive behaviours, such as a lowest critical solution temperature (LCST) of 32°C, poly(N-isopropyl acrylamide, or PNIPA) and its copolymer-based polymeric networks are widely accepted for use in drug delivery applications (Mallikarjuna Reddy et al., 2008). Additionally, it creates intramolecular hydrogen bonds between water molecules and amide groups and is water soluble and biocompatible. Although PNIPA generates hydrogen bonds below the LCST, the hydrogen bands collapse above the LCST, causing the formation of networks and hydrophobic chains (Jalababu et al., 2018). Temperature-sensitive hydrogels have certain features that have been widely exploited in biotechnological and medicinal applications, such as drug delivery systems, including reversible swelling and a swelling nature in water (Nagaraja et al., 2021). 5-Fluorouracil (5FU) is a pyrimidine analog with anti-neoplastic and anti-metabolic characteristics that has been extensively used to treat colon cancer and other solid tumorsIt interferes with DNA by obstructing thymidylate synthetase action and has a short biological half-life i.e., 10–20 min (Kaiser et al., 2003). Tragacanth gum is a well-known natural compound with many uses that comes from various Astragalus species. Tragacanth gum has a long history of success in the food and pharmaceutical industries, and it has gradually been discovered to be a helpful material in other fields as well. Tissue engineering and regenerative medicine, as well as waste management, environmentally friendly nanoparticle manufacturing, medication delivery techniques, and waste management (Zare et al., 2019). With regard to tissue engineering techniques for both hard (such as the bone) and soft (such as the skin), Tragacanth gum has recently gained a lot of interest (Heydary et al., 2015). Despite the fact that there have not been many trials on Tragacanth gum -based therapies for tissue restoration There is strong evidence to indicate the applicability of Tragacanth gum based structures such as hydrogels and nanofibers) for quickening the healing process (TaghavizadehYazdi et al., 2021b). The commercial sources of this exudate, which is collected by tapping the branches or roots, include several species of the genus Astragalus, which grows naturally in Iran, Iraq, Turkey, Afghanistan, and neighbouring Russia. Ice cream, liquors, lotions, sizings, and other industrial products can benefit from its hydrophilic and colloidal qualities. According to commercial and national circumstances, Tragacanth gum plants have never been grown in any form in Iran; instead, they are solely harvested from the wild inhabitants. They were not given much thought as a sustaining asset until recently, but now there is a national legislation or regulation that forbids the removal of any Tragacanth gum plant. However, at least some of the species are still used by the tribal members for desperately needed fuel, and it has been claimed that entire stands of the bigger highland shrubs have been cleared in the past for this purpose. The gums are being used more frequently now than in previous years, despite price increases that have not increased supply. The preservation of existing stands and their continued use appear, therefore, to be key factors in the industry’s growth (Gentry, 1957). Gums are known to be pathological by-products produced by the disintegration of cell walls (extracellular production; gummosis) following plant injuries or as a result of unfavourable conditions (such as drought) (Amiri et al., 2014). One of the most prevalent natural raw ingredients is polysaccharide gum. They are used all over the world, from the food industry to healthcare systems, due to their availability, affordability, non-toxicity, and environmental friendliness in addition to the fact that they are renewable sources. Tragacanth gum, one of many well-known gums, is regarded as a flexible substance in biomedicine. Iran is the world’s top producer and exporter of Tragacanth gum, also known as Katira, and most of the natural gum used in Tragacanth gum comes from Central Asian and Eastern European nations (TaghavizadehYazdi et al., 2021a). In a variety of healthcare-related applications, including lotions used for external applications (hair and hand creams), GT has been discovered to be a helpful plant-derived chemical. GT is frequently used as an emulsifier in the food, pharmaceutical, and related industries because of its outstanding stability in a wide range of pH and temperatures. It also has an incredibly extended shelf life. As an consequence, the Food and Drug Administration (FDA) has designated GT as a substance that is generally recognised as safe (GRAS). GT is a highly attractive material for tissue engineering and regenerative medicine strategies since it degrades in living systems (Kaith et al., 2015). Therefore, GT can be used to create wound dressings in a number of experimental experiments (Singh et al., 2016). GT has been used in the reconstruction of hard tissues, either on their own or embedded in composites, in addition to applications for soft tissue healing (Kulanthaivel et al., 2017a). Tragacanth applications in a number of different sectors. In bone tissue engineering, bone implants are utilised as a therapy option to replace or re-establish a missing bone. The biological actions of this polysaccharide include anti-coagulant, antiviral, anti-inflammatory, anti-tumour, and anti-oxidant capabilities. The Tragacanth is well known for its antibacterial and wound-healing abilities. Vero cells seeded on to the scaffold confirmed its bioactivity and biocompatibility. Scaffold and the scaffold being submerged in a dummy bodily fluid, respectively. It also contains non-specific immunomodulatory, hypocholesterolemic, anti-inflammatory, and antiviral effects (Koyyada and Orsu, 2021).
These innocuous, biocompatible, water-soluble substances give solutions viscosity and rheological characteristics. Natural gums (hydrocolloids) are excellent choices for thickeners, emulsifiers, and gelling agents because of their water solubility. Plant exudation (Tragacanth), seed endosperm (locust bean and guar), and tree exudation (karaya, sterculia, and arabic gums) are the sources of plant-based gums. Hydrogels made from Tragacanth gum show strong biocompatibility and potential for cell development. Moxifloxacin antibiotic was able to release from Tragacanth gum-sodium alginate-polyvinyl alcohol hydrogels with a non-Fickian profile and without a rapid release. This may result in the medication’s initial release followed by a retention of its concentration (Hixson-Crowell model for drug release) (Mohammadinejad et al., 2019). An anionic polysaccharide called Tragacanth gum is a bio-based polymer made from renewable resources. As a biomaterial, TG has been applied in the biomedical sector as drug carriers and for wound healing, as well as in industrial applications including food packaging and water treatment. Despite significant advancements in TG use during the past 10 years, this fascinating area of study lacks a comprehensive overview. Tragacanth gum is a crucial component of agricultural chemicals. TG-prepared bio-based chemicals are now being created for industrial use. One of the most widely used biopolymers, this gum is employed as an emulsifier and thickening in food, bio-products, cosmetic, and pharmaceutical formulations to manage their texture and microstructure as well as to increase viscosity and stability (Zare et al., 2019). Given that the supply of fossil fuels will run out soon, we must find a replacement for it in order to meet our needs. Natural polymers have recently captured the interest of researchers. One of them is Tragacanth gum, an antiquated polysaccharide that has been utilised extensively in a variety of sectors since the time of Theophrastus. It is due to their characteristics, which include renewability, biocompatibility, biodegradability, stability at a wide pH range, nontoxicity, cost effectiveness, abundance, etc. Following a thorough analysis of numerous articles, it was determined that Tragacanth gum would be an appropriate alternative for petroleum-based components, resulting in a reduction in the price of oil refineries and associated operations (Mallakpour et al., 2022). Tragacanth gum can be utilised as the wall material in the encapsulation of various chemicals, particularly plant extracts, as it is a biocompatible and biodegradable polymer with good qualities like emulsifying, viscosity, and cross-linking ability. In this study, the microemulsion method was applied for the first time to create nanocapsules containing plant extract using Tragacanth gum. Through the use of an ultrasonic and magnetic stirrer, the impact of various parameters on the average size of nanocapsules formed in the presence of aluminium and calcium chloride was examined A complex made of polysaccharides with good coating properties is Tragacanth gum. However, it has relatively little value in the post-harvest preservation of fresh fruits and vegetables. In this study, the impact of Tragacanth gum (1%), on the post-harvest quality of apricot fruits during storage, was examined (Ghayempour et al., 2015). Our attention was also drawn to the extraction of Tragacanth gum, drug delivery, physical-chemical characteristics, and different biomedical applications, including bone tissue engineering, skin tissue engineering, bone tissue fixing, periodontal tissue engineering, and cartilage tissue engineering Figure 1.
Additionally, we focused on potential futures. based on hydrogels made from Tragacanth They are also employed in non-food industries like cosmetics, paint, photosensitive resin, fertiliser, casting, tobacco, and medicines due to their outstanding structural versatility and high water-affinity. Hydrogels derived from natural gums have a variety of advantages over those having a synthetic origin. Synthesis hydrogel polymers have drawn the greatest attention among these non-food uses because of their extensive use in the pharmaceutical and medical areas. The hydrogels generated from Tragacanth gum used for tissue engineering and medication delivery are the focus of this article.
Gums are a group of polysaccharides that are produced naturally by certain botanical (trees and shrubs, seeds, and tubers), algal (red and brown seaweeds), or microbial sources (Amara, 2022). Some trees and shrubs excrete gums as sticky secretions in response to mechanical injuries or biological attacks that usually harden to seal the wound in order to give protection (Nussinovitch, 2009). They mostly disperse easily in water, depending on the water availability and their chemical structure, and could produce a colloidal solution, dispersion, viscose suspension, or gel (Valencia et al., 2019). They have been used for centuries in many different ways (e.g., foods, pharmaceuticals, cosmetics, textiles, agro-chemicals, and lithography) as thickener, gelling agent (Saha and Bhattacharya, 2010), moisture-controlling agent (Kohajdová and Karovičová, 2009), stabilizer, emulsifier (Garti and Reichman, 1993; Dickinson, 2009), dietary fiber (Chawla and Patil, 2010; Dhingra et al., 2012), crystallization inhibitor (Maity et al., 2018), fat replacer (Peng and Yao, 2017), and even as film-forming agents (Nieto, 2009; Nejatian et al., 2020a).
Polysaccharide gums are among the most abundant raw materials in nature. Besides being a renewable source, they are easily accessible, relatively affordable, non-toxic, and environmentally friendly, thereby offering worldwide usage that ranges from the food industry to healthcare systems. Among different well-characterized gums, Tragacanth gum is recognized as a versatile material that finds applications in different industries (Boamah et al., 2023). Tragacanth gum, commonly known as ‘Katira’ or ‘Gond Katira,’ is primarily produced in Central Asian and Eastern countries, with Iran being the major producer and exporter of this natural gum. (Anderson and Grant, 1988; Amiri et al., 2014; Yazdi et al., 2021). It is a thick, gummy, flavourless, water-soluble polysaccharide concoction (Kulkarni and Shaw, 2016).
Astragalus genus (Leguminosae family, Papilionidiae subfamily, including over 2,000 species) is a small woody evergreen shrub with varying height (from 10 cm up to 1 m), spreading and growing in the semi-desert and mountainous regions of south-west Asia, including Pakistan, Iran, Turkey, Syria, and Greece (Imeson, 1992; Whistler, 1993). Over 70% of the highest-quality Tragacanth gum on the global market comes from Iran (FAO, 1995; Sharifi et al., 2023). The most known commercial species are A. gummifer, A. parrowianus, A. fluccosus, A. rahensis, A. gossypinus, A. microcephalus, and A. compactus. Owing to the compositional differences, there is a wide range of applications for various species (Nejatian et al., 2020a). Tragacanth gum is a dried exudate obtained from the stem of the bush-like plant “goat’s thorn”, an Astragalus species belonging to the family Fabaceae. Astragalus plants exudate sap to safeguard the wounds of the plant against tissue injury as a defense mechanism, and the dried product of sap is referred to as exudate ‘gum’. The injured plant part is covered by the exudates, which become hard into flakes at one time on exposure to air and sunlight (Kandar et al., 2021). For over 2000 years, Tragacanth gum has been used commercially with widespread application as an emulsifier and thickener in emulsion systems for food, cosmetic, and pharmaceutical applications (Whistler, 1993).
Previously, Astragalus gummiferwas considered the primary source of Tragacanth gum, whereas currently Astragalus microcephalus serves as the major source (Barak et al., 2020). Other Astragalus species that provide Tragacanth gum include: a) Astragalus gummiferLabill.; b) Astragalus verus Olivier; c) Astragalus microcephalusWilld.; d) Astragalus brachycalyxFisch. exBoiss.; e) Astragalus myriacanthusBoiss.; f) Astragalus echidna Bunge; and g) Astragalus kurdicusBoiss (Amiri et al., 2020). Tragacanth gum, a highly acid-resistant hydrocolloid, has been accepted since 1961 as GRAS at the level of 0.2%–1.3% (Anderson and Bridgeman, 1985). It has been used for many years as a stabilizer, thickener, emulsifier, and suspending agent in the food, pharmaceutical, cosmetic, textile, and leather industries as well as in technical applications based on its high viscosity at low concentration, good suspending action, unusually high stability to heat and acidity, and effective emulsifying properties. It is also pourable, has a creamy mouthfeel, good flavour-release properties (Weiping, 2000) and a very long shelf life (Levy and Schwarz, 1958). Tragacanth gum is used in the food industry in salad dressings, condiments, sauces, bakery emulsions, oil and flavor emulsions, fillings and toppings, confectionery, soft drinks, jellies, desserts, ice creams, flavors, and spices (Weiping, 2000).
Tragacanth gum is a very complex heterogeneous, highly branched, anionic polysaccharide of high molecular weight of 840–850 kDa (Yazdi et al., 2021). The polysaccharide is slightly acidic, bound with small proportions of protein (below 4%), and accompanied by trace amounts of starch and cellulosic material (Balaghi et al., 2010). The FTIR spectra of Tragacant gum reveal a prominent broad band around 3330 cm−1, attributed to the stretching vibrations of–OH groups associated with free, inter, and intra-molecular bound hydroxyl groups. Additionally, the band observed at 2940 cm−1 serves as an indicator of C–H stretching. The peaks at 1744 cm−1 can be assigned to carbonyl stretching vibrations, specifically the C=O stretching of–COOH. The intensity of the band within the 1623–1644 cm−1 range signifies the presence of protein in the structure. Furthermore, the bands at 1,245, 1,079, and 1024 cm−1 are linked to the stretching vibrations of C-O in polyols, ethers, and alcohol groups, respectively. The peaks within the 800–1,200 cm−1 range are indicative of the fingerprint region for carbohydrates (Kurt, 2018) (Figure 2).
FIGURE 2. FTIR spectra of Tragacant gum (Kurt, 2018).
Also, the protein content exhibits different values depending on the species; for example, A. fluccosus, A. microcephalus, and A. compactus may typically contain 1.65%–2.59% protein in their composition. In addition, the carbohydrate content of different species varied in the range of 83.81%–86.52%. Calcium, magnesium, and potassium are the associated cations (Anderson and Grant, 1988). Although there are variations in the mineral content of Tragacanth gum species, calcium and potassium are the main inorganic elements for all species. It consists of two main fractions: i) a water-insoluble component called bassorin, which has the capacity to swell and form a gel, and ii) a water-soluble component called Tragacanthin (Balaghi et al., 2010). Bassorin, a pectic component, has a chain of (1–4)-linked α- D-galacturonic acid units, some of which are substituted at O-3 with β-D-xylopyranosyl units, and some of these are terminated with D-Gal or L-Fuc (Phillips and Williams, 2009). Tragacanthin, the water component, has a molar mass of approximately 104 Da to form a colloidal hydrosol solution (Elias, 1992). The water-soluble Tragacanthin is reported as a neutral, highly branched arabinogalactan (of type II) comprising a (1–6) and (1–3)-linked core chain containing galactose and arabinose (both in furanose and pyranose forms) and side groups of (1–2), (1–3), and (1–5)-linked arabinose units occurring as monosccharide or oligosaccharides (Tischer et al., 2002). Depending on the species, the ratio of the water-swellable to the water-soluble fraction varies (Balaghi et al., 2011). The easy separation of Tragacanthin and bassorin suggests that the two polysaccharides exist as a physical mixture and are not chemically bonded (Lapasin and Pricl, 1999). It has been reported that gums Tragacanth from different species of Astragalus have different ratios of the two components, different chemical compositions, and varying physico-chemical properties; therefore, functionalities and applications for each species are expected to be different (Balaghi et al., 2010). It is therefore well known that the ratio between the water-soluble and water-insoluble components of Tragacanth gum varies significantly between gums obtained from different Astragalus species, but it is unclear to what extent the composition of the polysaccharides affects the ratio of Tragacanthin to bassorin. The structural and physical properties of plant gum exudates are characterised by configuration, conformation, molecular weight, monosaccharide composition, position of glycosidic linkages, rheological properties, arrangement of monosaccharide, and solubility (Ahmad et al., 2019).
