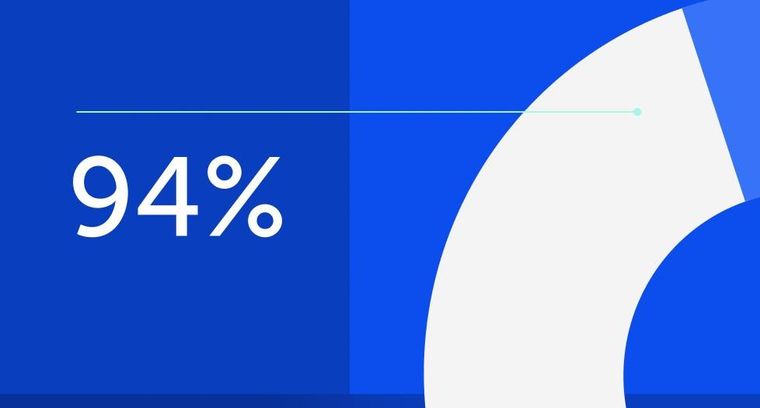
94% of researchers rate our articles as excellent or good
Learn more about the work of our research integrity team to safeguard the quality of each article we publish.
Find out more
ORIGINAL RESEARCH article
Front. Mater., 11 January 2024
Sec. Polymeric and Composite Materials
Volume 10 - 2023 | https://doi.org/10.3389/fmats.2023.1331308
This article is part of the Research TopicRecent Advances in Sustainable Polymer MaterialsView all 5 articles
Microplastic pollution is a growing concern, and natural materials are being increasingly sought as plastic alternatives. Semisynthetic biopolymers occupy a grey area between natural and synthetic materials and are often presented as green alternatives to conventional plastic. They can be water-soluble or insoluble, and are ubiquitous in commercial products as thickeners, films, filters, viscosity modifiers and coatings. This work compares the mineralization kinetics of cellulose, guar and several of their commercialized derivatives using a simple pseudo first-order kinetic model to extrapolate half-lives and lifetimes, while identifying the levers that influence the mineralization rates of these ubiquitous materials. Industrial composting rates were consistently faster than those of wastewater. While partially substituted biopolymers exhibited measurable degradation, kinetic analysis revealed this effect could be entirely accounted for by the fraction of unsubstituted biopolymer. Surprisingly, the initial rates of highly substituted biopolymers exhibited persistence on par with conventional plastics over the experimental durations studied.
The use of persistent chemicals and materials has contributed to environmental pollution (Li et al., 2016; Andrady, 2017), biocide resistance (Stewart et al., 2001; Mathur and Singh, 2005; Green, 2007; Green and Owen, 2011) and hazards such as hormone disruption, (Hoffman et al., 2017), cancer (Vaughn et al., 2013), and birth defects (Ngo et al., 2006). A principal goal of the bioeconomy is the conversion of biological resources into products that are competitive with those derived from nonrenewable sources, thereby reducing our reliance on “fossilized” carbon feedstocks (Philp et al., 2013; Bugge et al., 2016; Kim et al., 2016; Matharu et al., 2016; Buckley et al., 2017). Synthetic modification of naturally-derived biochemicals, including biomacromolecules, can produce semisynthetic materials with value-added properties that are not present in the parent biomolecule (Debeaufort et al., 1998).
However, while adding commercial viability, these synthetic modifications may also reduce the biodegradability of the resulting semisynthetic material by orders of magnitude (Wirick, 1974; Rivard et al., 1992; Buchanan et al., 1993; Puls et al., 2011). A primary example of this reduced degradability is cigarette filters which are made of cellulose acetate, a mostly natural biomacromolecule, that is a leading visible source of plastic pollution, especially in large bodies of water (Driedger et al., 2015).
Other common plastics such as polyvinyl alcohol, used to make laundry or dish pods, polyethylene, and polyethylene terephthalate (Morét et al., 2010), are also reportedly biodegradable under unique conditions, such as exposure to specific fungi, rare bacteria (Yoshida et al., 2016), or wax moth larvae (Bombelli et al., 2017). However, these examples illustrate a critical distinction between a material’s possible capacity for biodegradation versus the extent to which mineralization actually occurs in natural environments (Kint and Muñoz-Guerra, 1999; Kubowicz and Booth, 2017). Experimental determinations of biopolymer degradability are highly variable depending on the media, inoculum, and conditions employed (Puls et al., 2011), and natural environments generally do not mimic the conditions found in the lab. The incomplete breakdown of plastics generates microplastics that accumulate in the human body, foods, and the natural environment (Andrady, 2011). While previous studies have examined the thermochemical degradation of cellulose (Osman et al., 2022), and biodegradability of natural and semisynthetic celluloses (Kubowicz and Booth, 2017; Torgbo and Sukyai, 2020; Frank et al., 2021; Polman et al., 2021; Yadav and Hakkarainen, 2021), investigations comprehensively evaluating the effect of functional group, medium (e.g., wastewater, compost) and degree of substitution (DS) using standard degradation protocols are needed.
