- 1Facultad de Química, Universidad Nacional Autónoma de México, Ciudad Universitaria, Mexico City, Mexico
- 2Tecnologico de Monterrey, Escuela de Ingeniería y Ciencias, Mexico City, Mexico
- 3Instituto de Investigaciones en Materiales, Universidad Nacional Autónoma de México, Ciudad Universitaria, Mexico City, Mexico
Introduction: One of the most attractive fields for cellulose aerogels is water remediation, basically in the pollutant’s adsorption and oil/water separation. There are different sources to extract cellulose, but the extraction from paper waste is an excellent option for impulse circular materials applications.
Methods: We obtained cellulose from office waste paper through a simple alkali method and used it to fabricate an aerogel by freeze-drying based on the materials’ circularity. To increase lipophilicity, the aerogel was modified using two different coatings: polycaprolactone (WOPP) and candelilla wax (WOPW), extracted from a Mexican plant. The aerogels were analyzed by several physicochemical techniques such as Scanning Electronic Microscopy, Infrared spectroscopy, and thermal analysis.
Results and Discussion: To our knowledge, this is the first time that candelilla wax has been reported as aerogel modification. The highly porous aerogels showed a density of around 0.1 g/cm3 and a fibrous structure. Furthermore, the contact angle of the aerogels was measured to compare the hydrophobicity of the surfaces, showing values around 120° in the modified aerogels compared with the hydrophilic behavior of pristine cellulose aerogel. The achieved recycled mineral oil absorption capacity for WOPW was 6.1 g/g, while for WOPP was 4.88 g/g. Thus, we obtained a natural coating aerogel with a high water/oil separation potential.
1 Introduction
Securing access to sufficient and clean water stands as a pressing global challenge, marked by its increasing scarcity and degradation (Mishra et al., 2021). The industrial footprint, extending across the globe, has exacerbated this issue by discharging hazardous pollutants into aquatic ecosystems. These pollutants include heavy metals, radioactive materials, pesticides, dyes, pharmaceuticals, chiral chemicals, and, notably, oil contaminants (Akpomie and Conradie, 2020). The menace posed by oil is particularly pronounced, representing a significant threat to both aquatic and terrestrial life due to its coating properties, unsightly appearance, and noxious odor (Laitinen et al., 2017). Addressing this challenge requires effective oil absorption and separation techniques, ranging from physical methods and chemical treatments to bioremediation (Bayat et al., 2005). Among these, oil absorption, characterized by its cost-effectiveness and straightforward process, is considered a prominent method, with recovered oil easily retrievable through squeezing, distillation, or extraction (Cheng et al., 2017).
In recent times, aerogels have emerged as a focal point of interest for oil/water separation, owing to their remarkable characteristics such as high porosity, low density, and a large surface area (Yuan et al., 2017; Zhang et al., 2019). The key to their effectiveness in selectively absorbing oil in water lies in their hydrophobic and oleophilic nature (Sai et al., 2015). The wet contact angle (WCA) serves as a metric for classifying aerogels by their hydrophobicity: below 90° is hydrophilic, between 90° and 150° is hydrophobic, and values exceeding 150° are considered superhydrophobic (Laitinen et al., 2017). Surface modifications using silylation routines, employing compounds like methyltrimethoxysilane (MTMS) (Nguyen et al., 2013) or trimethylchlorosilane (TMCS) (Fan et al., 2017), enhance the aerogel’s hydrophobicity. Additionally, environmentally friendly polymeric separators like polycaprolactone (PCL) have found application (He et al., 2021).
Conversely, inorganic materials like silica and polymers such as polyvinyl alcohol (PVA) (Yang et al., 2022; Lu et al., 2023) have been extensively used for fabricating aerogels to absorb oil from water. However, their non-biodegradable nature raises environmental concerns, highlighting the growing need for efficient and cost-effective materials for oil and water separation (Yang et al., 2022). This has led to an increased focus on biopolymers sourced from biomass residues, including cellulose, lignin, or chitosan, due to their advantageous characteristics such as abundant raw materials, low cost, and environmental friendliness (Zhang et al., 2023).
Cellulose, as the most abundant polymer on Earth, emerges as an ideal candidate for aerogel fabrication. It boasts renewability, biocompatibility, biodegradability, low density, high porosity, and a large specific surface area (Long et al., 2018). Cellulose can be sourced from various natural resources such as bamboo, cotton, pineapple, and sugarcane bagasse (Zhang et al., 2018; Do et al., 2020; Li et al., 2021), among others. The potential of cellulose-based aerogels for oil absorption has been widely explored, particularly utilizing waste paper as a sustainable source. Nguyen et al. (2013) achieved notable success in developing an ultralight porous hydrophobic material from waste paper using MTMS, showcasing substantial absorption capacities for different crude oils.
Similarly, Han et al. (2012) synthesized a hydrophobic and porous aerogel using waste newspaper as the sole raw material, demonstrating impressive absorption capabilities for organic solvents and oils. The resulting product could be recycled and reused through squeezing, combustion, and distillation. Fan et al. (2017) continued this trajectory, utilizing newspaper as the sole raw material, crosslinking with glutaraldehyde, and treating with TMCS to obtain a hydrophobic and porous cellulose-based aerogel with significant oil absorption capacities. Laitinen et al. (2017) produced a cellulose nanofibril aerogel with MTMS hydrophobic modification by freeze-drying, exhibiting impressive absorption capacities for diverse oils and organic solvents, including the recyclability of 30 cycles for diesel oil. Additionally, Sanguanwong et al. (2020) employed napkins with MTMS to obtain an aerogel capable of absorbing oil, with a recyclability of 10 cycles dependent on freeze-drying temperatures.