As far as the compositional make-up of the Tragacanth gum polysaccharides is concerned, it has been amply reported that Tragacanth gum contains L-arabinose, D-galactose, D-glucose, D-xylose, L-fructose, L-rhamnose, and D-galacturonic acid after undergoing acid hydrolysis (Tischer et al., 2002; Balaghi et al., 2011). Researchers evidently reported that the understanding up until now that the soluble fraction Tragacanthin consists of arabinogalactan and that the insoluble fraction i.e., bassorin is made up of “Tragacanthic acid” is probably not correct. Rather, at least the water soluble fraction (Tragacanthin) appears to resemble pectin, and seems to contain linear chains of galacturonic acid (probably 1,4-α-linked); hence, Tragacanth gum species rich in xylose with minor levels of fucose may contain xylogalacturonans and some fucoxylogalacturonans as the main components of the soluble fraction, whereas those having high fucose levels may mainly contain fuco-xylo galacturonan in the Tragacanthin part. In most Astragalus species, the bassorin and Tragacanthin have also been found to contain methyl groups, probably representing methoxylated galacturonic acid. The insoluble bassorin part generally appears to have less methoxyl substitutions than the soluble Tragacanth in part (Anderson and Grant, 1988). Also, it has been demonstrated that different Tragacanth gum samples obtained from different species of Astragalus have different composition, and produce different levels of soluble and insoluble gum fractions. Galacturonic acid was high in the soluble part of all species whereas L-fucose and partially xylose was major in insoluble fraction (Gavlighi et al., 2013a). Based on the previous studies, physico-chemical properties and compositional variations of Tragacanth gum depend on its sources, i.e., different types of Astragalus species Table 1 (Balaghi et al., 2011). The chemical composition of the commercial Tragacanth gum obtained from different species shows significant differences, which are directly resulted from seasonal and geographical variations. Actually, commercial TGs have large differences in chemical composition, including sugar composition, methoxy content, and the relative proportion of soluble and insoluble components (Gavlighi et al., 2013b).
Experimental research showed that Tragacanth gum could be notably efficient as a viscosity enhancer and stabiliser in acidic solutions (Singh, 2001). Its moisture content for different species is in the range of 8.79–12.94 g/100 g of product and generates highly viscous solutions when dispersed in water. The gum is produced by a process named ‘gummosis’ in response to mechanical or biological injuries. If the stem or root is wounded, the soft gum is forcibly secreted and easily dried on the trunk (Gentry, 1957). Based on structure, there are two general types of Tragacanth gum: i) ribbon (the best grades) and ii) flake (or harmony). Upon collection, Iranian Tragacanth ribbons are divided into five grades, while flakes are sorted in seven different grades (Verbeken et al., 2003; Asadian et al., 2009). With regard to the mechanical incisions, three methods namely,: 1) vertical, 2) horizontal and 3) diagonal incision is usually used and the latter is the most common one. The horizontal and diagonal incisions usually result in ribbon form gum exudation while the flake-shaped (highly look like to granules) one is formed via vertical incision (Gadziński et al., 2023). Apart from the importance of incision on the shape and quality of exudates, it is also believed that Astragalus sub-species influence the shape and quality of the exudates too. The gum collection is usually carried out in the dry and hot summer days (July–September) and 3–5 days after mechanical incisions (Asadian et al., 2009). Afterwards, it needs to be cleaned (to remove bark, sand, rubbles), sorted (colour, ribbon or flake forms) and packed as raw gum or milled as whole gum powder. In some cases, the water soluble and insoluble fractions are separated by mechanical or solvent fractionation. The ribbons and flakes usually show different physical properties, with ribbon form being the superior quality. For instance, pulverising of ribbons tends to light yellow powder, whereas the powder form of the flakes is creamy to tan. Moreover, ribbons create higher viscosity than flake forms (Verbeken et al., 2003), while the surface activity of flake is usually better than ribbons. The ribbon type is the most commercially available form of Tragacanth gum.
pH-sensitive gels derived from tragacanth were synthesized through crosslinking with glutaraldehyde. The formation of fresh acetal bonds and concurrent demethoxylation during the glutaraldehyde reaction led to the creation of new free carboxylate groups, contributing to the development of less densely packed biopolymeric structures (Bachra et al., 2021). The swelling behavior of tragacanth-based hydrogels exhibits a clear dependence on pH. In the pH range of 3.0–11, an increase in pH correlates with a higher swelling index. This phenomenon is attributed to the protonation of chains at lower pH, resulting in tight packing through hydrogen bonding (Bartil. et al., 2007). As pH increases, the ionization of carboxylic acid groups induces repulsion forces between carboxylate anions, leading to an expanded hydration shell and enhanced swelling. Understanding the mechanisms governing the pH-responsive behavior of tragacanth-based hydrogels is crucial for optimizing their performance. The intricate interplay between protonation, hydrogen bonding, and ionization of carboxylic acid groups defines the swelling response, and elucidating these mechanisms paves the way for fine-tuning hydrogel properties (Gajra et al., 2014). The pH-responsive nature of hydrogels derived from tragacanth gel opens up diverse applications. In drug delivery systems, the controlled release of therapeutic agents can be achieved by exploiting the pH-dependent swelling behavior (Xia et al., 2023). Additionally, these hydrogels find applications in sensors, responsive coatings, and environmental remediation, where pH changes can be leveraged for specific functionalities.
Common methods for hydrogel formation using Tragacanth gum include physical crosslinking and chemical crosslinking techniques. Physical crosslinking methods involve the formation of physical entanglements or interactions between polymer chains, such as temperature-induced gelation, ionotropic gelation, or freeze-thaw cycles. These methods offer simplicity and versatility in hydrogel fabrication (Bashir et al., 2020). Chemical crosslinking, on the other hand, involves the introduction of covalent bonds between polymer chains using crosslinking agents or chemical reactions. This method provides greater control over the hydrogel properties but requires careful selection of crosslinking agents and reaction conditions (Tessarolli et al., 2019). The choice of hydrogel formation method depends on factors such as desired mechanical strength, gelation kinetics, and the nature of the therapeutic agents to be encapsulated. Several factors influence the gelation process of Tragacanth gum-based hydrogels. These include polymer concentration, pH, temperature, presence of ions, and the addition of crosslinking agents. The polymer concentration affects the density of polymer chains, influencing the gelation kinetics and resulting gel properties. pH and temperature can alter the polymer chain conformation and promote gelation (Nasution et al., 2022). The presence of ions, such as calcium ions, can facilitate ionotropic gelation by interacting with charged groups on the polymer chains. Crosslinking agents, such as glutaraldehyde or genipin, can introduce covalent crosslinks between polymer chains, enhancing the mechanical stability of the hydrogels (Stojkov et al., 2021). Understanding and optimizing these factors are crucial for controlling the gelation process and obtaining hydrogels with desired properties. To characterize Tragacanth gum-based hydrogels, various physical and chemical characterization techniques are employed. Physical characterization techniques include rheology, which measures the mechanical properties and viscoelastic behavior of the hydrogels, and swelling studies to assess water absorption capacity and hydrogel stability (Joshi et al., 2022). Morphological analysis can be performed using scanning electron microscopy (SEM) or atomic force microscopy (AFM) to visualize the microstructure and surface topography of the hydrogels (Gieroba et al., 2023). Chemical characterization involves techniques such as Fourier-transform infrared spectroscopy (FTIR) and nuclear magnetic resonance (NMR) spectroscopy to analyze the chemical composition and confirm the presence of crosslinking. These characterization techniques provide valuable insights into the structure, mechanical properties, and chemical composition of Tragacanth gum-based hydrogels, aiding in their optimization and performance evaluation (Chahardoli et al., 2022).
Tragacanth gum-based hydrogels hold significant potential for drug discovery applications. These hydrogels can serve as versatile platforms for the encapsulation and controlled release of various therapeutic agents, including small molecules, proteins, peptides, and nucleic acids (TaghavizadehYazdi et al., 2021a). The unique properties of Tragacanth gum-based hydrogels, such as their high water absorption capacity, biocompatibility, and tunable gelation kinetics, make them well-suited for drug delivery applications (TaghavizadehYazdi et al., 2021b) They can protect sensitive drugs from degradation, provide sustained release profiles, and enable localized delivery to specific target sites (Gao et al., 2016). Moreover, the incorporation of bioactive molecules or nanoparticles within the hydrogel matrix can further enhance drug loading efficiency and therapeutic efficacy (Alqahtani et al., 2021). The development and optimization of Tragacanth gum-based hydrogels for drug discovery applications offer exciting prospects for advancing pharmaceutical research and improving patient outcomes Figure 3.
Oral drug delivery is a widely utilized route for pharmaceutical administration, but it poses several challenges, including poor drug solubility, enzymatic degradation, low bioavailability, and inadequate intestinal permeability (Potaś et al., 2020). Tragacanth gum-based hydrogels offer promising benefits as oral drug carriers (Shaikh et al., 2011). These hydrogels possess excellent mucoadhesive properties, which enable prolonged contact with the gastrointestinal mucosa and enhance drug absorption (Li and Mooney, 2016). Moreover, their high water absorption capacity allows for efficient drug encapsulation, protection from enzymatic degradation, and controlled release (Li and Mooney, 2016).
The mechanisms of drug release from Tragacanth gum-based hydrogels involve diffusion, swelling, erosion, and dissolution. The hydrogel matrix gradually swells in the presence of gastrointestinal fluids, enabling the diffusion of the encapsulated drug (Dehghan-Niri et al., 2015). Recent studies have focused on advancing oral drug delivery using Tragacanth gum-based hydrogels. For instance, the incorporation of nanoparticles or the use of stimuli-responsive hydrogels allows for site-specific drug release and enhanced therapeutic efficacy (Singh et al., 2020). Additionally, the application of novel techniques such as 3D printing enables the fabrication of personalized dosage forms and tailored drug release profiles. These advancements in oral drug delivery using Tragacanth gum-based hydrogels hold great promise for improving drug bioavailability, patient compliance, and therapeutic outcomes (Enke et al., 2023).
Studies shows that the TG polymer possesses the ability to form a gel by utilizing the carboxylic groups found in Tragacanth. Consequently, Tragacanth presents an opportunity for interaction with insulin, particularly in an acidic environment below the isoelectric point (pI) of insulin (Nur et al., 2016). Another study utilized TG to encapsulate quercetin by structuring the TG shell and forming self-assembled micelles with a polycaprolactone (PCL) core. The release of quercetin from these micelles demonstrated pH dependency, with a significant increase in release rate observed at pH 2.2 (Hemmati and Ghaemy, 2016). Through a study on gelation and mucoadhesion, it was discovered that Tragacanth exhibits potential as an excipient for the oral administration of proteins and peptides, such as insulin (Singh et al., 2017b). Tragacanth’s properties suggest that it could enhance the drug loading capacity, encapsulation efficiency, and stability of insulin encapsulated within Tragacanth particles by utilizing ionic attraction between Tragacanth and the amino groups of insulin’s amino acid residues.
Topical drug delivery is of great importance in the treatment of various dermatological conditions and localized skin disorders. Tragacanth gum-based hydrogels offer distinct advantages for topical applications. These hydrogels possess excellent mucoadhesive properties, allowing them to adhere to the skin surface, enhance drug retention, and promote efficient drug permeation through the skin barrier (TaghavizadehYazdi et al., 2021a). Tragacanth gum-based hydrogels also exhibit good biocompatibility, biodegradability, and water absorption capacity, making them suitable for prolonged drug release and sustained therapeutic effects (Li and Mooney, 2016). Strategies for controlled drug release in topical hydrogels include the incorporation of drug-loaded nanoparticles, lipid-based vesicles, or microparticles within the hydrogel matrix. These strategies enhance drug stability, control release kinetics, and improve drug penetration into the skin layers. Recent studies and developments in topical drug delivery using Tragacanth gum-based hydrogels have demonstrated their efficacy in various applications (Fayazzadeh et al., 2014). For instance, the use of Tragacanth gum-based hydrogels for wound healing (Ghorbani et al., 2021) has shown accelerated healing rates, reduced infection risk, and improved tissue regeneration (Zagórska-Dziok and Sobczak, 2020). Additionally, the incorporation of growth factors or plant-derived extracts into these hydrogels has demonstrated promising results in the treatment of skin diseases, such as psoriasis and atopic dermatitis (Homayun et al., 2019). These case studies and advancements highlight the potential of Tragacanth gum-based hydrogels as versatile platforms for targeted and controlled drug delivery in topical applications.
Injectable drug delivery systems play a crucial role in providing targeted and controlled drug release, bypassing barriers such as oral administration limitations (Chegini et al., 2020). Tragacanth gum-based hydrogels possess properties that make them suitable for injectable applications. These hydrogels exhibit shear-thinning behavior, allowing easy injection through fine-gauge needles and rapid gelation at physiological temperatures, leading to in situ gel formation at the injection site (Kulkarni et al., 2023). Moreover, their biocompatibility, biodegradability, and ability to encapsulate a wide range of therapeutic agents make them attractive candidates for injectable drug delivery systems (Vigata et al., 2020). The evaluation of drug release kinetics from injectable hydrogels involves the study of factors such as gelation time, drug loading efficiency, and drug release profiles (Singh et al., 2020). Researchers have employed techniques like diffusion studies, release kinetics analysis, and mathematical modeling to understand and optimize drug release from Tragacanth gum-based injectable hydrogels (Omidian and Chowdhury, 2023). Several examples demonstrate the effectiveness of injectable drug delivery using Tragacanth gum-based hydrogels. For instance, these hydrogels have been utilized for the localized delivery of anticancer drugs, growth factors, and stem cells for tissue regeneration and wound healing (Chahardoli et al., 2022). The injectable nature of Tragacanth gum-based hydrogels provides versatility and ease of administration, making them a promising option for targeted and sustained drug delivery002E.
Tragacanth gum-based gels hold immense potential in tissue engineering applications. These gels provide a biocompatible and biodegradable scaffold that can support cell adhesion, proliferation, and differentiation (Hama et al., 2023). Tragacanth gum-based gels have been extensively explored for the regeneration of various tissues, including skin, cartilage, bone, and nerve. The three-dimensional structure of these gels mimics the extracellular matrix, creating an optimal microenvironment for cell growth and tissue regeneration (Smagul et al., 2020). Additionally, the incorporation of bioactive molecules, growth factors, or stem cells within the gel matrix enhances the therapeutic potential and promotes tissue-specific regeneration (Polez et al., 2022). Tragacanth gum-based gels offer a versatile platform for tissue engineering, enabling the development of innovative strategies for repairing and regenerating damaged or diseased tissues Table 2.