In this work, we compared the mineralization rates of semisynthetic polysaccharides to unmodified cellulose and guar in aerobic wastewater and soil compost environments (hydroxypropyl methylcellulose (HPMC), carboxymethyl cellulose (CMC), hydroxyethyl cellulose (HEC), ethyl cellulose (EC), cellulose acetate (CA), hydroxypropyl guar (HPG)); Figure 1). These substances were selected due to their widespread use and commercial viability. A respirometry method was utilized to measure the mineralization of the cellulosic materials in the wastewater and compost matrices. Pseudo first-order kinetic analysesenabled the quantitative determinaton of relative biodegradability rates, half-lives and predicted lifetimes. Similar approaches (Hart-Cooper et al., 2015a; Hart-Cooper et al., 2015b; Flynn et al., 2020), which have been used to quantify chemical reaction rates, enables rapid determination of biodegradation half-lives, and their extrapolation to material lifetimes, typically defined as five half-lives, or ∼97% conversion. The effect of functional group type (e.g., ether, ester, unmodified) on degradation was determined. A kinetic model was used to deconvolute how the degree of polymer substitution influenced degradation rate. Comprehensively, these metrics provide a facile, robust, and practical way to assess and characterize the biodegradability of semisynthetics. A kinetic framework for understanding the effects of functional group derivatization, degree of substitution and media is presented herein.
FIGURE 1. (A) Structures of celluloses and derivatives, (B) guar and hydroxypropyl guar, and (C) end of life schematic for waste celluloses.
Chemicals were acquired from commercial sources (Ashland: HEC, CMC; DuPont: EC, HPMC; Sigma Aldrich: CA; Rita Corp: HPG; Avicell: cellulose; Rhodia: Guar; Supplementary Table S1), with grades based on commercial availability. Molecular weight (MW) and degree of substitution (DS) are indicated in Table 1. Compost (Miracle-Gro Garden Soil All Purpose) was purchased from a local hardware store. The compost contained 0.09% total nitrogen, 0.05% available phosphate (P2O5), and 0.07% soluble potash (K2O). Moisture content of the compost was determined using a moisture analyzer (Ohaus Model MB25, Switzerland).
TABLE 1. Kinetic parameters, projected half-lives, and projected lifetimes of biopolymer mineralization.
Respiration rates obtained via monitoring CO2 concentrations using a respirometer (Micro-Oxymax, Columbus Instruments, Columbus, OH). Since there is a baseline amount of CO2 produced by the compost or wastewater, the CO2 production attributable to each treatment sample was determined by subtracting the baseline production (control) from the total CO2 production detected in the respirometry chambers containing the sample material being tested. A first order rate constant was found for each data set using an exponential fit and used to calculate projected half-lives and lifetimes.
The compost was prepared by sieving (14 mesh), adjusting the moisture content to 60% (w/w), and equilibrating overnight in a plastic bag. Polymer samples were prepared by milling to 40 mesh per ASTM D 5338 (ASTM D5338 et al., 2003). Glass respiration chambers (500 mL) were prepared by loading 40.0 g of compost and 0.500 g of milled sample, measured using an analytical balance. The contents of each respirometer chamber were thoroughly mixed before attaching to the respirometer via tubing. The respiration chambers were kept in an enclosed incubator (60°C ± 0.5°C) to maintain a stable temperature based on industrial composting standards. The experimental design included three replicates for each treatment. Four controls containing only compost were also included in each experiment for baseline determination. The net increase in CO2 production for each treatment was calculated. The standard deviations for individual time points were 5%–15%.
Respirometry determination in wastewater closely followed ISO 14851 (International Organization for Standardization, 2019). A standard test medium was prepared, composed from 10 mL solution A (8.5 g KH2PO4, 21.75 g K2HPO4, 33.4 g Na2HPO4×2H2O, 0.5 g NH4Cl; diluted to 1,000 mL), 1 mL each of solutions B (22.5 g MgSO4×7H2O; diluted to 1,000 mL), C (36.4 g CaCl2×2H2O; diluted to 1,000 mL), and D (0.25 g FeCl3×6H2O; diluted to 1,000 mL), and the resulting solution further diluted to a total volume of 1,000 mL with DI water. The activated sludge, obtained from East Bay Municipal Utilities District (Oakland, CA), was filtered through a 16-mesh sieve. Activated sludge (0.5 mL) with a solids content of 1.66% was added into each chamber to achieve a solids content of 830 mg dry weight. The milled sample (0.500 g) was added to the respirometer chambers and the contents were agitated before attaching them to the respirometer via tubing. Respirometry was carried out in an incubator maintained at 30°C (±0.5°C). CO2 accumulation in the headspace of each chamber was continuously recorded. Each sample was run in triplicate with four controls to measure the baseline CO2 production of the activated sludge. The net CO2 production for each sample was used to determine the mineralization rates.