In this study, we present a groundbreaking approach to fabricating an aerogel using reconstituted cellulose sourced from waste paper. To augment its efficacy in adsorbing mineral oils, we performed surface modifications on the cellulose aerogel by employing two hydrophobic polymers. The key objectives of this project encompass providing a practical and cost-effective solution for repurposing waste paper, coupled with the efficient removal of hydrophobic contaminants.
To realize these objectives, we strategically selected two distinct coatings. Firstly, we applied poly-ε-caprolactone, a biodegradable polyester known for its robust mechanical and thermal properties. This polymer significantly contributed to enhancing the aerogel’s durability and overall performance. Complementarily, we incorporated candelilla wax, a natural alternative renowned for augmenting the aerogel’s hydrophobic properties. The utilization of candelilla wax adds further value to our approach by leveraging a locally abundant Mexican product.
The amalgamation of waste paper, poly-ε-caprolactone, and candelilla wax aims to yield an aerogel with exceptional capabilities in adsorbing mineral oils, all while maintaining a commitment to environmental friendliness and economic viability. This innovative solution not only provides a sustainable avenue for the utilization of waste paper but also addresses the pressing need for efficient removal of hydrophobic contaminants. Ultimately, this project is poised to make significant contributions to both environmental preservation and economic advancement.
2 Materials and methods
2.1 Materials
The waste office paper (WOP) was collected from old documents and crushed as pretreatment. Urea, chloroform, and acetone were purchased from J.T. Baker. Analytical grade Sodium Hydroxide (NaOH) was obtained from Reactivos Química Meyer. Ethanol was purchased from CTR Scientific. Polycaprolactone (PCL, Mn = 80,000 Da, Tg = 60°C) was obtained from Sigma Aldrich, and Candelilla wax (CW) (MW = 436 g/mol, ρ = 0.9 g/cm3, melting point 70 °C) was purchased from ABREIKO S DE RL DE CV. Recycled mineral oil came from different laboratory equipment and was dyed using Chefmaster candy color red from a local chocolate store.
2.2 Cellulose extraction from office wastepaper
Cellulose fiber extraction from WOP was obtained by treating the paper with an alkali aqueous solution, NaOH (7 wt%), and urea (12 wt%). NaOH allows the dissolution of the cellulose, while the urea works as an additive that improves the extraction and delays the gelation process, allowing for better gel handling (Budtova, 2019). Then, 100 mL of solution was cool-prepared and refrigerated for 1 h at −20°C. Afterward, 4 g of pretreated WOP were added to the solution to dissolve the paper and extract the cellulose. An immersion blender was used to mix and crush the paper until a paste-like texture was obtained.
2.3 Aerogel elaboration
To replace the alkaline solution within the gel, 100 mL of ethanol was introduced, and the mixture was allowed to stand at room temperature for 24 h. This initiates the coagulation process, resulting in the formation of a stable alcogel. Subsequently, the alcogel was rinsed with distilled water until a neutral pH was achieved. The purified alcogel was then immersed in 50 mL of ethanol to facilitate a solvent exchange (Pircher et al., 2016). Once the alcogel was fully formed and stable, it was dried via lyophilization with a freeze dryer for 48 h at −50°C and 0.060 mPa. This process yields a stable WOP-based aerogel (control).
2.4 Surface modifications
To produce a hydrophobic aerogel, two methods of surface modification were employed. The initial modification involved the utilization of PCL as a plasticizer. For this, 0.5 g of PCL was dissolved in 50 mL of chloroform, and the aerogel was submerged in the solution for 15 min, followed by air-drying at room temperature for 1 h. Subsequently, it was subjected to another round of freeze-drying for 24 h. For the second modification, CW was used; 0.4 g of candelilla pellets were dissolved in 50 mL of acetone, and the aerogel was immersed for 15 min in the solution and freeze-dried for 24 h.
2.5 Physicochemical characterization
2.5.1 Aerogel properties
The general properties of the synthesized aerogels, such as their physical description, mass, dimensions, density, and porosity, were documented and reported to see their evolution throughout the experimental process.
The density (ρ) was calculated with Eq. 1, while porosity (P) was measured with Eq. 2.
Where m is the mass (g), V is the volume (cm3), and
2.5.2 Morphology
Scanning electron microscopy (SEM) studies were carried out using a DSM-950 (Zeiss, Germany) equipment with a QBSD detector for compositional contrast imaging operating at 20 kV. Samples of aerogels were deposited on pin stubs of aluminum and sputter coated with a thin carbon layer.
2.5.3 Chemical characterization
FT-IR spectra were acquired on an FTIR Nicolet 6700 (Thermo Fischer Scientific, United States) at a scanning range of 4,000–400 cm−1. This analysis was performed to characterize the components of the aerogels, proving the presence of cellulose and the Candelilla wax and PCL coatings.
2.5.4 Thermal characterization
Calorimetric data were obtained by differential scanning calorimetry (DSC) with a DSC 2910 (Modulated TA Instruments, United States) equipped with a refrigeration cooling system (RCS). Experiments were conducted under a flow of dry nitrogen with a sample weight of approximately 5 mg. Heating runs were performed at a rate of 10 °C/min, respectively. Thermal degradation was studied at a 10 °C/min heating rate in a TGA 2950 Thermogravimetric Analyzer (TA Instruments, United States) with ranged test temperatures from 25°C to 500 °C.
2.5.5 Wet contact angle (WCA)
Static contact angle measurements with the sessile water drop method were recorded and analyzed at room temperature using a low-cost contact angle analyzer (Han et al., 2022). Contact angle values of the right and left sides of the water drop were measured and averaged. Measurements were performed 5 s after the drop of 5 µL was deposited on the sample surface. All data were an average of ten measurements on different surface locations. The angle was estimated using Eq. 3.