Tragacanth gum-based hydrogels offer numerous advantages as scaffolding materials in tissue engineering (Caló and Khutoryanskiy, 2015). These hydrogels possess excellent biocompatibility, biodegradability, and water absorption capacity, making them compatible with the physiological environment. Their porous structure allows for efficient nutrient and oxygen transport, facilitating cell growth and proliferation (Potaś et al., 2020). Tragacanth gum-based hydrogels also exhibit tunable mechanical properties, which can be adjusted to match the target tissue’s requirements. Furthermore, their mucoadhesive properties enable effective adhesion to the tissue surface, providing stability and preventing displacement (Mantha et al., 2019). The hydrogel’s ability to encapsulate and release bioactive molecules or growth factors promotes cellular activities such as differentiation and tissue regeneration. The versatility of Tragacanth gum-based hydrogels enables their application in various tissue engineering approaches, including wound healing, bone regeneration, and cartilage tissue engineering (Mohammadinejad et al., 2020). Their advantages as scaffolding materials make Tragacanth gum-based hydrogels highly attractive for tissue engineering strategies, contributing to the development of effective and functional tissue substitutes.
Various strategies have been employed for incorporating cells and growth factors into Tragacanth gum-based hydrogels, enhancing their potential in tissue engineering applications (Andersen et al., 2015). One approach involves direct cell seeding, where cells are mixed with the hydrogel solution before gelation, allowing for uniform distribution of cells within the gel matrix. Alternatively, cells can be encapsulated within microspheres or nanoparticles that are subsequently incorporated into the hydrogel (Li and Mooney, 2016). This approach provides a controlled release of cells, maintaining their viability and functionality. Growth factors can be loaded into Tragacanth gum-based hydrogels either by physical entrapment or through covalent binding. Physical entrapment involves the incorporation of growth factors within the gel matrix, allowing for their sustained release over time (Fang et al., 2019). Covalent binding, on the other hand, enables a more controlled release by conjugating the growth factors to the hydrogel structure. The choice of incorporation strategy depends on factors such as the desired release kinetics, stability of growth factors, and compatibility with the hydrogel system. These strategies for incorporating cells and growth factors into Tragacanth gum-based hydrogels offer versatility and flexibility in designing tissue-engineered constructs with enhanced therapeutic potential (Dixit et al., 2022).
Case studies and recent advances have demonstrated the effectiveness of Tragacanth gum-based hydrogels as tissue engineering scaffolds. In one study, Tragacanth gum-based hydrogels were utilized as scaffolds for cartilage tissue engineering. The hydrogels showed excellent biocompatibility, supporting chondrocyte adhesion, proliferation, and extracellular matrix production. The scaffolds promoted cartilage regeneration, with enhanced mechanical properties and improved histological outcomes compared to control groups (Dixit et al., 2022). Another case study focused on bone tissue engineering, where Tragacanth gum-based hydrogels were combined with bioactive ceramic particles. The hybrid scaffolds exhibited favorable properties, such as controlled porosity, enhanced mechanical strength, and sustained release of growth factors (Polez et al., 2022). In vivo experiments demonstrated successful bone regeneration with increased bone formation and improved integration with the surrounding tissue. Recent advances in Tragacanth gum-based hydrogels include the incorporation of bioactive molecules, such as growth factors and nanoparticles, to enhance cellular response and tissue regeneration (Singh et al., 2017a). Furthermore, the combination of 3D bioprinting techniques with Tragacanth gum-based hydrogels has enabled the fabrication of complex tissue structures with precise spatial control. These case studies and advancements highlight the potential of Tragacanth gum-based hydrogels as versatile scaffolding materials for tissue engineering, offering promising solutions for tissue regeneration and repair (Singh et al., 2017b).
A cascade of many cellular and molecular activities in response to injury make up the highly coordinated, complex process of wound healing. Hemostasis, inflammation, proliferation, and remodelling are the four steps of the wound healing process (Panuncialman and Falanga, 2009). The healing of wounds is necessary for tissue regeneration, and encourages the growth of healthy tissue by increasing the amount of cells and matrix in the wound bed (Tottoli et al., 2020). Regeneration is the capacity to grow back (sections of) an organ or a tissue after injury or amputation, contrary to wound healing, that involves tissue closure and may occasionally lead to the creation of scars (He et al., 2021). A patient’s immunological condition affects the healing pace of acute wounds, which is different from the healing frequency of chronic wounds. Depending on the dimensions, depth, and degree of harm to the layers of skin (epidermis, dermis), acute wounds typically recover between 8 and 12 weeks (Robert and Jaminelli, 2015). Chronic wounds, on the other hand, cannot go through the regular physiological healing process. The extended inflammatory phase, ongoing infection, and the inability of the epidermal cells to respond to repair stimuli are all shared traits of these chronic wounds (Demidova-Rice et al., 2012). Along with a higher risk of infection, ulcers and diabetic wounds, are the main causes of chronic wounds. Chronic wounds that do not heal are caused by a variety of causes, including reduced bioavailability of growth factors and receptors, erroneous matrix protein synthesis or modification, decreased resident cell proliferation, and inadequate or impaired wound perfusion. Hyperglycemia, persisting inflammation, deficiency in growth factors and cytokines receptors, microbial infections are frequently present in chronic wounds. These conditions impair progenitor cell recruitment, angiogenesis, delayed epithelialization, and excessive proteolysis (Demidova-Rice et al., 2012). It is necessary to produce a new kind of wound dressing in order to help targeted medications and biomolecules to promote wound recovery, tightly fit the wound area and to maintain moisture in the wound.
Treatment of a wound is a complex process because three elements—cell interaction, extracellular matrix (ECM), and growth—are necessary for its regeneration. A successful wound dressing must guard against infection, offer sufficient moisture, remove exudates, and promote further tissue regeneration (Hemmatgir et al., 2022). Wound dressings were first intended to offer the wound passive protection. Traditional dressings like gauze, lint, nonstick dressings and tulle dressings cannot be regulated or sustained to release healing factors or adapt to changing wound circumstances (Hemmatgir et al., 2022). George Winter’s concept of wet healing, which totally altered wound care and gave rise to the idea of moisturising dressing (Weller and Sussman, 2006). Modern wound dressings should have the following qualities. They should show good hygroscopicity (moisture retention) and thereby effective wound exudate retention but should not adhere to wound exudate, prevent the abnormal collection of fluid in between tissues of the body (effusion), little pain and harm to the newly formed tissue during wound dressing changes; light adherence to the wound tissue; effective antimicrobial qualities that prevent wound infection and also outstanding biocompatibility (Xiang et al., 2020). Many different forms of wound dressings, such as film dressings, hydrocolloid dressings, foam dressings, and hydrogel dressings, are currently available and are based on wet healing concepts (Balakrishnan et al., 2005). The capabilities of hydrogels are of particular significance. Since hydrogel dressings are insoluble aqueous gel type moist bandages, they create a moist environment for wounds. The hydrogel matrix typically contains more than 90% water, which is ideal for maintaining a humid environment near the wound area (Zhao et al., 2020). They speed up granulation hyperplasia, epidermal restoration, and the removal of extra dead tissue. Hydrogels can crosslink in situ and have the ability to prevent the wound from desiccating, or drying up, as well as to encourage the autolytic debridement of necrotic areas (Singh and Singh, 2021). They are able to carry a variety of active wound healing agents, antimicrobials and have ease of encapsulating cells. The hydrogel’s minimal adhesion to the wound makes it simple to remove the dressing without causing further damage, which greatly decreases the discomfort and risk of infection associated with doing so (Xiang et al., 2020). Some hydrogel dressings are made to be transparent, allowing for clinical evaluation of wound healing without removing the dressing. TG has the ability to both heal wounds and act as an antioxidant, making it a potential candidate for usage in wound dressings (Ranjbar-Mohammadi and Bahrami, 2015). There was an attempt to combine TG with additional composite materials, such Polycaprolactone (PCL) (Xiang et al., 2020). The impact of PCL/TG nanofibers on granulation tissue reveals their potential as a skin scaffold or patch for treating wounds (Ranjbar-Mohammadi and Bahrami, 2015). Biomaterials based on TG and Polyvinyl alcohol (PVA) have also demonstrated effective antibacterial activities (Ranjbar-Mohammadi et al., 2013). In order to create the hybrid hydrogels, the natural polysaccharide Tragacanth gum (TG) showed compatibility with synthetic polymers (Rakkappan and Anbalagan, 2009). In experiments using TG hydrogels, tests for thrombogenicity, hemolysis, DPPH scavenging assay, and F-C reagent assay are performed. Mucoadhesion, oxygen permeability, tensile strength, burst strength, relaxation force, resilience force, folding endurance, and microbial penetration are the other tests done on the TG hydrogel. All the studies show significant results and thereby concluding TG hydrogel as an effective candidate for wound healing (Singh and Singh, 2021). In order to explore wound healing, bacterial cellulose/keratin nanofibrous mats were modified by TG conjugated hydrogel. The findings suggest that the hybrid composite scaffold could be a promising candidate for soft tissue engineering and wound dressing (Azarniya et al., 2019). Due to its favourable swelling, optimum degradation ratio, high mechanical properties, strong cell adhesion, and adequate antibacterial activity, the PEGDA (Polyethylene glycol diacrylate)-PVA-TG network made by Hemmatgir et al. can be deemed a favourable hydrogel for wound dressing purposes.
It has been demonstrated that a variety of polysaccharide biopolymers, including chitosan, alginate, and glycosaminoglycans, with hydrogel-forming capabilities, are useful as materials for wound dressing. In a study conducted by Fayazzadeh et al., in rats it has been proved the suitability of Tragacanth, a different natural substance based on arabinogalactan, as a dressing for wound occlusion and a promoter of the healing process. According to one study, the addition of TG to the wound dressing increased its elastic and mechanical characteristics (bacterial cellulose/keratin), as well as the cell adhesion and proliferation (Azarniya et al., 2019). Another instance of the use of TG dressing bandages was for burns. With that healing occurred without skin toxicity and faster full wound recovery than control has been reported. In this investigation, a full thickness wound on a rat was treated with 250 μL (5%) of Tragacanth gum solution twice daily for a total of 10 days (Fayazzadeh et al., 2014). TG was found to be helpful in topical application by speeding up the pace of skin wound contraction as well as healing. Bassorin and Tragacanthin, two of the Tragacanth gum’s active constituents, were discovered to be responsible for the wound healing action (Singh and Singh, 2021). According to Qu et al., the hydrolysis of Tragacanth into arabinose and glucuronic acid may lead to protein coagulation that speeds up wound healing When wound fluid absorption studies were performed in a hydrogel made up of TG and Sodium alginate (SA) by Singh B et al., the hydrogel wound dressing absorbed simulated wound fluid of 4.45 ± 0.11 g/g of gel. Exudates intake by dressing materials needs to be carefully controlled in order to speed up wound healing and prevent maceration of the surrounding skin (White and Cutting, 2003). Knowledge of the dressing materials’ capacity to absorb wound fluid is also necessary. Overall, the TG/SA-based dressings in this instance have the ability to absorb wound fluid, which can hasten the healing process. Studies on the water vapour permeability (WVPR) of TG-PVA hydrogel reveal an intermittent value, which is advantageous for hastening the healing of wounds. Healing of wounds is aided by maintaining a correct WVTR of a polymer film, and the hydrogel film’s porosity network can control the rate at which wound exudates are transmitted (Zheng et al., 2019). Higher WVTR causes wounds to dry up more quickly and may lead to severe wound dryness, which can hinder wound healing even more (Ahmed et al., 2018). Exudates build up and the healing process is slowed down by low WVTR. It is so important to choose a suitable wound dressing. Low and high exuding wounds can be covered by films with a lower and higher WVTR, respectively (Aini, 2018).
Hydrogels that are generated below the freezing point of a solvent are known as cryogels, which are characterised by interconnected macropores (Jones et al., 2021). For accurate oxygen and nutrition supply to cells, waste elimination, vascularization, and tissue development, an open macroporous structure with connected pores is necessary. For sufficient mechanical support, elasticity is also necessary (Demir et al., 2022). These have led to the widespread usage of cryogels in recent years. In a recent study, tragacanth gum crosslinked chitosan polyelectrolyte complex cryogel scaffolds were synthesised by cryogelation. The resultant cryogels, depending on the ratio of tragacanth gum—chitosan composition, showed an open macroporous interconnected pore structure, enhanced mechanical properties, appropriate swelling ratio and pH-sensitive swelling properties. Future applications of cryogels as controlled drug release systems may depend heavily on these properties. In a biocompatibility study conducted with tragacanth gum—chitosan cryogel matrix in Mouse Embryonic Fibroblast cells (MEF); significant adherence, high viability and cell interconnection network on the surface of the scaffold was confirmed. Additionally, the genotoxicity investigations showed that the scaffold has no potential for genotoxicity and did not cause DNA fragmentation (Demir et al., 2022). After taking into account all of the data, it was determined that the CS:GT cryogel scaffolds show a great deal of promise for tissue engineering applications, particularly when it comes to the restoration of soft tissues like muscle and skin, which in turn links to wound healing.
In recent years, Tragacanth gum has been used as a superabsorbent hydrogel, antimicrobial nanocapsules, wound dressings, skin scaffolds, and drug release systems (Meghdadi and Boddohi, 2019). Tragacanth gum, being a natural polysaccharide, has been used for producing hydrogels and drug release vehicles (Singh and Sharma, 2014; Hemmati et al., 2016). Thanks to its emulsifying ability, good thermal stability as well as high long shelf life. As stated earlier also, Tragacanth gum has been used as a natural thickener and emulsifier in cosmetics, food, drug, textile and adhesive industries. Moreover, this polysaccharide is durable over a wide pH range and highly hydrophilic to absorb water. Owing to its biocompatibility, biodegradability, non-allergenicity, non-carcinogenicity, emulsifying ability, thermal stability, and resistance in a wide range of pH, safe oral ingestion, and long shelf life, Tragacanth is an appropriate material in various fields including water purification, food packaging, textile, adhesive, and cosmetics (Mohamadisodkouieh and Kalantari, 2022). Also, the Tragacanth gum exhibits compatibility with synthetic polymers to form the blend hydrogels (Ben-Zion and Nussinovitch, 1997; Rakkappan and Anbalagan, 2009).
Tragacanth gum is non-toxic, non-mutagenic, non-teratogenic, non-carcinogenic and therefore finds applications in cosmetic, pharmaceutical, leather, and textile industries (Anderson et al., 1984; Eastwood et al., 1984; Hagiwara et al., 1992; Moghbel et al., 2008). Besides being biodegradable, Tragacanth gum based polymeric hydrogels are widely used in drug delivery systems, control release of pesticides, and metal ion recovery (Saruchi et al., 2013; Masoumi and Ghaemy, 2014; Hemmati et al., 2015; SaruchiKaith et al., 2015; Badakhshanian et al., 2016; Hemmati et al., 2016; Hosseini et al., 2016). The Tragacanth structure can be easily modified because of its different functional groups such as hydroxyl and carboxylic acid which can contribute to cross-linking reactions (Pathania et al., 2018). As a biomaterial, Tragacanth gum has been applied in industrial settings, such as in food packaging (Tonyali et al., 2018), the processing of bioproducts as an additive (Ghayempouret al., 2015), cosmetics as an emulsifying and suspending agent in a variety of pharmaceutical formulations (BeMiller and Whistler, 2012), for environmental purposes of purification of water polluted with dyes and toxic heavy metal ions (Zare et al., 2018), nanofibers and textiles (Schiffman and Schauer, 2013), in the green synthesis of reducing agents and stabilizers (Darroudi et al., 2013), for antimicrobial applications (Monjezi et al., 2019), biomolecules and drug delivery (Gupta and Chatterjee, 2018), wound healing (Anita Lett et al., 2019), bone tissue engineering (Fang et al., 2011) immobilization and cell growth matrix (Torres et al., 2012), as well as the regeneration of damaged peripheral nerves.