The carbon content of each sample was calculated and used to determine the theoretical yield of CO2 if 100% of the sample carbon was mineralized. The percent mineralization of each sample was calculated by dividing the cumulative CO2 produced by the theoretical CO2 and multiplying by 100.
We compared the biodegradability of natural cellulose to commercial semisynthetic derivatives. Semisynthetic derivatives were selected based on commercial utility and exhibited varying degrees of substitution (DS). Biodegradation was monitored by measuring accumulated CO2 relative to a baseline that does not contain test material (Figure 2).
FIGURE 2. (A) Soil compost (60°C) and (B) aerobic wastewater (30°C) mineralization of cellulose, carboxymethyl cellulose (CMC), cellulose acetate (CA), and hydroxypropyl methylcellulose (HPMC).
The accumulation of CO2 was a direct measurement of the thermodynamic final product of biopolymer consumption and is a useful metric for assessing full biodegradation, as it measures only the final oxidation product of carbon. Like many methods to assess biodegradation, this approach is not a perfect model for envrironmental biodegradation, in that it does not include continual inputs of organic matter, and has a limited ability to determine which functionalities are being respired. Monitoring oxygen and starting material consumption alone does not account for the possibility that starting material could be consumed, yet not entirely mineralized to CO2. Plots of the percent mineralization versus time fit well to pseudo first-order kinetic models using Eq. 1 and shown in Figure 3):
FIGURE 3. Initial biodegradation rates (Eq. 1) for (A) cellulose, (B) carboxymethyl cellulose (CMC), and (C) cellulose acetate (CA) in compost (○) and wastewater (●). Note differences in scales.
Where [A] represents the concentration of a substance at a given time [A]0 is the starting concentration of the substance, kobs is the pseudo first-order rate constant and t represents time.
Determination of first-order rate constants (kobs) enabled the calculation of reaction half-lives and projected material lifetimes, which represent five half-lives (Figure 3; Table 1).
A perpetual challenge in biodegradation studies is the high variability observed between inoculum compositions. To address this issue in our measurements, we calculated relative mineralization rates, krel, which represents the ratio of a given biodegradation rate divided by the minimum rate observed in the experimental set, shown in Eq. 2. This value (krel) provides a robust comparison of an inoculum’s pseudo first-order biodegradation rate relative to a positive control (cellulose). In our hands, relative biodegradation rates spanned up to two orders of magnitude, indicating that HPMC, EC and HEC are at minimum 100 times more persistent than cellulose.
In soil compost (60°C), cellulose mineralized rapidly at an average rate of 3% per day for the first 23 days, with an extrapolated lifetime of 3 months. Carboxymethyl cellulose (CMC; DS = 0.7) degraded slightly slower, on average 1% per day (first 23 days) with a lifetime of 1 year. This projected lifetime is remarkably close to those reported in previous studies of CMC, DS = 0.7 (Nie et al., 2004). Cellulose acetate (DS = 3) mineralized an order of magnitude more slowly than the CMC sample (0.1% per day; lifetime of 8 years, assuming no induction period). Given the high DS of CA, this observed mineralization rate is lower than what is typically observed with less substituted analogues (Buchanan et al., 1993; Puls et al., 2011; Yadav and Hakkarainen, 2021). The remaining synthetically modified celluloses (HPMC; HEC; EC) did not measurably degrade. We attribute the higher degradability rate of CA compared to EC to CA’s ester bonds, which are more rapidly cleaved than an ethyl ether bond. This result led us to establish a maximum estimate of their biodegradation rates based on detection limits, which were comparable to the minimum measurable biodegradation rate observed in compost (kobs ≤ 0.001 days−1, lifetime of ≥10 years, assuming no induction period).