Where h is the height of the droplet, w is its width and θ the Wet contact angle.
2.5.6 Absorption capacity and degradability in water
The aerogels’ oil absorption capacity (Q) was calculated by measuring their initial and final weight after saturation from an oil immersion using Eq. 4.
Where m0 and mf are the initial and final mass, respectively, measured in grams.
Finally, three samples of each aerogel, with and without coatings, were placed in an aqueous solution under agitation, and their weight loss was monitored for 3 days. After a defined time, the samples were freeze-dried for 24 hours. The weight loss percent was calculated using the Eq. (5a) and Eq. (5b).
Where m0 and mt are the initial and final mass, respectively, measured in grams.
3 Results and discussion
The optimization of the method for obtaining aerogels was initially carried out using new office paper to minimize the number of variables that could impact the correct cellulose aerogel production. During this phase, adding a second ethanol bath was necessary after neutralizing the paper mixture dissolved in NaOH/urea. Subsequently, tests were conducted using office waste paper, concluding that neutralizing the aerogel with ethanol baths was appropriate.
This step removed excess NaOH present in the alco-gel structure and effectively eliminated a significant amount of ink from the final material. By implementing these minor adjustments without the addition of any crosslinker, a successful cellulose aerogel was obtained from WOP, following similar procedures to those reported by Li et al. (2018). After optimizing the WOP-based aerogel, it underwent a hydrophobicity enhancement process by applying PCL (WOPP) and wax (WOPW) coatings. The detailed procedure is shown in Figure 1.
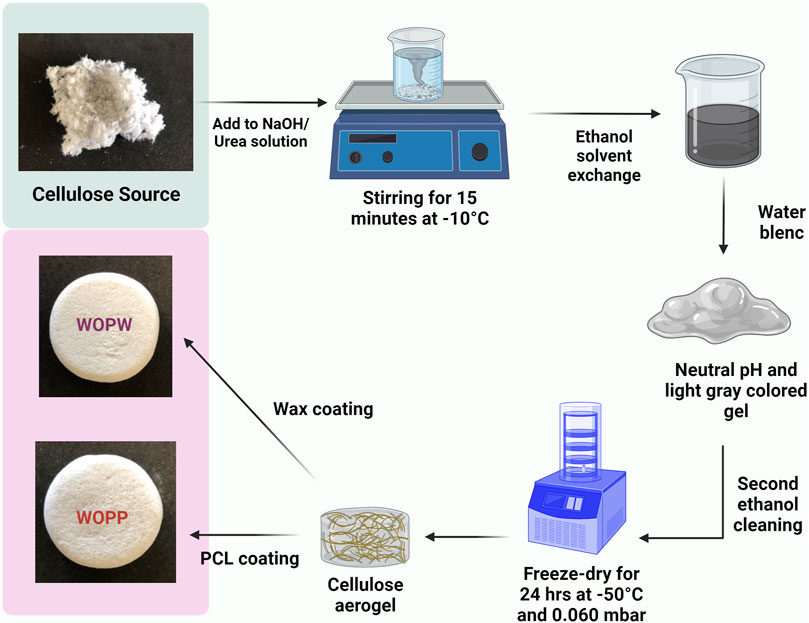
FIGURE 1. Schematic representation of the experimental procedure for obtaining the aerogels used in this study.
The FT-IR spectra of WOP (line a), WOP-based aerogel (line b), modified WOPP (line c), and WOPW (line d) aerogels are displayed in Figure 2. The spectra obtained from the analysis of office paper waste and the WOP-based aerogel reveal discernible differences in both composition and structure, arising from the fundamental maceration process applied to the paper. Notably, the WOP spectrum exhibits signals linked to the presence of hemicellulose around 1,672 cm−1, which becomes imperceptible in the WOP-base aerogel following the processing. Furthermore, characteristic lignin signals at ν = 1,595 and ν = 1,506 cm−1, indicative of aromatic hydrocarbons, are absent in the base aerogel, suggesting the successful removal of lignin during the bleaching process.
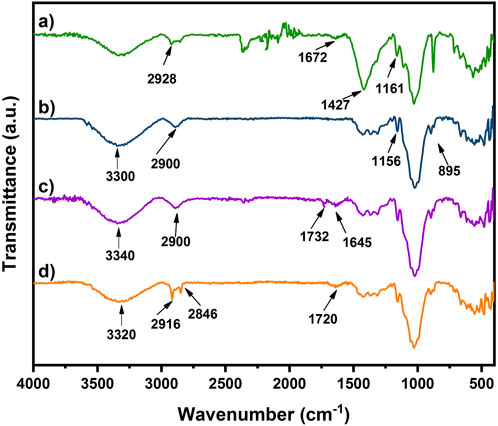
FIGURE 2. FTIR spectra of WOP (line a) and, WOP-based (line b), WOPP (line c), and WOPW (line d) aerogels.
Certain infrared signals are sensitive to structural changes within the transformed crystalline area of cellulose (Oh et al., 2005). For instance, the signal corresponding to the stretching modes of the hydroxyl -OH group, abundant in the cellulose structure, appears to increase after alkaline treatment, implying intensified inter- and intermolecular hydrogen bonds facilitated by sodium hydroxide. The maximum absorbance signal attributed to C-H stretches undergoes a shift from 2,928 to 2,900 cm−1, indicating changes in the arrangement of β-glycosidic linkage angles. Concurrently, the decrease in the 1,427 cm−1 signal assigned to CH2 unfolding suggests an increase in the number of disordered structures due to alkali action.