Although pure Tragacanth gum has a degree of absorption capacity, it still raises questions. By mixing Tragacanth gum with water, it can swell as the insoluble fraction, bassorin (60%–70%), swells to form a gel (Gupta and Chatterjee, 2018). The acidic and ionic units in the chemical structure of gums are responsible for their different hygroscopic properties. Studies on the water absorption properties of various gums at temperatures between 20°C and 65°C have shown that Tragacanth gum has higher water absorption than guar gum and locust bean gum (Behrouzi and Moghadam, 2018). Therefore, it can be considered an absorbent material but not yet a superabsorbent material. Recently, some researchers have developed SAPs based on Tragacanth gum. For example, a superabsorbent co-polymer synthesized by graft polymerization of acrylic acid onto carboxymethyl Tragacanth gum (Khoylou and Naimian, 2009), a superabsorbent composed of Tragacanth gum and polyethylene oxide (PEO) obtained by gamma radiation (L´opez-Castej´on et al., 2016), as well as a bioplastic absorbent material produced by a mixture of TG and egg white (Liu et al., 2018). As stated above, the use of biopolymers as superabsorbent materials requires some chemical modifications, in particular raising the number of anionic parts in the saccharide backbone. The chemical process of carboxymethylation of biopolymers involves the reaction of hydroxyl groups with saccharide units. It has been reported as one of the methods of grafting carboxylate groups into the matrix of biopolymers such as cellulose (Wang et al., 2020), starch (Wang et al., 2020), chitosan (Lou et al., 2020), guar gum (Gupta and Verma, 2015), Tragacanth gum (Torres et al., 2012), and tamarind gum (Khushbu et al., 2019). Unlike conventional cross linking techniques, acetalization with dialdehydes has been reported as an environmentally friendly method to avoid radical polymerization, based on acrylic monomers and MBA (N,N′-methylene bismethacrylamide). Thus, glutaraldehyde (GA), a dialdehyde generally used for protein binding and sterilization, can also be induced to form acetal groups by reaction with hydroxyl groups (Hemmati et al., 2015; Ngwabebhoh et al., 2016; Kumar et al., 2017). Nowadays, considering the turbulent economic conditions that the world is facing due to the consequences of the COVID-19 pandemic that is creating a global war on the price of petroleum and its derivatives, the biggest challenge in the field of biodegradable SAPs is to synthesise or manufacture superabsorbent materials based on fully biodegradable polymers that would absorb and retain water rapidly and reversibly (Bachra et al., 2020). For these reasons, our approach to the development of SAPs is to synthesise an environmentally friendly superabsorbent material based on a fully biodegradable polymer (Tragacanth gum) that would absorb and retain water rapidly and reversibly while being competitive with synthetic SAPs on the market in terms of absorption capabilities under specific conditions and market price. Therefore, the approach will be carried out through a carboxymethylation reaction followed by acetalization crosslinking to avoid the side effects of radical polymerization as described above.
Artificial nanofibers made from natural gum have been produced by electrospinning the gum and eliminating the insoluble components. The production of electrospun fibers made of TG/PVA (Tragacanth gum/polyvinyl alcohol) was accomplished by combining three distinct concentrations of TG/PVA (9, 6, and 3 weight percent) in a different combination (TG/PVA; 100/0 to 0/100) (Zarekhalili et al., 2017). In order to ensure the smooth production of nanofibers, other restrictions, including those pertaining to the environment, processes, and systems, were controlled. The ratio of PVA to TG was 60/40. Phenomenal antibacterial viability of TG/PVA was discovered about Gram-negative pathogens (P. aeruginosa), and the nanofibers exhibited outstanding compatibility with biological processes and cell growth. (Ranjbar-Mohammadi et al., 2013; Ahmad et al., 2019).
There is no doubt that the search for the perfect hydrogel made from plants that can imitate the characteristics of human tissue continues to be a difficult task. The use of tragacanth gum in conjunction with cellulose nanofibrils and lignin nanoparticles resulted in the creation of a unique multi-component hydrogel that was natural, sustainable, and biocompatible. As a result of the synergistic effects of the antioxidant activity of lignin, the analgesic action of tragacanth gum, and the rheological features of TEMPO-oxidized cellulose nanofiber (TOCNF), the hydrogel is ideal for use in tissue engineering. The hydrogels exhibited shear-thinning behavior as a result of TOCNF, and they also possessed visco-elastic properties that could be tuned as a result of TG. This combination made it possible to print scaffolds in three dimensions with great printability. The scaffolds exhibited a high swelling ratio, and the breakdown rate was accelerated by increasing the amount of TG that was present in the hydrogels when measured. After conducting an analysis of the mechanical properties, it was discovered that hydrogels are soft gels that have a level of stiffness that falls in between that of skin and muscle tissues. It was also simple to modify the hydrogels’ porosity, viscosity, and stiffness by adjusting the TG ratio, which made it possible to optimize the hydrogels for specific uses. In tests of cell viability, it was found that the scaffolds that were created via 3D printing were not harmful and that they encouraged the proliferation of HepG2 cells. Because of all of these characteristics, these plant-based multi-component hydrogels are great candidates for the construction of soft materials that can be used in tissue engineering applications (Polez et al., 2022).
Due to its great sensitivity to toxins, the hepatocellular carcinoma cell line, also known as HepG2, was utilized as a model cell line for the purpose of assessing cytotoxicity and cell proliferation (Zheng et al., 2019, Mersch-Sundermann et al., 2004). Additionally, cells were implanted onto pure TOCNF in addition to scaffolds composed of several components. The WST-1 test was utilized in order to ascertain the rate of proliferation based on the metabolic activity of mitochondria through the utilization of colorimetric analysis. Biomaterials based on TOCNF have already been investigated, and they have been found to have a high level of biocompatibility. Furthermore, when cultivated with myoblasts, fibroblasts, or liver cancer cells, there were no indications of any toxicological effects. (Ajdary et al., 2019). All of the samples exhibited a high level of cell viability (more than 90 percent), indicating that the scaffolds did not exhibit any signs of toxicity to the cells. There was a significant increase in the cell viability of the 3D hydrogel scaffolds when compared to the control samples, which consisted of cells grown directly on a culture plate in 2D. More surfaces were made available for cell seeding due to the three-dimensional structure, swelling, and porosity of the scaffolds that contained TG. Additionally, the scaffolds made it easier for cells to exchange nutrients and waste products with the surrounding environment (Liu et al., 2018). After 1,3 and 5 days of culture, the hybrid hydrogels’ optical density, which was determined by colorimetric analysis at 420 nm, revealed significant changes in the metabolic activity of the cells (p < 0.05). Through the synthesis of the formazan product, which is closely connected with cell survival and proliferation, high optical density readings imply increased metabolic activity. This is because of the production of the formazan product. All of the scaffold compositions demonstrated good cell viability throughout the entirety of the incubation time, which indicates that the scaffolds did not inflict any deleterious effects on the cells. In addition, the increase in cell proliferation that occurred as a result of the increased TG content can be attributed to the fact that the greater swelling ratio improved the surface area that was available for cell growth. There was no evidence of cytotoxicity of TG against human fibroblast cells or human epithelial cells in the investigations that were conducted in the past (Ghayempour et al., 2015). This provides more evidence that the hydrogels offered an environment and surface area that were conducive to the adhesion, survival, and proliferation of certain cell types.
In certain tissue engineering procedures, the eventual disintegration of the scaffolds is a typical strategy. In these strategies, the by-products of the degraded scaffold must be non-toxic and able to leave the body without interfering with other organs within the body. Therefore, cellulose-based materials are, in principle, suitable candidates for implants in situations where scaffold breakdown is not necessary. This is due to the fact that both humans and animals lack the enzyme cellulase. Degradation tests, on the other hand, have the potential to provide information regarding the stability of the scaffolds under physiological settings. The assessment of the degradation of the hydrogel scaffolds was conducted by analyzing the loss of mass following incubation in a solution of DPBS (Dulbecco’s phosphate buffered saline) at a temperature of 37°C and 5% carbon dioxide for a sequence of days. A more pronounced deterioration was found in the scaffolds that included larger quantities of TG, particularly over a period of at least 10 days. Following a period of 20 days, the rate of degradation was as follows: 0.4% per day for TG00, 0.8% per day for TG10, 1.4% per day for TG25, 1.3% per day for TG50, and 1.3% per day for TG75. The dissociation of the components that were loosely linked in the network during the washing with DI water, drying, and handling of the samples can be a possible explanation for the decrease in mass. The presence of a high degradation rate may be indicative of the mild cross-linking of the components that was established by Ca+2 ions, which ultimately results in the release of the components that were not cross-linked in an adequate manner. Therefore, it appears from the findings that TG was beneficial to the breakdown of the scaffolds. Enzymes would be required in order to achieve a more comprehensive or expedited breakdown. For instance, the abundant glycosidic connections, such as a β-one to four linked arabinogalactan, and the linkages that are comparable to pectin in TG, are capable of being broken by the action of enzymes (Gavlighi et al., 2013a; Kulanthaivel et al., 2017a). The degradation tests that were carried out in previous reports were carried out with a matrix of degrading enzymes that mimicked the in vivo process. In these research, TG was mixed into sodium alginate beads, and the breakdown rate reached around 80 percent (Kulanthaivel et al., 2017a). Additionally, some in vitro investigations have indicated that the gastrointestinal tract undergoes complete breakdown of TG substances (Gavlighi et al., 2013a).
In their research, Ranjbar-Mohammadi and Bahrami (2015) came to the conclusion that as compared to pure poly ε-caprolactone (PCL), the addition of TG made a significant reduction in the diameter of the fibers and altered the shape of the fibers. The morphology of the scaffolds that were produced from 7% TG and 20% PCL was superior, and a composition of 3:1.5 (PCL/TG) with a significant proportion of TG was chosen for subsequent tests. PCL/TG scaffolds were able to successfully attach and promote the proliferation of human fibroblasts as well as NIH 3T3 fibroblast cells. The hydrophilicity of the nanofibers, the degradation behavior, the mechanical strength, the excellent morphology of the cells on the PCL/TG nanofibers, and the cytotoxicity assessment methodologies shown that these scaffolds are safe and have the potential to be developed as skin scaffolds or wound dressing patches.
Based on their research, Sodkouieh and Kalantari (2022) reported the production of a cheap semi-synthetic hydrogel by crosslinking tragacanth gum with ethylene glycol. The hydrogel has promising agricultural applications. The products’ ability to absorb water was maximized by optimizing the synthesis conditions. At a temperature of 100°C, with a time constant of 4 h and a pH of 6, the ideal circumstances for EG concentration were 0.0064 M. The maximum water absorption was equal to 490 g g−1, representing a 528% increase compared to the absorption capacity of pure tragacanth. The FT-IR, TGA, SEM, and XRD techniques were utilized in order to do an investigation into the properties of both the pure tragacanth and the hydrogel that was generated. The results demonstrated that the cross-linking method was successful in producing the hydrogel that was supposed to be produced. In order to determine whether or not the hydrogel is capable of conserving water for agricultural purposes, two parameters were measured. These parameters were the water holding capacity (WH) and the water retention ratio (WR). As a consequence of the presence of hydrogel, both of the metrics witnessed an increase. In addition, the influence of hydrogel on the fertility of soil was explored by planting barley seeds in soil that contained hydrogel as well as soil that did not contain hydrogel. The results showed that the presence of hydrogel resulted in a 6.5-fold increase in the growth of the plants. These results make it abundantly evident that hydrogels based on tragacanth gum have a tremendous amount of potential to solve issues that are related with the agricultural industry, including those pertaining to soil fertility and water retention.
The synthesis and characterisation of two different types of antimicrobial graft copolymer hydrogels found on natural biopolymer TG were accomplished by Monjezi et al., 2018. These hydrogels were referred to as QTG-AA and QTG-AM accordingly. This work focuses primarily on the development of pH-responsive hydrogels that are antibacterial and antifungal in nature. These hydrogels are based on a biopolymer called Quaternary Ammonium Functionalized-Tragacanth Gum (QTG), which is used as a drug delivery vehicle. A total of five typical bacteria, namely, Candida albicans, Escherichia coli, Bacillus subtilis, Staphylococcus aureus, and P. aeruginosa, were included in the study that studied the antimicrobial properties of the graft-copolymer hydrogels QTG/polyacrylic acid (QTG-AA) and QTG/polyacrylamide (QTG-AM). The results of the in-vitro release of quercetin as a drug from the functionalized copolymer hydrogels showed that the pH, immersion period, medium, and temperature all had a role in the release of the drug. Antibacterial and antifungal characteristics were revealed by all of the copolymer hydrogels. Furthermore, the antimicrobial activity of QTG-AM copolymers was found to be higher than that of QTG-AA copolymers. The antimicrobial graft copolymer hydrogels that were created released the medicine that was placed into them in a regulated manner. These hydrogels also possessed salt- and pH-responsive characteristics. Furthermore, the examined microorganisms were susceptible to the antibacterial and antifungal effects of both the QTG-AA and QTG-AM hydrogels.
In their study, Shirazi et al., 2021 investigated the development and properties of nano hydrogels composed of Keratin and Tragacanth gum for use in medical garments containing drug delivery systems. Using the MTT assay on L929 fibroblast cells after varying incubation durations, they determined the viability of nanogels with and without cinnamon at varied doses (i.e., 2.5–40 mg/mL). However, cell viability above 80% is achieved at all concentrations (even at relatively high concentrations up to 40 mg/mL), suggesting the good biocompatibility of the fabricated TGK nanogels. This is in contrast to the decreasing cell viability observed with increasing nanogels content, for example, from 100% to 90% for 2.5 and 40 mg/mL CE-loaded nanogels at 24 h, respectively. This reduction in viability that was dose-dependent was also investigated by other researchers (Li et al., 2012). Keratin and TG are biopolymers that have been extensively researched for their potential biomedical uses; they are also biocompatible and biodegradable. Because keratin has cell-binding motifs in its protein structure, keratin biomaterials can facilitate cellular adhesion and growth (Feroz et al., 2020). Additionally, TG has demonstrated an improvement in fibroblast cell survival, adhesion, and proliferation; it is non-toxic, non-carcinogenic, and non-allergenic (Taghavizadeh Yazdi et al., 2021a).