A polysaccharide’s degree of substitution (DS) represents, on average, how many hydroxyl moieties in each monomer are functionalized. High degrees of substitution are associated with lower degradation rates compared to the unmodified polymer, an effect that can be attributed to, for example, reduced enzyme affinity for the modified substrate, and bulk polymer properties, such as reduced water solubility or bioavailability. To determine whether the inhibiting effects of synthetic modification on mineralization are general to other polysaccharides, guar and hydroxypropyl guar (HPG; DS 1.3) were evaluated (Supplementary Figure S1). Guar mineralized at approximately twice the rate of cellulose and 22 times faster than HPG. Unlike HPMC, HEC, and EC, the mineralization of HPG was fast enough to be measured. We attribute this effect to the fact that a monomer of guar contains three sugar subunits: one galactose and two mannoses. DS = 1.3 implies that on average, at least one subunit of sugar would not be derivatized per guar monomer. The observed small levels of mineralization can be attributed to microbial consumption of unmodified galactose and mannose. Mineralization rates in soil compost decreased in the following order: guar > cellulose > CMC > HPG > CA > HPMC, HEC, EC.
A subset of cellulose derivatives representing a range of soil mineralization rates were additionally evaluated in wastewater (Table 1). Relatively low-temperature wastewater (30°C) mineralization rates were approximately an order of magnitude slower than in higher temperature soil compost (60°C) conditions, except for cellulose acetate, where the magnitude of the effect was smaller, krel ∼ 3; Figure 2B. Thermal effects on reaction rate are likely significant contributors for these differences. Within viable temperature ranges, most biological reaction rates increase by a factor of 2-3 with every 10°C increase in temperature (Reyes et al., 2008). The slower mineralization rate in wastewater may be attributed to the low microbial density and reduced diversity. Microbial strains present in compost will also be different than those in wastewater. All these factors impact the overall mineralization rate and can account for the observed differences between wastewater and compost conditions.
An apparent induction period of several days occurred for cellulose, suggesting microbial acclimation. Relative mineralization rates among different materials followed the same trend as observed in soil. As with compost, HPMC did not measurably mineralize to CO2 and was assigned a maximum estimate for its biodegradability rate constant based on detection limits (kobs ≤ 0.0004 days−1, lifetime of ≥24 years, assuming no induction period). Unlike soil compost, wastewater treatments included no additional carbon source aside from the test substance, allowing for an improved signal to noise ratio and a lower maximum rate estimate.
A probable biodegradation mechanism for substituted cellulose derivatives is depicted in Figure 4. A substituted fragment (1) undergoes slow deprotection (k1) to afford an unsubstituted, cellulose fragment (2), which is quickly depolymerized (k2) and the resulting monomers (4) rapidly mineralized to CO2 (k5). A conceivable alternate pathway (k3k4) involving depolymerization of 1 followed by deprotection of the substituted monomer (3) is thought to be prohibitively slow, as many semisynthetic celluloses with high DS are relatively inert to cellulases (k2 >> k3) (Puls et al., 2011). The slow mineralization rates of highly substituted celluloses, combined with the simultaneous decreases in molecular weight and DS observed during semisynthetic biodegradation (Gu et al., 1993; Puls et al., 2011; Yadav and Hakkarainen, 2021) are consistent with the k1k2 pathway, where k1 is rate-limiting.
FIGURE 4. General mechanism for the mineralization of cellulose and semisynthetic derivatives. R represents a semisynthetic group as specified in Figure 1A. This framework is consistent with prior reports (see reference 16).
Intrinsic chemical reactivity rates predict that biodegradability would proceed more rapidly with esterified CA than the alpha-ether CMC. The observed inversion (kobs (CA) < kobs (CMC)) could result from enzymatic catalysis or differences in DS (3 and 0.7, respectively). Nonlinear curve fitting enabled us to determine whether differences in DS alone could account for this anomaly. Eq. 3 (Hart-Cooper et al., 2015a), which weights the mineralization rates of derivatized (ka) and nonderivatized (kb) cellulose with their respective carbon mol fractions (fA, fB), was used to deconvolute these steps separately when incorporated into Eq. 4 (Supplementary Material). When DS > 1, fA = 1 and fB = 0, reducing Eq. 3 to Eq. 1, where kobs = ka. In the case of cellulose, fA = 0, fB = 1 and kobs = kb. Fitting Eq. 3 to the mineralization curves of CMC revealed kb values that were comparable (wastewater) or slightly less (compost) than that of cellulose (Table 2; Supplementary Figure S2).
TABLE 2. Kinetic parameters of cellulose and carboxymethyl cellulose (CMC; DS 0.7) in soil and wastewater conditions.
Of the partially substituted CMC copolymer, its 30% unmodified cellulose portion could readily account for the observed mineralization rate in both soil and wastewater. An upper limit for ka was determined to be at least an order of magnitude smaller than the rate of cellulose mineralization (kb), implying environmental lifetimes on the order of years for the carboxymethyl-substitution portion of CMC (DS 0.7), which is comparable to the fully substituted semisynthetics that were tested. Upper estimates of CMC deprotection rates (ka) were comparable to kobs of CA mineralization, which is consistent with the notion that ester hydrolysis is at least as fast as carboxymethyl cleavage. A lower DS thus accounted for the faster mineralization of CMC relative to CA.