A subtle yet significant change is observed in the signal transitioning from 1,161 to 1,156 cm−1, associated with C-O-C stretching, indicative of the transformation of the crystal structure from cellulose I to cellulose II. The band at 895 cm−1, belonging to β-anomers or β-bonded glucose polymers, further confirms that the aerogel base primarily consists of cellulose without lignin or hemicellulose impurities. Hence, it can be confidently asserted that the cellulose extraction process from waste paper has been accomplished.
Poly-ε-caprolactone is a biodegradable polyester, wherein the intrinsic signals of the aliphatic groups appear around 2,900 cm−1, and a distinct peak at 1732 cm−1 corresponds to the ester group (C=O). Candelilla wax is primarily composed of n-alkanes with a chain length ranging between 29 and 33 carbons (≈50%) and high molecular weight esters (20%–29%) (Toro-Vazquez et al., 2007).
As a result, in WOPW spectra (Figure 2, line d), the characteristic signals of these compounds can be observed. Specifically, the peaks at νs = 2,916 and νas = 2,894 cm−1 are attributed to the symmetric and asymmetric vibrations of the saturated hydrocarbons. In contrast, the peak at ν = 1720 cm−1 corresponds to the vibrations of the functional group C=O of the esters. By observing the presence of the characteristic functional groups of these compounds in the WOPP and WOPW aerogels, it is possible to ensure that they were successfully coated and that these polymers are integrated into the material’s structure.
The coating is also evident in the electron microscopies shown in Figure 3. In these micrographs, the aerogels are observed at two different magnification levels. For the WOP-based aerogel, it is apparent that it is formed by cellulose fibers that are heterogeneously distributed, leaving spaces between them. This three-dimensional arrangement generates the presence of pores or free space within the structure. The fibers appear to be well integrated, which could contribute to the material’s adequate mechanical behavior.
In contrast, the aerogels coated with PCL (b, e) and candelilla wax (c, f) exhibit a different appearance. The fibers appear to be wrapped with a coating that has an oily aspect. Additionally, there is an accumulation of these coatings in certain areas, connecting the fibers and causing lamellae formation. The observed differences in the micrographs indicate the successful coating of the aerogels with PCL and candelilla wax, resulting in distinct structural features compared to the uncoated WOP-based aerogel. To enhance visibility during observation, some specific regions of the aerogels, corresponding to PCL and wax components, were delineated using red and blue arrows on the microscopy images.
The thermal analysis (TGA) provides insights into the thermal stability of the obtained aerogels (Figure 4). The WOPW sample (Figure 4, blue) shows no degradation until temperatures exceed 300 °C, while the WOPP (Figure 4, red) sample remains stable up to approximately 400 °C. The WOP-based aerogel (Figure 4, black) exhibits an initial weight loss at 100 °C, attributed to adsorbed water, accounting for around 10% of its weight. This indicates a high affinity of the aerogel for water.
Conversely, the aerogels coated with PCL and candelilla wax show no significant loss at 100 °C, proving they have acquired a hydrophobic character. This hydrophobicity is crucial for facilitating the adsorption of oils, the primary purpose of these materials. The thermal degradation process for the aerogel composed solely of cellulose occurs in a step between 260°C and 350 °C, resulting in a carbon yield of approximately 10%. This is due to the presence of non-volatile carbonaceous compounds, as previously observed in regenerated cellulose, associated with the formation of sodium salts during the basic hydrolysis process (Yeng et al., 2015; Hameed et al., 2022).
The coated samples exhibit a distinctive degradation process that closely corresponds to the behavior observed for pure coatings, primarily because the mass proportion of cellulose is low in the coated fibers. This indicates that the coatings predominantly determine the thermal behavior of the coated aerogels.
DSC heating runs shown in Figure 5 revealed that the samples were primarily amorphous. Three distinct endothermic transitions were observed for the regenerated cellulose sample (WOP-based aerogel, black). The first transition occurred between 43°C and 119 °C, which aligns with findings from other reports in the literature (Mahato et al., 2013) and is attributed to the rearrangement of the chains in the amorphous components due to the loss of adsorbed water molecules (Al-Maqdasi et al., 2021). The glass transition temperature (Tg) of an amorphous material is significantly influenced by the presence of water molecules, given their ability to exist in various thermodynamic states with different bonding configurations (Hancock and Zografi, 1994). This variability can exert a pronounced impact on molecular mobility, particularly affecting the behavior of end groups and side chains (Picker and Hoag, 2002).
Two additional transitions are observed, with the second occurring between 125°C and 187 °C and the third between 195°C and 235 °C. These transitions underscore the heterogeneous structure of the sample, indicating distinct thermal properties in different regions (Picker and Hoag, 2002). During the maceration process, it is plausible that the cellulose base structure undergoes varied changes in each region, encompassing both physical alterations, such as changes in crystalline states, and chemical modifications, including modifications of β-glycosidic bonds.
Moreover, native cellulose fibers and their derivatives typically exhibit an extended chain fibrous structure with potential orientation in different directions (Morán et al., 2008). Heating may induce rearrangements in this orientation, contributing to the observed multiple transitions. Notably, when transitions unrelated to the amorphous part decrease in intensity, it indicates a lower ratio between the crystalline and amorphous components (Mwaikambo and Ansell, 2002).
In summary, the transitions in the structure of regenerated cellulose manifest diverse physicochemical phenomena within the material’s architecture. Finally, at 320 °C, the depolymerization of cellulose occurred due to the rupture of the glycosidic bonds.