Natural macromolecules or hydrocolloids like gums are attracting more attention as a result of the growing desire from consumers and regulatory bodies to reduce or eliminate ‘additives’ from food and other health-related products. As a result of its many useful properties, the multi-exudate gum tragacanth gum (TG) has found widespread application in many different areas of food science, including i) non-fat or low-fat food formulations, ii) colloid-based products, iii) edible films and coatings, and iv) nano-encapsulation of food ingredients. In addition to being great model systems, research has shown that TG and its components can stabilize actual food products (Nejatian et al., 2020a). The browning index was significantly reduced and the rehydration quality was significantly improved after coating banana slices with GT solution (0.7% w/w) (Farahmandfar and Asnaashari, 2017). After 90 days of storage, total mesophilic aerobic bacteria in Cheddar cheese were shown to be drastically reduced when coated with 1% GT, as compared to the control (polyvinyl acetate). Additionally, the coating enhanced fat oxidation prevention (Pourmolaie et al., 2018). GT-based edible coating for fresh-cut apple slices was made with calcium chloride (0.5%) and ascorbic acid (2%). This coating protects vitamin C better than soybean soluble polysaccharide-based coating for 12 days (Jafari et al., 2018). GT-based coatings can also carry antimicrobials and essential oils. By covering button mushroom with GT (0.6%) and Zataria multiflora Boiss or Satureja khuzistanica essential oil (100–1,000 ppm), researchers improved quality and shelf life. Their studies showed that the GT layer around the product preserved tissue firmness (93%) and reduced microbial contamination and browning index. (Nasiri et al., 2017; Nasiri et al., 2018).
Tragacanth gum is biocompatible and safe even for oral intake, is a medicinally imported carbohydrate, and possesses no cytotoxicity (Greenwald et al., 1980; Weiping et al., 2000; Balbir et al., 2016). Also, Tragacanth gum is “generally regarded as safe” (GRAS). This gum, which has the functions of stabiliser, emulsifier, and thickener, has been approved by the US FDA. This drug has also been approved by the European Union under the function of food additive in the class of thickeners, stabilisers, emulsifiers, and gelling agents (Kora and Arunachalam, 2012). In addition, Tragacanth gum is a medically important polysaccharide that has been approved by the FDA (Zare et al., 2019). It is also non-toxic, stable over a wide pH range, biocompatible, and safe for oral intake according to REACH regulations (ECHA, 2017); therefore Tragacanth gum is widely used for different applications.
In the field of Tragacanth gum-based hydrogels for drug delivery and tissue engineering, there are several emerging trends and future directions that hold great promise. Researchers have been actively investigating innovative strategies to enhance the properties and functionality of these hydrogels. For instance, the incorporation of bioactive molecules and nanoparticles into the hydrogel matrix has gained significant attention. As demonstrated by Smith et al., the inclusion of growth factors, cytokines, or antimicrobial agents within the hydrogels allows for localized and sustained release, promoting tissue regeneration and combating infections (Fan et al., 2021). Additionally, the integration of nanoparticles, such as metallic nanoparticles or drug-loaded nanocarriers, offers the potential for targeted drug delivery, improved stability, and enhanced therapeutic efficacy (Desai et al., 2021). Hybrid systems that combine Tragacanth gum-based hydrogels with other biomaterials or techniques are also emerging as a promising trend (Polez et al., 2022). By incorporating synthetic polymers like polyethylene glycol (PEG) or poly(lactic-co-glycolic acid) (PLGA) into the hydrogels, researchers have been able to enhance mechanical strength, stability, and tunable degradation rates (Makadia and Siegel, 2011). Moreover, the fusion of Tragacanth gum-based hydrogels with advanced manufacturing techniques such as 3D printing or electrospinning enables the fabrication of intricate structures and tissue-engineered scaffolds with precise architectures and functionalities (Seidi et al., 2021). Another notable trend in the field involves the utilization of advanced characterization techniques and computational modeling. These approaches provide valuable insights into the structure-property relationships of Tragacanth gum-based hydrogels. Techniques such as rheology, scanning electron microscopy (SEM), and atomic force microscopy (AFM) facilitate the understanding of mechanical behavior, morphology, and interfacial interactions of the hydrogels at different scales (Rao et al., 2017). Computational modeling, on the other hand, allows for the prediction and simulation of drug release kinetics, swelling behavior, and mechanical properties, aiding in efficient design and optimization (Pareek et al., 2017). Looking ahead, future directions in the field of Tragacanth gum-based hydrogels encompass various aspects. These include addressing scalability and commercialization challenges, conducting comprehensive in vivo studies to evaluate safety and efficacy, and exploring the potential of combinational therapies by integrating Tragacanth gum-based hydrogels with advanced techniques such as gene therapy or stem cell transplantation. Continual research and development in these areas, as highlighted by Smith et al., will undoubtedly drive the successful translation of Tragacanth gum-based hydrogels into clinical applications, revolutionizing the fields of drug delivery and tissue engineering (Mohammadinejad et al., 2020).
In conclusion, the key findings and applications of Tragacanth gum-based hydrogels highlight their immense potential in the fields of drug delivery and tissue engineering. These hydrogels offer excellent biocompatibility, biodegradability, and water absorption capacity, making them suitable for encapsulating therapeutic agents and supporting tissue regeneration. The incorporation of bioactive molecules and nanoparticles further enhances their functionality, enabling localized and sustained drug release as well as targeted delivery. The combination of Tragacanth gum with other biomaterials or techniques creates hybrid systems with improved mechanical properties and precise control over degradation rates. Advanced characterization techniques and computational modelling provide valuable insights into their structure-property relationships. Looking ahead, future research should focus on addressing scalability and commercialization challenges to enable widespread adoption of Tragacanth gum-based hydrogels. Comprehensive in vivo studies are essential to evaluate their safety and efficacy in various biological systems. Exploring combinational therapies by integrating these hydrogels with gene therapy or stem cell transplantation holds great potential for advanced tissue engineering applications. Furthermore, efforts should be directed towards optimizing manufacturing processes and ensuring regulatory compliance for commercialization. Overall, Tragacanth gum-based hydrogels offer promising solutions for drug delivery and tissue engineering. Further research and development in these areas will contribute to their successful translation into clinical applications, revolutionizing healthcare and opening new avenues for regenerative medicine.
GA: Conceptualization, Investigation, Resources, Software, Supervision, Visualization, Writing–original draft, Writing–review and editing. MJ: Conceptualization, Data curation, Investigation, Methodology, Writing–original draft. NP: Conceptualization, Investigation, Software, Writing–original draft. MT: Writing–original draft. SC: Conceptualization, Methodology, Software, Validation, Writing–original draft. PK: Conceptualization, Data curation, Project administration, Validation, Writing–original draft. NR: Conceptualization, Investigation, Methodology, Writing–original draft. SM: Conceptualization, Formal Analysis, Methodology, Writing–original draft. UU: Conceptualization, Data curation, Methodology, Writing–original draft.
The author(s) declare that no financial support was received for the research, authorship, and/or publication of this article.
The authors declare that the research was conducted in the absence of any commercial or financial relationships that could be construed as a potential conflict of interest.
All claims expressed in this article are solely those of the authors and do not necessarily represent those of their affiliated organizations, or those of the publisher, the editors and the reviewers. Any product that may be evaluated in this article, or claim that may be made by its manufacturer, is not guaranteed or endorsed by the publisher.
Ahmad, S., Ahmad, M., Manzoor, K., Purwar, R., and Ikrama, S. (2019). A review on latest innovations in natural gums based hydrogels: preparations and applications. Int. J. Biol. Macromol. 136, 870–890. doi:10.1016/j.ijbiomac.2019.06.113
Ahmed, A., Getti, G., and Boateng, J. (2018). Ciprofloxacin-loaded calcium alginate wafers prepared by freeze-drying technique for potential healing of chronic diabetic foot ulcers. Drug Deliv. Transl. Res. 8, 1751–1768. doi:10.1007/s13346-017-0445-9
Aini, A. N. (2018). “Hydrogel film of polyethylene oxide-polypropylene glycol diacrylate for wound dressing application,” in IOP Conference Series: Materials Science and Engineering, China, October 31, 2023 (UGC).403.
Ajdary, R., Huan, S., Zanjanizadeh Ezazi, N., Xiang, W., Grande, R., Santos, H. A., et al. (2019). Acetylated nanocellulose for singlecomponent bioinks and cell proliferation on 3D-printed scaffold. Biomacromolecules 20 (7), 2770–2778.
Alam, S., Kalita, H., Jayasooriya, A., Samanta, S., Bahr, J., Chernykh, A., et al. (2014). 2-(Vinyloxy) ethyl soyate as a versatile platform chemical for coatings: an overview. Eur. J. Lipid Sci. Technol. 116 (1), 2–15. doi:10.1002/ejlt.201300217
Alqahtani, M. S., Kazi, M., Alsenaidy, M. A., and Ahmad, M. Z. (2021). Advances in oral drug delivery. Front. Pharmacol. 12, 618411. doi:10.3389/fphar.2021.618411
Amara, A. A. A. F. (2022). Natural polymer types and applications. Biomol. Nat. Sources Adv. Appl., 31–81. doi:10.1002/9781119769620.ch2
Amiri, M. S., Joharchi, M. R., Nadaf, M., and Nasseh, Y. (2020). Ethnobotanical knowledge of Astragalus spp.: the world’s largest genus of vascular plants. Avicenna J. Phytomed. 10, 128–142.
Amiri, M. S., Joharchi, M. R., and TaghavizadehYazdi, M. E. (2014). Ethno-medicinal plants used to cure jaundice by traditional healers of Mashhad, Iran. Iran. J. Pharm. Res. 13, 157–162.
Andersen, T., Auk-Emblem, P., and Dornish, M. (2015). 3D cell culture in alginate hydrogels. Microarrays 4 (2), 133–161. doi:10.3390/microarrays4020133
Anderson, D., and Grant, D. (1988). The chemical characterization of some Astragalus gum exudates. Food Hydrocoll. 2, 417–423. doi:10.1016/s0268-005x(88)80006-7
Anderson, D. M. W., Ashby, P., Busuttil, A., Kempson, S. A., and Lawson, M. E. (1984). Transmission electron microscopy of heart and liver tissues from rats fed with gums Arabic and tragacanth. Toxicol. Lett. 21, 83–89. doi:10.1016/0378-4274(84)90227-3
Anderson, D. M. W., and Bridgeman, M. M. E. (1985). The composition of the proteinaceous polysaccharides exuded by Astragalus microcephalus, A. gummifer and A. kurdicus-the sources of Turkish gum tragacanth. Phytochemistry 24 (10), 2301–2304. doi:10.1016/s0031-9422(00)83031-9
Anita Lett, J., Sundareswari, M., Ravichandran, K., Latha, B., and Sagadevan, S. (2019). Fabrication and characterization of porous scaffolds for bone replacements using gum tragacanth. Mater. Sci. Eng. C 96, 487–495. doi:10.1016/j.msec.2018.11.082
Asadian, G., Kolahci, N., and Sadeghimanesh, M. (2009). An investigation on the effect and type of construct in different times on amount of gum tracaganth production in yellow milk-vetch (Astragalus parrowianus). Iran. J. Res. Const. Nat. Resour. 21 (4), 170–175.
Aspinall, G. O., and Baillie, J. (1963). 318. Gum tragacanth. Part I. Fractionation of the gum and the structure of tragacanthic acid. J. Chem. Soc. (Resumed), 1702–1714. doi:10.1039/jr9630001702
Azarniya, A., Tamjid, E., Eslahi, N., and Simchi, A. (2019). Modification of bacterial cellulose/keratin nanofibrous mats by a tragacanth gum-conjugated hydrogel for wound healing. Int. J. Biol. Macromol. 134, 280–289. doi:10.1016/j.ijbiomac.2019.05.023
Bachra, Y., Grouli, A., Damiri, F., Bennamara, A., and Berrada, M. (2020). A new approach for assessing the absorption of disposable baby diapers and superabsorbent polymers: a comparative study. Results Mater. 8, 100156. Article ID 100156. doi:10.1016/j.rinma.2020.100156
Bachra, Y., Grouli, A., Damiri, F., Talbi, M., and Berrada, M. (2021). A novel superabsorbent polymer from crosslinked carboxymethyl tragacanth gum with glutaraldehyde: synthesis, characterization, and swelling properties. Int. J. Biomaterials 2021, 1–14. doi:10.1155/2021/5008833
Badakhshanian, E., Hemmati, K., and Ghaemy, M. (2016). Enhancement of mechanical properties of nanohydrogels based on natural gum with functionalized multiwall carbon nanotube: study of swelling and drug release. Polymer 90, 282–289. doi:10.1016/j.polymer.2016.03.028
Balaghi, S., Mohammadifar, M. A., and Zargaraan, A. (2010). Physicochemical and rheological characterization of gum tragacanth exudates from six species of Iranian Astragalus. Food Biophys. 5 (1), 59–71. doi:10.1007/s11483-009-9144-5
Balaghi, S., Mohammadifar, M. A., Zargaraan, A., Gavlighi, H. A., and Mohammadi, M. (2011). Compositional analysis and rheological characterization of gum tragacanth exudates from six species of Iranian Astragalus’. Food Hydrocolloids. Food Hydrocoll. 25 (7), 1775–1784. doi:10.1016/j.foodhyd.2011.04.003
Balakrishnan, B., Mohanty, M., Umashankar, P. R., and Jayakrishnan, A. (2005). Evaluation of an in situ forming hydrogel wound dressing based on oxidized alginate and gelatin. Biomaterials 26 (32), 6335–6342. doi:10.1016/j.biomaterials.2005.04.012
Balbir, S. K., Rajiv, J., and Rachna, S. (2016). Study of ionic charge dependent salt resistant swelling behavior and removal of colloidal particles using reduced gum rosinpoly (acrylamide)-based green flocculant. Iran. Polym. J. 25, 349–362. doi:10.1007/s13726-016-0427-7
Barak, S., Mudgil, D., and Taneja, S. (2020). Exudate gums: chemistry, properties and food applications–a review. J. Sci. Food Agric. 100 (7), 2828–2835. doi:10.1002/jsfa.10302
Bartil, T., Bounekhel, M., Cedric, C., and Jérôme, R. (2007). Swelling behavior and release properties of pH-sensitive hydrogels based on methacrylic derivatives. Acta Pharm. 57 (3), 301–314. doi:10.2478/v10007-007-0024-6
Bashir, S., Hina, M., Iqbal, J., Rajpar, A. H., Mujtaba, M. A., Alghamdi, N. A., et al. (2020). Fundamental concepts of hydrogels: synthesis, properties, and their applications. Polymers 12 (11), 2702. doi:10.3390/polym12112702
Behrouzi, M., and Moghadam, P. N. (2018). Synthesis of a new superabsorbent copolymer based on acrylic acid grafted onto carboxymethyltragacanth. Carbohydr. Polym. 202, 227–235. doi:10.1016/j.carbpol.2018.08.094
BeMiller, J., and Whistler, R. (2012). Industrial gums: polysaccharides and 4eir derivatives. Cambridge, MA, USA: Academic Press.
Ben-Zion, O., and Nussinovitch, A. (1997). Physical properties of hydrocolloid wet glues. Food Hydrocoll. 11 (4), 429–442. doi:10.1016/s0268-005x(97)80041-0
Boamah, P. O., Afoakwah, N. A., Onumah, J., Osei, E. D., and Mahunu, G. K. (2023). Physicochemical properties, biological properties and applications of gum tragacanth-A review. Carbohydr. Polym. Technol. Appl. 5, 100288. doi:10.1016/j.carpta.2023.100288
Caló, E., and Khutoryanskiy, V. V. (2015). Biomedical applications of hydrogels: a review of patents and commercial products. Eur. Polym. J. 65, 252–267. doi:10.1016/j.eurpolymj.2014.11.024
Chahardoli, A., Jamshidi, N., Varvani, A., Shokoohinia, Y., and Fattahi, A. (2022). “Application of micro-and nanoengineering tragacanth and its water-soluble derivative in drug delivery and tissue engineering,” in Micro-and nanoengineered gum-based biomaterials for drug delivery and biomedical applications (Germany: Elsevier), 409–450.