Commercial grade semisynthetic celluloses, which are common ingredients in consumer products especially single-use items, packaging, and cigarette filters, mineralized up to 100 times more slowly than cellulose when degraded in soil compost. Semisynthetic derivatives, CA and CMC, afforded measurable degradation rates in this typical garden soil compost, as well as in aerobic wastewater, while no measurable degradation was observed with HPMC, EC, and HEC. A similar effect was observed with guar and HPG. Biodegradation rates decreased in the order guar > cellulose > CMC > HPG > CA > HPMC, HEC, EC. Mineralization in wastewater (30°C) was generally an order of magnitude slower than compost (60°C). These results are consistent with a biodegradation mechanism involving rate-limiting deprotection to yield the natural polymer, which is rapidly mineralized. Deprotection rates for semisynthetic biopolymers are at least one order of magnitude slower than mineralization of the natural biopolymer (cellulose or guar). This observation implies that highly synthetically substituted biopolymers may, when water-soluble, persist invisibly for up to decades in the natural environment. Based on initial rates, this degree of persistence is comparable to some conventional plastics (Chamas et al., 2020).
Respirometry experiments have limitations. Experiments are conducted in isolated containers under controlled conditions. Biodegradation rates are dependent upon the selected conditions, temperature, microbial population levels and compositions. Mineralization rates in an uncontrolled environment would vary considerably with temperature fluctuations, the introduction of organic matter, moisture levels, oxygen availability, the activity of microbial compositions. For example, organic matter that could biodegrade relatively quickly in some environments may persist for centuries in a dry, cold, or toxic environment, such as a landfill (Bogner, 1990). Additionally, predicting material lifetimes by extrapolation carries the assumption that the entire degradation process will follow a pseudo first-order relationship. Mineralization rates may deviate from first-order kinetics and slow down, or even accelerate, later in the degradation process. Nevertheless, determining the relative mineralization rates in environments to which materials may be most likely exposed, and providing an estimate of their persistence, is important in anticipating the extent to which these materials will impact the environment in the long-term.
While longer duration studies are needed, it is conceivable that highly substituted biopolymers may exhibit comparable persistence to conventional plastics. Perhaps of most concern is that with the degradation of the polymer backbone, the substituted synthetic groups could be released into the environment as a form of microplastic. Microplastics are a concern due to the many health conditions associated with their accumulation in the human body and their discovery in most ecosystems (Hasan et al., 2021; Osman et al., 2023).
Despite their benefit as renewable materials, the slow mineralization kinetics of these semisynthetic biopolymers highlight the need and opportunity to develop more rapidly degradable compositions for non-durable products where biodegradation is desired. Environmental pollution resulting from highly substituted semisynthetic biopolymers may be greatly reduced by replacing them with materials with less or no substitution. Additional research on non-synthetic modification of material properties is likewise needed. For example, hydrothermal processing is a simple, low cost, and environmentally friendly process for modifying the properties of starches, without the use of chemical treatments and resulting hazardous waste (Dutta and Sit, 2022).
The original contributions presented in the study are included in the article/Supplementary Material, further inquiries can be directed to the corresponding author.
WH-C: Conceptualization, Data curation, Formal Analysis, Funding acquisition, Investigation, Methodology, Project administration, Resources, Software, Supervision, Validation, Visualization, Writing–original draft, Writing–review and editing. NK: Conceptualization, Data curation, Formal Analysis, Methodology, Writing–review and editing. AK: Data curation, Investigation, Methodology, Writing–review and editing. LT: Conceptualization, Methodology, Validation, Writing–review and editing. GG: Investigation, Methodology, Project administration, Resources, Writing–review and editing. JC: Methodology, Software, Validation, Visualization, Writing–review and editing. KJ: Conceptualization, Investigation, Methodology, Writing–review and editing. WO: Project administration, Resources, Writing–review and editing.
The author(s) declare financial support was received for the research, authorship, and/or publication of this article. This project was funded by the USDA.
The authors are grateful to Diana Franqui for helpful discussions.
Author KJ was employed by Method Products.
The remaining authors declare that the research was conducted in the absence of any commercial or financial relationships that could be construed as a potential conflict of interest.
All claims expressed in this article are solely those of the authors and do not necessarily represent those of their affiliated organizations, or those of the publisher, the editors and the reviewers. Any product that may be evaluated in this article, or claim that may be made by its manufacturer, is not guaranteed or endorsed by the publisher.