The influence of coatings on thermal transitions is evident. For example, in WOPP, a pronounced first transition is observed because the glass transition or melting point of polycaprolactone typically occurs between 60°C–70 °C, depending on the molecular weight (Balsamo et al., 2001; Gómez-Lizárraga et al., 2017). The shift to 78 °C of this transition concerning the control indicates that more energy is required for the molecular reorganization of the PCL-coated aerogel, making it more stable to thermal changes, it seems that the presence of cellulose facilitated the crystallization of PCL, probably because of a nucleating effect Similarly, the second transition observed in the WOPP aerogel suggests an enhancement in the structural organization of cellulose, as evidenced by the heightened intensity of the transition at 212 °C. As previously discussed, this phenomenon appears to be closely associated with the rearrangement occurring within the crystalline portion of the fibers.
Regarding the aerogel coated with candelilla wax, it is possible to observe that the first endothermic transition is split into two compared to the control (Toro-Vazquez et al., 2011; Lionetto et al., 2019). This characteristic behavior is attributed to candelilla wax, as it first undergoes the rearrangement of the n-alkane chains, mainly hentriacontane, to its crystalline form at 57 °C. Subsequently, around 66 °C, melting occurs, which is associated with the structural order of the gelator molecules within the assembly units (i.e., microplatelet crystals) that form the three-dimensional crystalline structure of wax.
Thus, it becomes evident that both coatings affect the modification of the thermal properties of the aerogels. However, it is clear that in the case of the polycaprolactone coating, the thermal properties are more significantly affected, resulting in increased stability.
The contact angles (θ) determined using water droplets indicate that the WOPP (θ = 129°) and WOPW (θ = 123°) aerogels are hydrophobic materials after coating (θ > 120°), in contrast to the uncoated WOP-based aerogel where it was not possible to determine the contact angle because the drop was fully adsorbed immediately after being deposited on the surface (Figure 6).
This behavior is due to the chemical composition of the aerogel surface, as both PCL and candelilla are composed of non-polar compounds (aliphatic chains) that are unable to form hydrogen bonds with water molecules. Additionally, the presence of the coatings alters the roughness of the materials (r), which, according to the Wenzel equation (Eq. 5), directly affects the wettability of the materials.
Thus, it is evident that the contact angles obtained result not only from the chemical structure of the materials but also from the material’s morphology. The evaluation of the transversal section of the aerogels showed contact angles for WOPP of 115° ± 7, while for WOPW, they were approximately 112° ± 4. In the case of the uncoated aerogel, determining the contact angle proved impossible as the droplet was promptly absorbed. The decrease in contact angle can be attributed to exposed portions of uncoated fibers due to the cut; nonetheless, the hydrophobic behavior remains evident. Notably, the coatings allow us to obtain aerogels with the necessary hydrophobic character for the correct adsorption of contaminant oils (Supplementary Video S1).
By comparing with previous reports, we observe that the obtained values fall within the desired range. We achieved these using coatings that do not raise additional contamination concerns, as is the case with a commonly used inorganic coating like silanes, with contact angles between 120° and 150° have been obtained (Nguyen et al., 2013; Duan et al., 2014; Feng et al., 2015; Zhai et al., 2015; Rafieian et al., 2018; Sanguanwong et al., 2020). We obtained highly hydrophobic aerogels using clean and natural alternatives like candelilla wax.
In Figure 7, it is possible to observe how the coated aerogels have the capacity to adsorb mineral oil drops placed on their surface (orange) while repelling water droplets (stained blue) confirming that they are ideal materials to be tested for removing these contaminants.
The average physical characteristics of the obtained aerogels are presented in Table 1. Notably, the aerogels exhibit a remarkably high porosity (>93%), indicating a substantial amount of free space within their structure to accommodate adsorbed contaminants. These porosity values are comparable to data previously reported for aerogels derived from waste paper.
For instance, Gong et al. (2021) reported obtaining aerogels composed of cellulose nanofibers with a porosity exceeding 99%. Considering that our aerogels, formed from microfibers, show only a 6% difference in porosity compared to Gong’s nanofiber-based aerogels, it can be regarded as a successful outcome. Moreover, our method utilizes economically viable materials and a simple process, presenting a promising avenue for giving the waste paper a second purpose.
The mineral oil absorption was carefully monitored for over 1 hour, and the images depicted in Figure 8. Remarkably, the WOPW aerogel exhibited the highest absorption capacity, reaching an impressive 6.1 g/g value. Even more noteworthy is that it achieved maximum absorption within 5 min, demonstrating its rapid and efficient oil-absorbing capabilities.
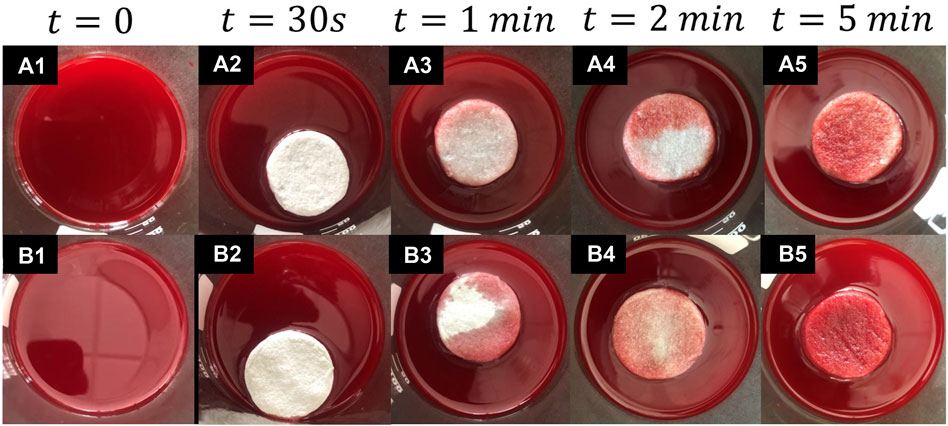
FIGURE 8. Images of mineral oil (stained red) adsorption process over time in WOPP (A1-A5) and WOPW (B1-B5) (B).