Chawla, R., and Patil, G. (2010). Soluble dietary fiber. Compr. Rev. Food Sci. Food Saf. 9, 178–196. doi:10.1111/j.1541-4337.2009.00099.x
Chegini, S. P., Varshosaz, J., Sadeghi, H. M., Dehghani, A., and Minaiyan, M. (2020). Shear sensitive injectable hydrogels of cross-linked tragacanthic acid for ocular drug delivery: rheological and biological evaluation. Int. J. Biol. Macromol. 165, 2789–2804. doi:10.1016/j.ijbiomac.2020.10.164
Darroudi, M., Sabouri, Z., Kazemi Oskuee, R., Khorsand Zak, A., Kargar, H., and Hamid, M. H. N. A. (2013). Sol-gel synthesis, characterization, and neurotoxicity effect of zinc oxide nanoparticles using gum tragacanth. Ceram. Int. 39 (8), 9195–9199. doi:10.1016/j.ceramint.2013.05.021
Dehghan-Niri, M., Tavakol, M., Vasheghani-Farahani, E., and Ganji, F. (2015). Drug release from enzyme-mediated in situ-forming hydrogel based on gum tragacanth–tyramine conjugate. J. biomaterials Appl. 29 (10), 1343–1350. doi:10.1177/0885328214568468
Demidova-Rice, T. N., Durham, J. T., and Herman, I. M. (2012). Wound healing angiogenesis: innovations and challenges in acute and chronic wound healing. Adv. wound care 1 (1), 17–22. doi:10.1089/wound.2011.0308
Demir, D., Aşık Uğurlu, M., Ceylan, S., Sakım, B., Genç, R., and Bölgen, N. (2022). Assessment of chitosan: gum tragacanth cryogels for tissue engineering applications. Polym. Int. 71 (9), 1109–1118. doi:10.1002/pi.6372
Desai, N., Momin, M., Khan, T., Gharat, S., Ningthoujam, R. S., and Omri, A. (2021). Metallic nanoparticles as drug delivery system for the treatment of cancer. Expert Opin. drug Deliv. 18 (9), 1261–1290. doi:10.1080/17425247.2021.1912008
Dhingra, D., Michael, M., Rajput, H., and Patil, R. T. (2012). Dietary fibre in foods: a review. J. Food Sci. Tech. 49 (3), 255–266. doi:10.1007/s13197-011-0365-5
Dickinson, E. (2003). Hydrocolloids at interfaces and the influence on the properties of dispersed systems. Food Hydrocoll. 17 (1), 25–39. doi:10.1016/s0268-005x(01)00120-5
Dickinson, E. (2009). Hydrocolloids as emulsifiers and emulsion stabilizers. Food Hydrocoll. 23 (6), 1473–1482. doi:10.1016/j.foodhyd.2008.08.005
Diolosà, M., Donati, I., Turco, G., Cadenaro, M., Di Lenarda, R., Breschi, L., et al. (2014). Use of methacrylate-modified chitosan to increase the durability of dentine bonding systems. Biomacromolecules 15 (12), 4606–4613. doi:10.1021/bm5014124
Dixit, K., Kulanthaivel, S., Agarwal, T., Pal, K., Giri, S., Maiti, T. K., et al. (2022). Gum tragacanth modified nano-hydroxyapatite: an angiogenic-osteogenic biomaterial for bone tissue engineering. Ceram. Int. 48 (10), 14672–14683. doi:10.1016/j.ceramint.2022.02.002
Eastwood, M. A., Brydon, W. G., and Anderson, D. M. W. (1984). The effects of dietary gum tragacanth in man. Toxicol. Lett. 21, 73–81. doi:10.1016/0378-4274(84)90226-1
Elias, H. G. (1992). ‘Makromoleküle. Band2, technologie’. Basel, Heidelberg, New York: Hüthig and WepfVerlag.
Enke, M., Schwarz, N., Gruschwitz, F., Winkler, D., Hanf, F., Jescheck, L., et al. (2023). 3D screen printing technology enables fabrication of oral drug dosage forms with freely tailorable release profiles. Int. J. Pharm. 642, 123101. doi:10.1016/j.ijpharm.2023.123101
Fan, F., Saha, S., and Hanjaya-Putra, D. (2021). Biomimetic hydrogels to promote wound healing. Front. Bioeng. Biotechnol. 9, 718377. doi:10.3389/fbioe.2021.718377
Fang, J., Li, P., Lu, X., Fang, L., Lü, X., and Ren, F. (2019). A strong, tough, and osteoconductive hydroxyapatite mineralized polyacrylamide/dextran hydrogel for bone tissue regeneration. Actabiomaterialia 88, 503–513. doi:10.1016/j.actbio.2019.02.019
Fang, Y., Huang, X. J., Chen, P. C., and Xu, Z. K. (2011). Polymer materials for enzyme immobilization and their application in bioreactors. BMB Rep. 44 (2), 87–95. doi:10.5483/bmbrep.2011.44.2.87
Farahandfar, M. M., and Asnaashari, M. M. (2017). Effects of quince seed, almond and tragacanth gum coating on the banana slices properties during the process of hot air drying. Food Sci. Nutr. 5 (6), 1057–1064. doi:10.1002/fsn3.489
Fayazzadeh, E., Rahimpour, S., Ahmadi, S. M., Farzampour, S., Anvari, M. S., Boroumand, M. A., et al. (2014). Acceleration of skin wound healing with tragacanth (Astragalus) preparation: an experimental pilot study in rats. ActaMedicaIranica 52, 3–8.
Feroz, S., Muhammad, N., Ratnayake, J., and Dias, G. (2020). Keratin - based materials for biomedical applications. Bioact. Mater 5, 496–509. doi:10.1016/j.bioactmat.2020.04.007
Funami, T. (2011). Next target for food hydrocolloid studies: texture design of foods using hydrocolloid technology. Food Hydrocoll. 25 (8), 1904–1914. doi:10.1016/j.foodhyd.2011.03.010
Gadziński, P., Froelich, A., Jadach, B., Wojtyłko, M., Tatarek, A., Białek, A., et al. (2023). Ionotropic gelation and chemical crosslinking as methods for fabrication of modified-release gellan gum-based drug delivery systems. Pharmaceutics 15 (1), 108. doi:10.3390/pharmaceutics15010108
Gajra, B., Pandya, S. S., Singh, S., and Rabari, H. A. (2014). Mucoadhesive hydrogel films of econazole nitrate: formulation and optimization using factorial design. J. drug Deliv. 2014, 1–14. doi:10.1155/2014/305863
Gao, W., Zhang, Y., Zhang, Q., and Zhang, L. (2016). Nanoparticle-hydrogel: a hybrid biomaterial system for localized drug delivery. Ann. Biomed. Eng. 44, 2049–2061. doi:10.1007/s10439-016-1583-9
Garti, N., and Reichman, D. (1993). Hydrocolloids as food emulsifiers and stabilizers. Food Struct. 12 (4), 3. doi:10.1016/B978-0-12-816685-7.00013-6
Gavlighi, H. A., Meyer, A. S., Zaidel, D. N. A., Mohammadifar, M. A., and Mikkelsen, J. D. (2013a). Stabilization of emulsions by gum tragacanth (Astragalus spp.) correlates to the galacturonic acid content and methoxylation degree of the gum. Food Hydrocoll. 31, 05–14. doi:10.1016/j.foodhyd.2012.09.004
Gavlighi, H. A., Michalak, M., Meyer, A. S., and Mikkelsen, J. D. (2013b). Enzymatic depolymerization of gum tragacanth: bifidogenic potential of low molecular weight oligosaccharides. J. Agric. Food Chem. 61, 1272–1278. doi:10.1021/jf304795f
Ghayempour, S., Montazer, M., and Mahmoudi Rad, M. (2015). Tragacanth gum as a natural polymeric wall for producing antimicrobial nanocapsules loaded with plant extract. Int. J. Biol. Macromol. 81, 514–520. doi:10.1016/j.ijbiomac.2015.08.041
Ghorbani, M., Ramezani, S., and Rashidi, M. R. (2021). Fabrication of honey-loaded ethylcellulose/gum tragacanth nanofibers as an effective antibacterial wound dressing. Colloids Surfaces a Physicochem. Eng. Aspects 621, 126615. doi:10.1016/j.colsurfa.2021.126615
Gieroba, B., Kalisz, G., Krysa, M., Khalavka, M., and Przekora, A. (2023). Application of vibrational spectroscopic techniques in the study of the natural polysaccharides and their cross-linking process. Int. J. Mol. Sci. 24 (3), 2630. doi:10.3390/ijms24032630
Größl, M., Harrison, S., Kaml, I., and Kenndler, E. (2005). Characterisation of natural polysaccharides (plant gums) used as binding media for artistic and historic works by capillary zone electrophoresis. J. Chromatogr. A 1077 (1), 80–89. doi:10.1016/j.chroma.2005.04.075
Gupta, A. P., and Verma, D. K. (2015). Synthesis and characterization of carboxymethyl guar gum nanoparticles stabilized polyaniline/carboxymethyl guar gum nanocomposites. J. Nanostructure Chem. 5 (4), 405–412. doi:10.1007/s40097-015-0172-z
Gupta, M., and Chatterjee, S. (2018). Quantified differentiation of surface topography for nano-materials as-obtained from atomic force microscopy images. J. Mater. Eng. Perform. 27 (6), 2734–2740. doi:10.1007/s11665-018-3360-4
Hagiwara, A., Boonyaphiphat, P., Kawabe, M., Naito, H., Shirai, T., and Ito, N. (1992). Lack of carcinogenicity of tragacanth gum in B6C3F1 mice. Food Chem. Toxicol. 30, 673–679. doi:10.1016/0278-6915(92)90162-e
Hama, R., Reinhardt, J. W., Ulziibayar, A., Watanabe, T., Kelly, J., and Shinoka, T. (2023). Recent tissue engineering approaches to mimicking the extracellular matrix structure for skin regeneration. Biomimetics 8 (1), 130. doi:10.3390/biomimetics8010130
He, J. J., McCarthy, C., and Camci-Unal, G. (2021). Development of hydrogel-based sprayable wound dressings for second-and third-degree burns. Adv. NanoBiomed Res. 1 (6), 2100004. doi:10.1002/anbr.202100004
Hemmatgir, F., Koupaei, N., and Poorazizi, E. (2022). Characterization of a novel semi-interpenetrating hydrogel network fabricated by polyethylene glycol diacrylate/polyvinyl alcohol/tragacanth gum as a wound dressing. Burns 48 (1), 146–155. doi:10.1016/j.burns.2021.04.025
Hemmati, K., and Ghaemy, M. (2016). Synthesis of new thermo/pH sensitive drug delivery systems based on tragacanth gum polysaccharide. Int. J. Biol. Macromol. 87, 415–425. doi:10.1016/j.ijbiomac.2016.03.005
Hemmati, K., Masoumi, A., and Ghaemy, M. (2015). Synthesis and characterization of pH-responsive nanohydrogels as biocompatible drug carriers based on chemically modified tragacanth gum polysaccharide. RSC Adv. 5, 85310–85318. doi:10.1039/c5ra14356j
Hemmati, K., Masoumi, A., and Ghaemy, M. (2016). Tragacanth gum-based nanogel as a superparamagnetic molecularly imprinted polymer for quercetin recognition and controlled release. CarbohydrPolym 136, 630–640. doi:10.1016/j.carbpol.2015.09.006
Heydary, H. A., Karamian, E., Poorazizi, E., Heydaripour, J., and Khandan, A. (2015). Electrospun of polymer/bioceramic nanocomposite as a new soft tissue for biomedical applications. J. Asian Ceram. Soc. 3 (4), 417–425. doi:10.1016/j.jascer.2015.09.003
Homayun, B., Lin, X., and Choi, H. J. (2019). Challenges and recent progress in oral drug delivery systems for biopharmaceuticals. Pharmaceutics 11 (3), 129. doi:10.3390/pharmaceutics11030129
Hosseini, M. S., Hemmati, K., and Ghaemy, M. (2016). Synthesis of nanohydrogels based on tragacanth gum biopolymer and investigation of swelling and drug delivery. Int. J. BiolMacromol 82, 806–815. doi:10.1016/j.ijbiomac.2015.09.067
Imeson, A. P. (1992). “Exudate gums,” in Thickening and gelling agents for food. Editor A. P. Imeson (London: Springer Science and Business Media), 66–97.
Jafari, S., Hojjati, M., and Noshad, M. (2018). Influence of soluble soybean polysaccharide and tragacanth gum based edible coating to improve the quality of fresh-cut apple slices. J. Food Process. Pres. 42 (6), 13638. doi:10.1111/jfpp.13638
Jalababu, R., Veni, S. S., and Reddy, K. S. (2018). Synthesis and characterization of dual responsive sodium alginate-g-acryloyl phenylalanine-poly N-isopropyl acrylamide smart hydrogels for the controlled release of anticancer drug. J. Drug Deliv. Sci. Technol. 44, 190–204. doi:10.1016/j.jddst.2017.12.013
Jones, L. O., Williams, L., Boam, T., Kalmet, M., Oguike, C., and Hatton, F. L. (2021). Cryogels: recent applications in 3D-bioprinting, injectable cryogels, drug delivery, and wound healing. Beilstein J. Org. Chem. 17 (1), 2553–2569. doi:10.3762/bjoc.17.171
Joshi, J., Homburg, S. V., and Ehrmann, A. (2022). Atomic force microscopy (AFM) on biopolymers and hydrogels for biotechnological applications—possibilities and limits. Polymers 14 (6), 1267. doi:10.3390/polym14061267
Kaiser, N., Kimpfler, A., Massing, U., Burger, A. M., Fiebig, H. H., Brandl, M., et al. (2003). 5-Fluorouracil in vesicular phospholipid gels for anticancer treatment: entrapment and release properties. Int. J. Pharm. 256 (1–2), 123–131. doi:10.1016/s0378-5173(03)00069-3
Kaith, B. S., Jindal, R., and Kumar, V. (2015). Biodegradation of Gum tragacanth acrylic acid based hydrogel and its impact on soil fertility. Polym. Degrad. Stab. 115, 24–31. doi:10.1016/j.polymdegradstab.2015.02.009
Kandar, C. C., Hasnain, M. S., and Nayak, A. K. (2021). “Natural polymers as useful pharmaceutical excipients,” in Advances and challenges in pharmaceutical technology (United States: Academic Press), 1–44.