The Supplementary Material for this article can be found online at: https://www.frontiersin.org/articles/10.3389/fmats.2023.1331308/full#supplementary-material
CMC, carboxymethyl cellulose; CA, cellulose acetate; HPMC, hydroxypropyl methylcellulose; HEC, hydroxyethyl cellulose; EC, ethyl cellulose; HPG, hydroxypropyl guar.
Andrady, A. L. (2011). Microplastics in the Marine environment. Mar. Pollut. Bull. 62 (8), 1596–1605. doi:10.1016/j.marpolbul.2011.05.030
Andrady, A. L. (2017). The plastic in microplastics: a review. Mar. Pollut. Bull. 119 (1), 12–22. doi:10.1016/j.marpolbul.2017.01.082
ASTM D5338-98(2003), Standard Test Method for Determining (2003). Aerobic biodegradation of plastic materials under controlled composting conditions. West Conshohocken, PA: ASTM International. https://www.astm.org/d5338-15r21.html (Accessed December 14, 2023).
Bogner, J. E. (1990). Controlled study of landfill biodegradation rates using modified BMP Assays. Waste Manag. Res. 8 (5), 329–352. doi:10.1016/0734-242X(90)90073-V
Bombelli, P., Howe, C. J., and Bertocchini, F. (2017). Polyethylene Bio-degradation by Caterpillars of the wax moth Galleria Mellonella. Curr. Biol. 27 (8), R292–R293. doi:10.1016/j.cub.2017.02.060
Buchanan, C. M., Gardner, R. M., and Komarek, R. J. (1993). Aerobic biodegradation of cellulose acetate. J. Appl. Polym. Sci. 47 (10), 1709–1719. doi:10.1002/app.1993.070471001
Buckley, H. L., Hart-Cooper, W. M., Kim, J. H., Faulkner, D. M., Cheng, L. W., Chan, K. L., et al. (2017). Design and testing of Safer, more effective Preservatives for consumer products. ACS Sustain. Chem. Eng. 5 (5), 4320–4331. doi:10.1021/acssuschemeng.7b00374
Bugge, M. M., Hansen, T., and Klitkou, A. (2016). What is the bioeconomy? A review of the Literature. Sustainability 8 (7), 691. doi:10.3390/su8070691
Chamas, A., Moon, H., Zheng, J., Qiu, Y., Tabassum, T., Jang, J. H., et al. (2020). Degradation rates of plastics in the environment. ACS Sustain. Chem. Eng. 8 (9), 3494–3511. doi:10.1021/acssuschemeng.9b06635
Debeaufort, F., Quezada-Gallo, J.-A., and Voilley, A. (1998). Edible films and Coatings: tomorrow’s packagings: a review. Crit. Rev. Food Sci. Nutr. 38 (4), 299–313. doi:10.1080/10408699891274219
Driedger, A. G. J., Dürr, H. H., Mitchell, K., and Van Cappellen, P. (2015). Plastic Debris in the Laurentian Great Lakes: a review. J. Gt. Lakes. Res. 41 (1), 9–19. doi:10.1016/j.jglr.2014.12.020
Dutta, D., and Sit, N. (2022). Comparison of properties of films prepared from Potato Starch modified by Annealing and heat–moisture treatment. Starch - Stärke 74 (11–12), 2200110. doi:10.1002/star.202200110
Flynn, A., Torres, L. F., Hart-Cooper, W., McCaffrey, Z., Glenn, G. M., Wood, D. F., et al. (2020). Evaluation of biodegradation of Polylactic Acid mineral Composites in composting conditions. J. Appl. Polym. Sci. 137 (32), 48939. doi:10.1002/app.48939
Frank, B. P., Smith, C., Caudill, E. R., Lankone, R. S., Carlin, K., Benware, S., et al. (2021). Biodegradation of functionalized nanocellulose. Environ. Sci. Technol. 55 (15), 10744–10757. doi:10.1021/acs.est.0c07253
Green, J. M. (2007). Review of Glyphosate and ALS-inhibiting herbicide Crop resistance and resistant weed Management. Weed Technol. 21 (2), 547–558. doi:10.1614/WT-06-004.1
Green, J. M., and Owen, M. D. K. (2011). Herbicide-resistant Crops: utilities and Limitations for herbicide-resistant weed Management. J. Agric. Food Chem. 59 (11), 5819–5829. doi:10.1021/jf101286h
Gu, J.-D., Eberiel, D., McCarthy, S. P., and Gross, R. A. (1993). Degradation and mineralization of cellulose acetate in Simulated Thermophilic compost environments. J. Environ. Polym. Degr 1 (4), 281–291. doi:10.1007/BF01458295
Hart-Cooper, W. M., Sgarlata, C., Perrin, C. L., Toste, F. D., Bergman, R. G., and Raymond, K. N. (2015a). Protein-like Proton exchange in a synthetic host Cavity. PNAS 112 (50), 15303–15307. doi:10.1073/pnas.1515639112