Impressively, this aerogel can absorb seven times its weight in mineral oil, showcasing its exceptional performance.
In contrast, the PCL-coated aerogel, WOPP, displayed a slightly lower absorption capacity of 4.88 g/g. The peak absorption capacity is achieved approximately 5 min into the process. Beyond this point, the quantity of oil retained in the aerogel plateaus, signifying that the aerogel has reached its saturation point. Although it showed a slightly reduced absorption rate compared to WOPW, it still exhibits significant potential for oil absorption.
The observed differences in absorption capacities between WOPW and WOPP could be attributed to the distinctive properties of their respective coatings. The inherent characteristics of the WOPW aerogel, coupled with its surface properties, likely contribute to its rapid and superior absorption performance. On the other hand, the PCL-coated WOPP aerogel, while slightly less absorbent, still represents a promising option for oil absorption applications.
Comparing the absorption capacity of our aerogels with those previously reported, such as the works by Yi et al. (2020), Wang et al. (2019), and Xu et al. (2019), it is evident that their capacities may not be the most outstanding. These reports have shown absorption capacities exceeding 20 g/g, attributed to lower densities and higher porosities of the aerogels used in their studies. It is important to acknowledge that cellulose derived from other sources was utilized in these works.
However, it is crucial to highlight the primary objective of our study, which is to leverage waste paper as a sustainable and environmentally friendly resource. The focus here is on repurposing waste paper, a significant factor in environmental pollution, and transforming it into high-performing aerogels with oil-absorbing capabilities. By utilizing waste paper, we can effectively address environmental concerns while producing valuable materials for oil spill response and other related applications.
Considering the environmentally conscious aspect of our approach, the achieved absorption capacities of 6.1 g/g for WOPW and 4.88 g/g for WOPP are indeed commendable. These aerogels demonstrate efficient oil absorption rates, making them promising candidates for practical applications, especially when rapid and effective oil cleanup is essential.
Stability and degradability tests in water were conducted to assess the viability of the aerogels, as illustrated in Figure 9. Remarkably, after 3 days immersed in an aqueous medium with continuous agitation, these aerogels exhibited a stability fivefold greater than that of cellulose aerogels. It is noteworthy to consider that, in their proposed use {of static filters, they would not be subject to the continuous wear and tear caused by agitation. Nevertheless, given their biodegradable origin, it is reasonable to anticipate a considerable degradation over a relatively short time frame.
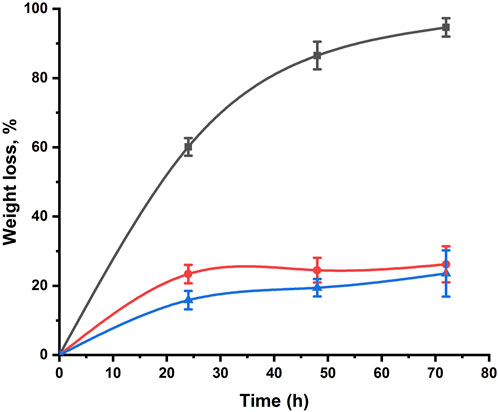
FIGURE 9. Aerogel degradation in aqueous media, WOP-based (black line), WOPP (red line) and WOPW (blue line).
Therefore, the results of this study provide a solid foundation for developing sustainable and eco-friendly solutions for managing oil spills and similar environmental challenges. While there may be aerogels with higher absorption capacities in the literature, our work significantly contributes to the advancement of eco-conscious materials derived from waste paper, paving the way for a greener and more sustainable future.
4 Conclusion
In this work, super hydrophobic cellulose-based aerogels were obtained. The low density, high porosity, and remarkable adsorption properties make them attractive candidates to be employed in water/oil separation. The Candelilla wax coating effectively decreased cellulose’s intrinsic hydrophilicity, achieving a contact angle larger than 120°. The Candelilla wax-coated aerogel showed a 6.1 g/g absorption capacity for mineral oil and a fibrillar structure. Further studies analyzing the behavior of the aerogel with other solvents are needed. Also, some conditions could be varied to obtain lower densities. However, this first attempt to use a natural and endemic resource as a coating shows promising results. Overall, the findings demonstrate that candelilla wax is a potential coating for cellulose aerogels, and its behavior could be explored deeply to find a natural alternative and avoid the side effects of using conventional coverings.
Data availability statement
The raw data supporting the conclusion of this article will be made available by the authors, without undue reservation.
Author contributions
AR-M: Data curation, Formal Analysis, Writing–original draft. SR-M: Conceptualization, Funding acquisition, Project administration, Resources, Writing–original draft. JV-B: Investigation, Methodology, Writing–original draft. IN-T: Investigation, Methodology, Writing–original draft. MP-B: Funding acquisition, Resources, Supervision, Writing–original draft, Writing–review and editing. LM-R: Investigation, Methodology, Writing–original draft. GL-G: Funding acquisition, Resources, Supervision, Writing–review and editing. MD: Conceptualization, Funding acquisition, Project administration, Resources, Supervision, Visualization, Writing–review and editing.
Funding
The author(s) declare financial support was received for the research, authorship, and/or publication of this article. This work was supported by the PAPIIT IT100222 grant from DGAPA, UNAM to MP-B, PAPIME PE205524 to GL-G.
Acknowledgments
AR-M would like to thank Conahcyt for their scientific and financial support through the program “Becas Posdoctorales”. The authors would like to thank Karla Eriseth Reyes-Morales for her technical assistance with thermal tests, and Miguel Ángel Canseco Martínez for the support in FTIR analysis.