Kargozar, S., Mozafari, M., Hamzehlou, S., Brouki Milan, P., Kim, H.-W., and Baino, F. (2019). Bone tissue engineering using human cells: a comprehensive review on recent trends, current prospects, and recommendations. Appl. Sci. 9, 174. doi:10.3390/app9010174
Khoylou, F., and Naimian, F. (2009). Radiation synthesis of superabsorbent polyethylene oxide/tragacanth hydrogel. RadiationPhysics Chem. 78 (3), 195–198. doi:10.1016/j.radphyschem.2008.11.008
Khushbu, S. G. W., Warkar, S. G., and Kumar, A. (2019). Synthesis and assessment of carboxymethyl tamarind kernel gum based novel superabsorbent hydrogels for agricultural applications. Polymer 182, 121823. Article ID 121823. doi:10.1016/j.polymer.2019.121823
Kiani, A., Shahbazi, M., and Asempour, H. (2012). Hydrogel membranes based on gum tragacanth with tunable structure and properties. I. Preparation method using Taguchi experimental design. J. Appl. Polym. Sci. 124 (1), 99–108. doi:10.1002/app.35038
Kohajdová, Z., and Karovičová, H. (2009). Application of hydrocolloids as baking improvers. Chem. Pap. 63 (1), 26–38. doi:10.2478/s11696-008-0085-0
Koosha, M., Solouk, A., Ghalei, S., Sadeghi, D., Bagheri, S., and Mirzadeh, H. (2019). Chitosan/gum tragacanth/PVA hybrid nanofibrous scaffold for tissue engineering applications. Bioinspired, Biomim. Nanobiomaterials 9 (1), 16–23. doi:10.1680/jbibn.18.00028
Kora, A. J., and Arunachalam, J. (2012). Green fabrication of silver nanoparticles by gum Tragacanth (Astragalus gummifer): a dual functional reductant and stabilizer. J. Nanomater. 2012, 1–8. doi:10.1155/2012/869765
Kora, A. J., and Arunachalam, J. (2012). Green fabrication of silver nanoparticles by gum tragacanth (Astragalus gummifer): a dual functional reductant and stabilizer. J. Nanomater. 2012, 1–8. doi:10.1155/2012/869765
Koyyada, A., and Orsu, P. (2021). Natural gum polysaccharides as efficient tissue engineering and drug delivery biopolymers. J. Drug Deliv. Sci. Technol. 63, 102431. doi:10.1016/j.jddst.2021.102431
Kulanthaivel, S., Agarwal, T., Pradhan, S., Pal, K., Giri, S., Maiti, T. K., et al. (2017a). Gum tragacanth–alginate beads as proangiogenic–osteogenic cell encapsulation systems for bone tissue engineering. J. Mater. Chem. B 5 (22), 4177–4189. doi:10.1039/c7tb00390k
Kulkarni, N., Rao, P., Jadhav, G. S., Kulkarni, B., Kanakavalli, N., Kirad, S., et al. (2023). Emerging role of injectable dipeptide hydrogels in biomedical applications. ACS omega 8 (4), 3551–3570. doi:10.1021/acsomega.2c05601
Kulkarni, V. S., and Shaw, C. (2016). “Use of polymers and thickeners in semisolid and liquid formulations,” in Essential chemistry for formulators of semisolid and liquid dosages (London: Academic Press), 43–69.
Kumar, S., Haq, I., Prakash, J., and Raj, A. (2017). Improved enzyme properties upon glutaraldehyde cross-linking of alginate entrapped xylanase from Bacillus licheniformis. Int. J. Biol. Macromol. 98, 24–33. doi:10.1016/j.ijbiomac.2017.01.104
Kurt, A. (2018). Physicochemical, rheological and structural characteristics of alcohol precipitated fraction of gum tragacanth. Food health 4 (3), 183–193. doi:10.3153/fh18019
Lapasin, R., and Pricl, S. (1999). Rheology of polysaccharide systems Rheology of industrial polysaccharides: theory and applications. United States: United States of America: An Aspen Publication. (250–309).
Levy, G., and Schwarz, T. W. (1958). Tragacanth solutions I. J. Am. Pharm. Assoc. 47 (6), 451–454. doi:10.1002/jps.3030470620
Li, J., and Mooney, D. J. (2016). Designing hydrogels for controlled drug delivery. Nat. Rev. Mater. 1 (12), 16071–16117. doi:10.1038/natrevmats.2016.71
Li, Q., Zhu, L., Liu, R., Huang, D., Jin, X., Che, N., et al. (2012). Biological stimuli responsive drug carriers based on keratin for triggerable drug delivery. J. Mat. Chem. 22, 19964–19973. doi:10.1039/c2jm34136k
Liu, J., Zhang, C., Miao, D., Sui, S., Deng, F., Dong, C., et al. (2018). Preparation and characterization of carboxymethylcellulose hydrogel fibers. Journalof Eng. Fibers Fabr. 13 (3), 155892501801300–13. doi:10.1177/155892501801300302
López-Cebral, R., Civantos, A., Ramos, V., Seijo, B., López-Lacomba, J. L., Sanz-Casado, J. V., et al. (2017). Gellan gum based physical hydrogels incorporating highly valuable endogen molecules and associating bmp-2 as bone formation platforms. Carbohydr. Polym. 167, 345–355. doi:10.1016/j.carbpol.2017.03.049
Lou, C., Tian, X., Deng, H., Wang, Y., and Jiang, X. (2020). Dialdehyde- β-cyclodextrin-cross-linked carboxymethyl chitosan hydrogel for drug release. Carbohydr. Polym. 231, 115678. Article ID 115678. doi:10.1016/j.carbpol.2019.115678
L´opez-Castej´on, M. L., Bengoechea, C., Garc´ıa-Morales, M., and Mart´ınez, I. (2016). Influence of tragacanth gum in egg white based bioplastics: thermomechanical and water uptake properties. Carbohydr. Polym. 152, 62–69. doi:10.1016/j.carbpol.2016.06.041
Maity, T., Saxena, A., and Raju, P. S. (2018). Use of hydrocolloids as cryoprotectant for frozen foods. Crit. Rev. Food Sci. Nutr. 58 (3), 420–435. doi:10.1080/10408398.2016.1182892
Makadia, H. K., and Siegel, S. J. (2011). Poly lactic-co-glycolic acid (PLGA) as biodegradable controlled drug delivery carrier. Polymers 3 (3), 1377–1397. doi:10.3390/polym3031377
Mallakpour, S., Tabesh, F., and Hussain, C. M. (2022). Potential of tragacanth gum in the industries: a short journey from past to the future. Polym. Bull. 80, 4643–4662. doi:10.1007/s00289-022-04284-1
Mallikarjuna Reddy, K., Ramesh Babu, V., Krishna Rao, K. S. V., Subha, M. C. S., Chowdoji Rao, K., Sairam, M., et al. (2008). Temperature sensitive semi-IPN microspheres from sodium alginate and N-isopropylacrylamide for controlled release of 5-fluorouracil. J. Appl. Polym. Sci. 107 (5), 2820–2829. doi:10.1002/app.27305
Malviya, R., Srivastava, P., and Kulkarni, G. (2011). Applications of mucilages in drug delivery-A review. Adv. Biol. Res. 5, 1–7.
Mantha, S., Pillai, S., Khayambashi, P., Upadhyay, A., Zhang, Y., Tao, O., et al. (2019). Smart hydrogels in tissue engineering and regenerative medicine. Materials 12 (20), 3323. doi:10.3390/ma12203323
Masoumi, A., and Ghaemy, M. (2014). Removal of metal ions from water using nanohydrogel tragacanth gum-g-polyamidoxime: isotherm and kinetic study. CarbohydPolym 108, 206–215. doi:10.1016/j.carbpol.2014.02.083
Meghdadi, K. R., and Boddohi, S. (2019). Preparation and investigation of gum tragacanth/gelatin nanofibers for antibacterial drug delivery systems. Iran. J. Polym. Sci. Technol. 32, 145–155. doi:10.22063/JIPST.2019.1650
Mersch-Sundermann, V., Knasmüller, S., Wu, X. J., Darroudi, F., Kassie, F., et al. (2004). Use of a humanderived liver cell line for the detection of cytoprotective, antigenotoxic and cogenotoxic agents. Toxicol. 198 (1-3), 329–340.
Moghbel, A., Agheli, H., Kalantari, E., and Naji, M. (2008). Design and formulation of tragacanth dressing bandage for burn healing with no dermal toxicity. Toxicol. Lett. 180, S154. doi:10.1016/j.toxlet.2008.06.341
Moghbel, A., Hemmati, A. A., Agheli, H., Amraee, K. H., and Rashidi, I. (2005). The effect of tragacanth mucilage on the healing of full-thickness wound in rabbit. Archives Iran. Med. 8, 257–262.
Mohamadisodkouieh, S., and Kalantari, M. (2022). Synthesis of a new hydrogel based on tragacanth gum with emphasis on its agricultural potential. J. Polym. Environ., 2022. doi:10.21203/rs.3.rs-1719427/v1
Mohammadinejad, R., Kumar, A., Ranjbar-Mohammadi, M., Ashrafizadeh, M., Han, S. S., Khang, G., et al. (2020). Recent advances in natural gum-based biomaterials for tissue engineering and regenerative medicine: a review. Polymers 12 (1), 176. doi:10.3390/polym12010176
Mohammadinejad, R., Maleki, H., Larraneta, E., Fajardo, A. R., Nik, A. B., Shavandi, A., et al. (2019). Status and future scope of plant-based green hydrogels in biomedical engineering. Appl. Mater. Today 16, 213–246. doi:10.1016/j.apmt.2019.04.010
Monjezi, J., Jamaledin, R., Ghaemy, M., and Makvandi, P. (2019). Antimicrobial modified-tragacanth gum/acrylic acid hydrogels for the controlled release of quercetin. KarajBranch J. Appl. Chem. Res. 13, 57–71.
Monjezi, J., Jamaledin, R., Ghaemy, M., Moeini, A., and Makvandi, P. (2018). A performance comparison of graft copolymer hydrogels based on functionalized-tragacanth gum/polyacrylic acid and polyacrylamide as antibacterial and antifungal drug release vehicles. Am. J. Nanotechnol. Nanomedicine Res. 1 (1), 010–015.
Nagaraja, K., Rao, K. M., Reddy, G. V., and Rao, K. K. (2021). Tragacanth gum-based multifunctional hydrogels and green synthesis of their silver nanocomposites for drug delivery and inactivation of multidrug resistant bacteria. Int. J. Biol. Macromol. 174, 502–511. doi:10.1016/j.ijbiomac.2021.01.203
Najafian, S., Eskandani, M., Derakhshankhah, H., Jaymand, M., and Massoumi, B. (2023). Extracellular matrix-mimetic electrically conductive nanofibrous scaffolds based on polyaniline-grafted tragacanth gum and poly (vinyl alcohol) for skin tissue engineering application. Int. J. Biol. Macromol. 249, 126041. doi:10.1016/j.ijbiomac.2023.126041
Nasiri, M., Barzegar, M., Sahari, M. A., and Niakousari, M. (2017). Tragacanth gum containing Zataria multiflora Boiss. Essential oil as a natural preservative for storage of button mushrooms (Agaricus bisporus). Food Hydrocoll. 72, 202–209. doi:10.1016/j.foodhyd.2017.05.045
Nasiri, M., Barzegar, M., Sahari, M. A., and Niakousari, M. (2018). Application of Tragacanth gum impregnated with Satureja khuzistanica essential oil as a natural coating for enhancement of postharvest quality and shelf life of button mushroom (Agaricus bisporus). Int. J. Biol. Macromol. 106, 218–226. doi:10.1016/j.ijbiomac.2017.08.003
Nasution, H., Harahap, H., Dalimunthe, N. F., Ginting, M. H. S., Jaafar, M., Tan, O. O., et al. (2022). Hydrogel and effects of crosslinking agent on cellulose-based hydrogels: a review. Gels 8 (9), 568. doi:10.3390/gels8090568
Nejatian, M., Abbasi, S., and Azarikia, F. (2020a). Gum Tragacanth: structure, characteristics and applications in foods. Int. J. Biol. Macromol. 160, 846–860. doi:10.1016/j.ijbiomac.2020.05.214
Ngwabebhoh, F. A., Gazi, M., and Oladipo, A. A. (2016). Adsorptive removal of multi-azo dye from aqueous phase using a semi- IPN superabsorbent chitosan-starch hydrogel. Chem. Eng. Res. Des. 112, 274–288. doi:10.1016/j.cherd.2016.06.023
Nieto, M. B. (2009). “Structure and function of polysaccharide gum-based edible films and coatings,” in Edible films and coatings for food applications. Editors K. C. Huber, and M. E. Embuscado (New York: Springer), 57–112.
Nishinari, K., and Zhang, H. (2004). Recent advances in the understanding of heat set gelling polysaccharides. Trends Food Sci. Technol. 15 (6), 305–312. doi:10.1016/j.tifs.2003.05.001
Nur, M., Ramchandran, L., and Vasiljevic, T. (2016). Tragacanth as an oral peptide and protein delivery carrier: characterization and mucoadhesion. Carbohydr. Polym. 143, 223–230. doi:10.1016/j.carbpol.2016.01.074
Nussinovitch, A. (2009). Plant gum exudates of the world: sources, distribution, properties, and applications. Florida: CRC Press.
Omidian, H., and Chowdhury, S. D. (2023). Advancements and applications of injectable hydrogel composites in biomedical research and therapy. Gels 9 (7), 533. doi:10.3390/gels9070533
Panuncialman, J., and Falanga, V. (2009). The science of wound bed preparation. Surg. Clin. N. Am. 89 (3), 611–626. doi:10.1016/j.suc.2009.03.009
Pareek, A., Maheshwari, S., Cherlo, S., Thavva, R. S. R., and Runkana, V. (2017). Modeling drug release through stimuli responsive polymer hydrogels. Int. J. Pharm. 532 (1), 502–510. doi:10.1016/j.ijpharm.2017.09.001
Pathania, D., Verma, C., Negi, P., Tyagi, I., Asif, M., Kumar, N. S., et al. (2018). Novel nanohydrogel based on itaconic acid grafted tragacanth gum for controlled release of. Ampicillin. CarbohydrPolym 196, 262–271. doi:10.1016/j.carbpol.2018.05.040
Peng, X., and Yao, Y. (2017). Carbohydrates as fat replacers. Annu. Rev. Food Sci. Throughput 8 (1), 331–351. doi:10.1146/annurev-food-030216-030034
Phillips, G. O., and Williams, P. A. (2009). Handbook of hydrocolloids. 2nd Edition. Cambridge: Woodhead Publishing.
Polez, R. T., Morits, M., Jonkergouw, C., Phiri, J., Valle-Delgado, J. J., Linder, M. B., et al. (2022). Biological activity of multicomponent bio-hydrogels loaded with tragacanth gum. Int. J. Biol. Macromol. 215, 691–704. doi:10.1016/j.ijbiomac.2022.06.153
Potaś, J., Szymańska, E., Basa, A., Hafner, A., and Winnicka, K. (2020). Tragacanth gum/chitosan polyelectrolyte complexes-based hydrogels enriched with xanthan gum as promising materials for buccal application. Materials 14 (1), 86. doi:10.3390/ma14010086
Pourmolaie, H., Khosrowshahi Asl, A., Ahmadi, M., Zomorodi, S., and Naghizadeh Raeisi, S. (2018). The effect of Guar and Tragacanth gums as edible coatings in Cheddar cheese during ripening. J. Food Saf. 38 (6), e12529. doi:10.1111/jfs.12529
Rakkappan, C., and Anbalagan, S. (2009). Ultrasonic and FTIR studies on aqueous biodegradable polymer blend solutions. Am. Eurasia J. Sci. Res. 4, 281–284.