Hart-Cooper, W. M., Zhao, C., Triano, R. M., Yaghoubi, P., Ozores, H. L., Burford, K. N., et al. (2015b). The effect of host Structure on the Selectivity and mechanism of Supramolecular catalysis of Prins Cyclizations. Chem. Sci. 6 (2), 1383–1393. doi:10.1039/C4SC02735C
Hasan Anik, A., Hossain, S., Alam, M., Binte Sultan, M., Hasnine, M. D. T., and Rahman, Md. M. (2021). Microplastics pollution: a comprehensive review on the sources, fates, effects, and potential remediation. Environ. Nanotechnol. Monit. Manag. 16, 100530. doi:10.1016/j.enmm.2021.100530
Hoffman, K., Sosa, J. A., and Stapleton, H. M. (2017). Do flame retardant chemicals increase the risk for Thyroid Dysregulation and cancer? Curr. Opin. Oncol. 29 (1), 7–13. doi:10.1097/CCO.0000000000000335
International Organization for Standardization (2019). Determination of the ultimate aerobic biodegradability of plastic materials in an aqueous medium. Available at: https://www.iso.org/standard/70026.html (Accessed December 14, 2023).
Kim, J. H., Hart-Cooper, W., Chan, K. L., Cheng, L. W., Orts, W. J., and Johnson, K. (2016). Antifungal efficacy of octylgallate and 4-isopropyl-3-Methylphenol for control of Aspergillus. Microbiol. Discov. 4 (1), 2. doi:10.7243/2052-6180-4-2
Kint, D., and Muñoz-Guerra, S. (1999). A review on the potential biodegradability of Poly(ethylene terephthalate). Polym. Int. 48 (5), 346–352. doi:10.1002/(SICI)1097-0126(199905)48:5<346::AID-PI156>3.0.CO;2-N
Kubowicz, S., and Booth, A. M. (2017). Biodegradability of plastics: challenges and Misconceptions. Environ. Sci. Technol. 51 (21), 12058–12060. doi:10.1021/acs.est.7b04051
Li, W. C., Tse, H. F., and Fok, L. (2016). Plastic waste in the Marine environment: a review of sources, occurrence and effects. Sci. Total Environ. 566–567, 333–349. doi:10.1016/j.scitotenv.2016.05.084
Matharu, A. S., de Melo, E. M., and Houghton, J. A. (2016). Opportunity for high value-added chemicals from food supply Chain wastes. Bioresour. Technol. 215, 123–130. doi:10.1016/j.biortech.2016.03.039
Mathur, S., and Singh, R. (2005). Antibiotic resistance in food Lactic Acid bacteria—a review. Int. J. Food Microbiol. 105 (3), 281–295. doi:10.1016/j.ijfoodmicro.2005.03.008
Morét-Ferguson, S., Law, K. L., Proskurowski, G., Murphy, E. K., Peacock, E. E., and Reddy, C. M. (2010). The size, Mass, and composition of plastic Debris in the western north Atlantic ocean. Mar. Pollut. Bull. 60 (10), 1873–1878. doi:10.1016/j.marpolbul.2010.07.020
Ngo, A. D., Taylor, R., Roberts, C. L., and Nguyen, T. V. (2006). Association between agent orange and birth defects: systematic review and Meta-analysis. Int. J. Epidemiol. 35 (5), 1220–1230. doi:10.1093/ije/dyl038
Nie, H., Liu, M., Zhan, F., and Guo, M. (2004). Factors on the Preparation of Carboxymethylcellulose hydrogel and its degradation Behavior in soil. Carbohydr. Polym. 58 (2), 185–189. doi:10.1016/j.carbpol.2004.06.035
Osman, A. I., Fawzy, S., Farrell, C., Al-Muhtaseb, A. H., Harrison, J., Al-Mawali, S., et al. (2022). Comprehensive Thermokinetic Modelling and Predictions of cellulose Decomposition in isothermal, non-isothermal, and stepwise heating Modes. J. Anal. Appl. Pyrolysis 161, 105427. doi:10.1016/j.jaap.2021.105427
Osman, A. I., Hosny, M., Eltaweil, A. S., Omar, S., Elgarahy, A. M., Farghali, M., et al. (2023). Microplastic sources, formation, Toxicity and remediation: a review. Environ. Chem. Lett. 21 (4), 2129–2169. doi:10.1007/s10311-023-01593-3
Philp, J. C., Ritchie, R. J., and Allan, J. E. M. (2013). Biobased chemicals: the Convergence of green chemistry with industrial Biotechnology. Trends Biotechnol. 31 (4), 219–222. doi:10.1016/j.tibtech.2012.12.007