Conflict of interest
The authors declare that the research was conducted in the absence of any commercial or financial relationships that could be construed as a potential conflict of interest.
The author(s) declared that they were an editorial board member of Frontiers, at the time of submission. This had no impact on the peer review process and the final decision.
Publisher’s note
All claims expressed in this article are solely those of the authors and do not necessarily represent those of their affiliated organizations, or those of the publisher, the editors and the reviewers. Any product that may be evaluated in this article, or claim that may be made by its manufacturer, is not guaranteed or endorsed by the publisher.
Supplementary material
The Supplementary Material for this article can be found online at: https://www.frontiersin.org/articles/10.3389/fmats.2023.1308094/full#supplementary-material
References
Akpomie, K. G., and Conradie, J. (2020). Banana peel as a biosorbent for the decontamination of water pollutants. A review. Environ. Chem. Lett. 18, 1085–1112. doi:10.1007/s10311-020-00995-x
Al-Maqdasi, Z., Joffe, R., Ouarga, A., Emami, N., Chouhan, S. S., Landström, A., et al. (2021). Conductive regenerated cellulose fibers for multi-functional composites: mechanical and structural investigation. Materials 14, 1746. doi:10.3390/ma14071746
Balsamo, V., Calzadilla, N., Mora, G., and Müller, A. (2001). Thermal characterization of polycarbonate/polycaprolactone blends. J. Polym. Sci. Part B Polym. Phys. 39, 771–785. doi:10.1002/1099-0488(20010401)39:7<771::aid-polb1052>3.0.co;2-i
Bayat, A., Aghamiri, S. F., Moheb, A., and Vakili-Nezhaad, G. R. (2005). Oil spill cleanup from sea water by sorbent materials. Chem. Eng. Technol. Industrial Chemistry-Plant Equipment-Process Engineering-Biotechnology 28, 1525–1528. doi:10.1002/ceat.200407083
Budtova, T. (2019). Cellulose II aerogels: a review. Cellulose 26, 81–121. doi:10.1007/s10570-018-2189-1
Cheng, H., Gu, B., Pennefather, M. P., Nguyen, T. X., Phan-Thien, N., and Duong, H. M. (2017). Cotton aerogels and cotton-cellulose aerogels from environmental waste for oil spillage cleanup. Mater. Des. 130, 452–458. doi:10.1016/j.matdes.2017.05.082
Do, N. H., Luu, T. P., Thai, Q. B., Le, D. K., Chau, N. D. Q., Nguyen, S. T., et al. (2020). Advanced fabrication and application of pineapple aerogels from agricultural waste. Mater. Technol. 35, 807–814. doi:10.1080/10667857.2019.1688537
Duan, B., Gao, H., He, M., and Zhang, L. (2014). Hydrophobic modification on surface of chitin sponges for highly effective separation of oil. ACS Appl. Mater. interfaces 6, 19933–19942. doi:10.1021/am505414y
Fan, P., Yuan, Y., Ren, J., Yuan, B., He, Q., Xia, G., et al. (2017). Facile and green fabrication of cellulosed based aerogels for lampblack filtration from waste newspaper. Carbohydr. Polym. 162, 108–114. doi:10.1016/j.carbpol.2017.01.015
Feng, J., Nguyen, S. T., Fan, Z., and Duong, H. M. (2015). Advanced fabrication and oil absorption properties of super-hydrophobic recycled cellulose aerogels. Chem. Eng. J. 270, 168–175. doi:10.1016/j.cej.2015.02.034
Gómez-Lizárraga, K., Flores-Morales, C., Del Prado-Audelo, M., Álvarez-Pérez, M., Piña-Barba, M., and Escobedo, C. (2017). Polycaprolactone-and polycaprolactone/ceramic-based 3D-bioplotted porous scaffolds for bone regeneration: a comparative study. Mater. Sci. Eng. C 79, 326–335. doi:10.1016/j.msec.2017.05.003
Gong, C., Ni, J.-P., Tian, C., and Su, Z.-H. (2021). Research in porous structure of cellulose aerogel made from cellulose nanofibrils. Int. J. Biol. Macromol. 172, 573–579. doi:10.1016/j.ijbiomac.2021.01.080
Hameed, S., Wagh, A. S., Sharma, A., Pareek, V., Yu, Y., and Joshi, J. B. (2022). Kinetic modelling of pyrolysis of cellulose using CPD model: effect of salt. J. Therm. Analysis Calorim. 147, 9763–9777. doi:10.1007/s10973-021-11192-5
Han, N., Johnson, J., Lannutti, J. J., and Winter, J. O. (2012). Hydrogel–electrospun fiber composite materials for hydrophilic protein release. J. Control. release 158, 165–170. doi:10.1016/j.jconrel.2011.09.094
Han, W., Shin, J., and Shin, J. H. (2022). Low-cost, open-source contact angle analyzer using a mobile phone, commercial tripods and 3D printed parts. HardwareX 12, e00327. doi:10.1016/j.ohx.2022.e00327
Hancock, B. C., and Zografi, G. (1994). The relationship between the glass transition temperature and the water content of amorphous pharmaceutical solids. Pharm. Res. 11, 471–477. doi:10.1023/a:1018941810744
He, N., Li, L., Chen, J., Zhang, J., and Liang, C. (2021). Extraordinary superhydrophobic polycaprolactone-based composite membrane with an alternated micro–nano hierarchical structure as an eco-friendly oil/water separator. ACS Appl. Mater. Interfaces 13, 24117–24129. doi:10.1021/acsami.1c03019