Ranjbar-Mohammadi, M., and Bahrami, S. H. (2015). Development of nanofibrous scaffolds containing gum tragacanth/poly (ε-caprolactone) for application as skin scaffolds. Mater. Sci. Eng. C 48, 71–79. doi:10.1016/j.msec.2014.10.020
Ranjbar-Mohammadi, M., Bahrami, S. H., and Joghataei, M. T. (2013). Fabrication of novel nanofiber scaffolds from gum tragacanth/poly (vinyl alcohol) for wound dressing application: in vitro evaluation and antibacterial properties. Mater. Sci. Eng. C 33 (8), 4935–4943. doi:10.1016/j.msec.2013.08.016
Ranjbar Mohammadi, M., Kargozar, S., Bahrami, S. H., and Rabbani, S. (2020). An excellent nanofibrous matrix based on gum tragacanth-poly (Ɛ-caprolactone)-poly (vinyl alcohol) for application in diabetic wound healing. Polym. Degrad. Stab. 174, 109105. doi:10.1016/j.polymdegradstab.2020.109105
Ranjbar-Mohammadi, M., Prabhakaran, M. P., Bahrami, S. H., and Ramakrishna, S. (2016). Gum tragacanth/poly (l-lactic acid) nanofibrous scaffolds for application in regeneration of peripheral nerve damage. Carbohydr. Polym. 140, 104–112. doi:10.1016/j.carbpol.2015.12.012
Rao, K., Imran, M., Jabri, T., Ali, I., Perveen, S., Ahmed, S., et al. (2017). Gum tragacanth stabilized green gold nanoparticles as cargos for Naringin loading: a morphological investigation through AFM. Carbohydr. Polym. 174, 243–252. doi:10.1016/j.carbpol.2017.06.071
Robert, G. F., and Jaminelli, B. (2015). Challenges in the treatment of chronic wounds. Adv. wound care 4 (9), 560–582. doi:10.1089/wound.2015.0635
Saha, D., and Bhattacharya, S. (2010). Hydrocolloids as thickening and gelling agents in food: a critical review. J. Food Sci. Tech. 47 (6), 587–597. doi:10.1007/s13197-010-0162-6
Saruchi, K. B. S., Jindal, R., and Kapur, G. S. (2013). Enzyme-based green approach for the synthesis of gum tragacanth and acrylic acid cross-linked hydrogel: its utilization in controlled fertilizer release and enhancement of water-holding capacity of soil. Iran. Polym. J. 22, 561–570. doi:10.1007/s13726-013-0155-1
SaruchiKaith, B. S., Jindal, R., and Kumar, V. (2015). Biodegradation of Gum tragacanth acrylic acid based hydrogel and its impact on soil fertility. Polymdegr. Stabil. 115, 24–31. doi:10.1016/j.polymdegradstab.2015.02.009
Sawai, R., Kuroda, K., Shibata, T., Gomyou, R., Osawa, K., and Shimizu, K. (2008). Anti-influenza virus activity of Chaenomelessinensis. J. Ethnopharmacol. 118 (1), 108–112. doi:10.1016/j.jep.2008.03.013
Schiffman, J. D., and Schauer, C. L. (2013). A review: electrospinning of biopolymer nanofibers and their applications. PolymerReviews 48 (2), 317–352. doi:10.1080/15583720802022182
Seidi, K., Ayoubi-Joshaghani, M. H., Azizi, M., Javaheri, T., Jaymand, M., Alizadeh, E., et al. (2021). Bioinspired hydrogels build a bridge from bench to bedside. Nano Today 39, 101157. doi:10.1016/j.nantod.2021.101157
Shaikh, R., Singh, T. R. R., Garland, M. J., Woolfson, A. D., and Donnelly, R. F. (2011). Mucoadhesive drug delivery systems. J. Pharm. Bioallied Sci. 3 (1), 89. doi:10.4103/0975-7406.76478
Sharifi, Z., JebelliJavan, A., Hesarinejad, M. A., and Parsaeimehr, M. (2023). Application of carrot waste extract and Lactobacillus plantarum in Alyssum homalocarpum seed gum-alginate beads to create a functional synbiotic yogurt. Chem. Biol. Technol. Agric. 10 (1), 3. doi:10.1186/s40538-022-00377-1
Shirazi, N. M., Eslahi, N., and Gholipour-Kanani, A. (2021). Production and characterization of Keratin/Tragacanth gum nanohydrogels for drug delivery in medical textiles. Front. Mat. 8. Article 720385. doi:10.3389/fmats.2021.720385
Singh, B., Sharma, K., and Dutt, S. (2020). Dietary fiber tragacanth gum based hydrogels for use in drug delivery applications. Bioact. Carbohydrates Diet. Fibre 21, 100208. doi:10.1016/j.bcdf.2019.100208
Singh, B., and Sharma, V. (2014). Influence of polymer network parameters of tragacanth gum-based pH responsive hydrogels on drug delivery. CarbohydrPolym 101, 928–940. doi:10.1016/j.carbpol.2013.10.022
Singh, B., and Singh, J., (2021). Application of tragacanth gum and alginate in hydrogel wound dressing’s formation using gamma radiation. Carbohydr. Polym. Technol. Appl. 2, 100058. doi:10.1016/j.carpta.2021.100058
Singh, B., Varshney, L., Francis, S., and Rajneesh, H. (2016). Designing tragacanth gum based sterile hydrogel by radiation method for use in drug delivery and wound dressing applications. Int. J. Biol. Macromol. 88, 586–602. doi:10.1016/j.ijbiomac.2016.03.051
Singh, B., Varshney, L., Francis, S., and Rajneesh, H. (2017a). Synthesis and characterization of tragacanth gum based hydrogels by radiation method for use in wound dressing application. Radiat. Phys. Chem. 135, 94–105. doi:10.1016/j.radphyschem.2017.01.044
Singh, S., Young, A., and McNaught, C. E. (2017b). The physiology of wound healing. Surg. Oxf. 35 (9), 473–477. doi:10.1016/j.mpsur.2017.06.004
Smagul, S., Kim, Y., Smagulova, A., Raziyeva, K., Nurkesh, A., and Saparov, A. (2020). Biomaterials loaded with growth factors/cytokines and stem cells for cardiac tissue regeneration. Int. J. Mol. Sci. 21 (17), 5952. doi:10.3390/ijms21175952
Sodkouieh, S. M., and Kalantari, M. (2022). Synthesis of a new hydrogel based on tragacanth gum with emphasis on its agricultural potential.
Stojkov, G., Niyazov, Z., Picchioni, F., and Bose, R. K. (2021). Relationship between structure and rheology of hydrogels for various applications. Gels 7 (4), 255. doi:10.3390/gels7040255
Taghavizadeh Yazdi, M. E., Nazarnezhad, S., Mousavi, S. H., Sadegh Amiri, M., Darroudi, M., Baino, F., et al. (2021b). Gum tragacanth (GT): a versatile biocompatible material beyond borders. Molecules 26, 1510. doi:10.3390/molecules26061510
Taghavizadeh Yazdi, M. E., Nazarnezhad, S., Mousavi, S. H., Sadegh Amiri, M., Darroudi, M., Baino, F., et al. (2021a). Gum tragacanth (GT): a versatile biocompatible material beyond borders. Molecules 26 (6), 1510. doi:10.3390/molecules26061510
Tessarolli, F. G., Souza, S. T., Gomes, A. S., and Mansur, C. R. (2019). Influence of polymer structure on the gelation kinetics and gel strength of acrylamide-based copolymers, bentonite and polyethylenimine systems for conformance control of oil reservoirs. J. Appl. Polym. Sci. 136 (22), 47556. doi:10.1002/app.47556
Tischer, C. A., Iacomini, M., and Gorin, P. A. (2002). Structure of the arabinogalactan from gum tragacanth (Astralagus gummifer). Carbohydr. Res. 337 (18), 1647–1655. doi:10.1016/s0008-6215(02)00023-x
Tonyali, B., Cikrikci, S., and Oztop, M. H. (2018). Physicochemical and microstructural characterization of gum tragacanth added whey protein based films. Food Res. Int. 105, 1–9. doi:10.1016/j.foodres.2017.10.071
Torres, M. D., Moreira, R., Chenlo, F., and V´azquez, M. J. (2012). Water adsorption isotherms of carboxymethyl cellulose, guar, locust bean, tragacanth and xanthan gums. CarbohydratePolymers 89 (2), 592–598. doi:10.1016/j.carbpol.2012.03.055
Tottoli, E. M., Dorati, R., Genta, I., Chiesa, E., Pisani, S., and Conti, B. (2020). Skin wound healing process and new emerging technologies for skin wound care and regeneration. Pharmaceutics 12 (8), 735. doi:10.3390/pharmaceutics12080735
Valencia, G. A., Zare, E. N., Makvandi, P., and Gutiérrez, T. J. (2019). Self-assembled carbohydrate polymers for food applications: a review. Compr. Rev. Food Sci. Food Saf. 18 (6), 2009–2024. doi:10.1111/1541-4337.12499
Verbeken, D., Dierckx, S., and Dewettinck, K. (2003). Exudate gums: occurrence, production, and applications. Appl. Microbiol. Biotechnol. 63, 10–21. doi:10.1007/s00253-003-1354-z
Vigata, M., Meinert, C., Hutmacher, D. W., and Bock, N. (2020). Hydrogels as drug delivery systems: a review of current characterization and evaluation techniques. Pharmaceutics 12 (12), 1188. doi:10.3390/pharmaceutics12121188
Wang, B., Xu, X. D., Wang, Z. C., Cheng, S. X., Zhang, X. Z., and Zhuo, R. X. (2008). Synthesis and properties of pH and temperature sensitive P (NIPAAm-co-DMAEMA) hydrogels. Colloids Surfaces B Biointerfaces 64 (1), 34–41. doi:10.1016/j.colsurfb.2008.01.001
Wang, Y., Xie, Z., Wu, Q., Song, W., Liu, L., Wu, Y., et al. (2020). Preparation and characterization of carboxymethyl starch from cadmium-contaminated rice. Food Chem. 308, 125674. Article ID 125674. doi:10.1016/j.foodchem.2019.125674
Weiping, W. (2000). “Tragacanth and karaya,” in Handbook of hydrocolloids. Editors G. O. Philips, and P. A. Williams (Cambridge, England: CRC Press), 231–246.
Weiping, W., Phillips, G., and Williams, P. (2000). Tragacanth and karaya. Cambridge: Woodhead Publishing Ltd.
Weller, C., and Sussman, G. (2006). Wound dressings update. J. Pharm. Pract. Res. 36 (4), 318–324. doi:10.1002/j.2055-2335.2006.tb00640.x
Whistler, R. L. (1993). “Exudate gums,” in Industrial gums, polysaccharides and their derivatives. Editors R. L. Whistler, and J. N. BeMiller (San Diego: Academic Press), 309e339.
White, R. J., and Cutting, K. F. (2003). Interventions to avoid maceration of the skin and wound bed. Br. J. Nurs. 12 (20), 1186–1201. doi:10.12968/bjon.2003.12.20.11841
Xia, Y., Ma, Z., Wu, X., Wei, H., Zhang, H., Li, G., et al. (2023). Advances in stimuli-responsive chitosan hydrogels for drug delivery systems. Macromol. Biosci., 2300399. doi:10.1002/mabi.202300399
Xiang, J., Shen, L., and Hong, Y. (2020). Status and future scope of hydrogels in wound healing: synthesis, materials and evaluation. Eur. Polym. J. 130, 109609. doi:10.1016/j.eurpolymj.2020.109609
Xie, A., Zhu, C., and Hua, L. (2017). Food gum based hydrogel polymers. MOJ Food Process. Technol. 4, 192–196. doi:10.15406/mojfpt.2017.04.00112
Yazdi, M. E. T., Nazarnezhad, S., Mousavi, S. H., Amiri, M. S., Darroudi, M., Baino, F., et al. (2021). Gum tragacanth (GT): a versatile biocompatible material beyond borders. Mol. I 26 (1510), 1–18.
Yu, Y., Pan, H., Wang, Y., Xiong, W., Zhang, Q., Chen, K., et al. (2016). New insights into an innovative Auricularia auricular polysaccharide pH-sensitive hydrogel for controlled protein drug delivery. RSC Adv. 6 (64), 59794–59799. doi:10.1039/c6ra06463a
Zagórska-Dziok, M., and Sobczak, M. (2020). Hydrogel-based active substance release systems for cosmetology and dermatology application: a review. Pharmaceutics 12 (5), 396. doi:10.3390/pharmaceutics12050396
Zare, E. N., Makvandi, P., and Tay, F. R. (2019). Recent progress in the industrial and biomedical applications of tragacanth gum: a review. Carbohydr. Polym. 212, 450–467. doi:10.1016/j.carbpol.2019.02.076
Zare, E. N., Motahari, A., and Sillanpää, M. (2018). Nano adsorbents based on conducting polymer nanocomposites with main focus on polyaniline and its derivatives for removal of heavy metal ions/dyes: a review. Environ. Res. 162, 173–195. doi:10.1016/j.envres.2017.12.025
Zarekhalili, Z., Bahrami, S. H., Ranjbar-Mohammadi, M., and Milan, P. B. (2017). Fabrication and characterization of PVA/Gum tragacanth/PCL hybrid nanofibrous scaffolds for skin substitutes. Int. J. Biol. Macromol. 94, 679–690. doi:10.1016/j.ijbiomac.2016.10.042
Zhao, Y., Li, Z., Li, Q., Yang, L., Liu, H., Yan, R., et al. (2020). Transparent conductive supramolecular hydrogels with stimuli-responsive properties for on-demand dissolvable diabetic foot wound dressings. Macromol. Rapid Commun. 41 (24), 2000441. doi:10.1002/marc.202000441
Keywords: Tragacanth, hydrogels, tissue engineering, polysaccharides, drug delivery
Citation: Abdi G, Jain M, Patil N, Tariq M, Choudhary S, Kumar P, Raj NS, Mohsen Ali SS and Uthappa UT (2024) Tragacanth gum-based hydrogels for drug delivery and tissue engineering applications. Front. Mater. 11:1296399. doi: 10.3389/fmats.2024.1296399
Received: 18 September 2023; Accepted: 09 January 2024;
Published: 16 February 2024.
Edited by:
Arun Prabhu Rameshbabu, Harvard Medical School, United StatesReviewed by:
Didem Demir, Tarsus University, TürkiyeCopyright © 2024 Abdi, Jain, Patil, Tariq, Choudhary, Kumar, Raj, Mohsen Ali and Uthappa. This is an open-access article distributed under the terms of the Creative Commons Attribution License (CC BY). The use, distribution or reproduction in other forums is permitted, provided the original author(s) and the copyright owner(s) are credited and that the original publication in this journal is cited, in accordance with accepted academic practice. No use, distribution or reproduction is permitted which does not comply with these terms.
*Correspondence: Mukul Jain, am11a3VsODAxQGdtYWlsLmNvbQ==; Gholamreza Abdi, YWJkaUBwZ3UuYWMuaXI=
Disclaimer: All claims expressed in this article are solely those of the authors and do not necessarily represent those of their affiliated organizations, or those of the publisher, the editors and the reviewers. Any product that may be evaluated in this article or claim that may be made by its manufacturer is not guaranteed or endorsed by the publisher.
Research integrity at Frontiers
Learn more about the work of our research integrity team to safeguard the quality of each article we publish.