Polman, E. M. N., Gruter, G.-J. M., Parsons, J. R., and Tietema, A. (2021). Comparison of the aerobic biodegradation of biopolymers and the corresponding Bioplastics: a review. Sci. Total Environ. 753, 141953. doi:10.1016/j.scitotenv.2020.141953
Puls, J., Wilson, S. A., and Hölter, D. (2011). Degradation of cellulose acetate-based materials: a review. J. Polym. Environ. 19 (1), 152–165. doi:10.1007/s10924-010-0258-0
Reyes, B. A., Pendergast, J. S., and Yamazaki, S. (2008). Mammalian Peripheral Circadian oscillators are temperature Compensated. J. Biol. Rhythms 23 (1), 95–98. doi:10.1177/0748730407311855
Rivard, C. J., Adney, W. S., Himmel, M. E., Mitchell, D. J., Vinzant, T. B., Grohmann, K., et al. (1992). Effects of natural polymer Acetylation on the Anaerobic Bioconversion to Methane and carbon Dioxide. Appl. Biochem. Biotechnol. 34–35 (1), 725–736. doi:10.1007/BF02920592
Stewart, P. S., and William Costerton, J. (2001). Antibiotic resistance of bacteria in Biofilms. Lancet 358 (9276), 135–138. doi:10.1016/S0140-6736(01)05321-1
Torgbo, S., and Sukyai, P. (2020). Biodegradation and thermal Stability of bacterial cellulose as biomaterial: the relevance in Biomedical Applications. Polym. Degrad. Stab. 179, 109232. doi:10.1016/j.polymdegradstab.2020.109232
Vaughn, B., Andrea, W., and Kyle, S. (2013). Perfluorooctanoic Acid (PFOA) exposures and incident cancers among Adults living near a chemical plant. Environ. Health Perspect. 121 (11–12), 1313–1318. doi:10.1289/ehp.1306615
Wirick, M. G. (1974). Aerobic biodegradation of Carboxymethylcellulose. J. (Water Pollut. Control Fed. 46 (3), 512–521. Available at: Aerobic biodegradation of carboxymethylcellulose—PubMed (nih.gov) and Aerobic Biodegradation of Carboxymethylcellulose on JSTOR.
Yadav, N., and Hakkarainen, M. (2021). Degradable or not? Cellulose acetate as a model for Complicated interplay between Structure, environment and degradation. Chemosphere 265, 128731. doi:10.1016/j.chemosphere.2020.128731
Keywords: modified celluloses, cellulose acetate, carboxymethyl cellulose, hydroxypropyl methylcellulose, hydroxyethyl cellulose, ethyl cellulose, green chemistry, environment
Citation: Hart-Cooper WM, Kalla N, Klamczynski A, Torres L, Glenn GM, Cunniffe J, Johnson K and Orts WJ (2024) Predicting environmental biodegradability using initial rates: mineralization of cellulose, guar and their semisynthetic derivatives in wastewater and soil. Front. Mater. 10:1331308. doi: 10.3389/fmats.2023.1331308
Received: 31 October 2023; Accepted: 18 December 2023;
Published: 11 January 2024.
Edited by:
Shanju Zhang, California Polytechnic State University, United StatesReviewed by:
Ahmed Ibrahim Osman, Queen’s University Belfast, United KingdomCopyright © 2024 Hart-Cooper, Kalla, Klamczynski, Torres, Glenn, Cunniffe, Johnson and Orts. This is an open-access article distributed under the terms of the Creative Commons Attribution License (CC BY). The use, distribution or reproduction in other forums is permitted, provided the original author(s) and the copyright owner(s) are credited and that the original publication in this journal is cited, in accordance with accepted academic practice. No use, distribution or reproduction is permitted which does not comply with these terms.
*Correspondence: William M. Hart-Cooper, d2lsbGlhbS5oYXJ0LWNvb3BlckB1c2RhLmdvdg==
Disclaimer: All claims expressed in this article are solely those of the authors and do not necessarily represent those of their affiliated organizations, or those of the publisher, the editors and the reviewers. Any product that may be evaluated in this article or claim that may be made by its manufacturer is not guaranteed or endorsed by the publisher.
Research integrity at Frontiers
Learn more about the work of our research integrity team to safeguard the quality of each article we publish.