Laitinen, O., SuopajaRvi, T., OSterberg, M., and Liimatainen, H. (2017). Hydrophobic, superabsorbing aerogels from choline chloride-based deep eutectic solvent pretreated and silylated cellulose nanofibrils for selective oil removal. ACS Appl. Mater. interfaces 9, 25029–25037. doi:10.1021/acsami.7b06304
Li, Z., Lei, S., Xi, J., Ye, D., Hu, W., Song, L., et al. (2021). Bio-based multifunctional carbon aerogels from sugarcane residue for organic solvents adsorption and solar-thermal-driven oil removal. Chem. Eng. J. 426, 129580. doi:10.1016/j.cej.2021.129580
Li, Z., Shao, L., Ruan, Z., Hu, W., Lu, L., and Chen, Y. (2018). Converting untreated waste office paper and chitosan into aerogel adsorbent for the removal of heavy metal ions. Carbohydr. Polym. 193, 221–227. doi:10.1016/j.carbpol.2018.04.003
Lionetto, F., López-Muñoz, R., Espinoza-González, C., Mis-Fernández, R., Rodríguez-Fernández, O., and Maffezzoli, A. (2019). A study on exfoliation of expanded graphite stacks in candelilla wax. Materials 12, 2530. doi:10.3390/ma12162530
Long, L.-Y., Weng, Y.-X., and Wang, Y.-Z. (2018). Cellulose aerogels: synthesis, applications, and prospects. Polymers 10, 623. doi:10.3390/polym10060623
Lu, C., Lang, X., Yu, Z., Yang, L., Yang, M., and Zhang, Z. (2023). Self-assembled silica aerogel-coated polylactic acid membrane for water-in-oil emulsion separation. J. Sol-Gel Sci. Technol. 105, 694–700. doi:10.1007/s10971-023-06045-6
Mahato, D., Mathur, B., and Bhattacherjee, S. (2013). DSC and IR methods for determination of accessibility of cellulosic coir fibre and thermal degradation under mercerization. Indian J. Fibre and Text. Res. 38, 96–100.
Mishra, B. K., Kumar, P., Saraswat, C., Chakraborty, S., and Gautam, A. (2021). Water security in a changing environment: concept, challenges and solutions. Water 13, 490. doi:10.3390/w13040490
Morán, J. I., Alvarez, V. A., Cyras, V. P., and Vázquez, A. (2008). Extraction of cellulose and preparation of nanocellulose from sisal fibers. Cellulose 15, 149–159. doi:10.1007/s10570-007-9145-9
Mwaikambo, L. Y., and Ansell, M. P. (2002). Chemical modification of hemp, sisal, jute, and kapok fibers by alkalization. J. Appl. Polym. Sci. 84, 2222–2234. doi:10.1002/app.10460
Nguyen, S. T., Feng, J., Le, N. T., Le, A. T., Hoang, N., Tan, V. B., et al. (2013). Cellulose aerogel from paper waste for crude oil spill cleaning. Industrial Eng. Chem. Res. 52, 18386–18391. doi:10.1021/ie4032567
Oh, S. Y., Yoo, D. I., Shin, Y., and Seo, G. (2005). FTIR analysis of cellulose treated with sodium hydroxide and carbon dioxide. Carbohydr. Res. 340, 417–428. doi:10.1016/j.carres.2004.11.027
Picker, K. M., and Hoag, S. W. (2002). Characterization of the thermal properties of microcrystalline cellulose by modulated temperature differential scanning calorimetry. J. Pharm. Sci. 91, 342–349. doi:10.1002/jps.10018
Pircher, N., Carbajal, L., Schimper, C., Bacher, M., Rennhofer, H., Nedelec, J.-M., et al. (2016). Impact of selected solvent systems on the pore and solid structure of cellulose aerogels. Cellulose 23, 1949–1966. doi:10.1007/s10570-016-0896-z
Rafieian, F., Hosseini, M., Jonoobi, M., and Yu, Q. (2018). Development of hydrophobic nanocellulose-based aerogel via chemical vapor deposition for oil separation for water treatment. Cellulose 25, 4695–4710. doi:10.1007/s10570-018-1867-3
Sai, H., Fu, R., Xing, L., Xiang, J., Li, Z., Li, F., et al. (2015). Surface modification of bacterial cellulose aerogels’ web-like skeleton for oil/water separation. ACS Appl. Mater. interfaces 7, 7373–7381. doi:10.1021/acsami.5b00846
Sanguanwong, A., Pavasant, P., Jarunglumlert, T., Nakagawa, K., Flood, A., and Prommuak, C. (2020). Hydrophobic cellulose aerogel from waste napkin paper for oil sorption applications. Nordic Pulp Pap. Res. J. 35, 137–147. doi:10.1515/npprj-2018-0075
Toro-Vazquez, J., Morales-Rueda, J., Dibildox-Alvarado, E., Charó-Alonso, M., Alonzo-Macias, M., and González-Chávez, M. (2007). Thermal and textural properties of organogels developed by candelilla wax in safflower oil. J. Am. Oil Chemists’ Soc. 84, 989–1000. doi:10.1007/s11746-007-1139-0
Toro-Vazquez, J. F., Charó-Alonso, M. A., Pérez-Martínez, J. D., and Morales-Rueda, J. A. (2011). Candelilla wax as an organogelator for vegetable oils—an alternative to develop trans-free products for the food industry. Edible oleogels 2011, 119–148. doi:10.1016/b978-0-9830791-1-8.50009-7
Wang, K., Liu, X., Tan, Y., Zhang, W., Zhang, S., and Li, J. (2019). Two-dimensional membrane and three-dimensional bulk aerogel materials via top-down wood nanotechnology for multibehavioral and reusable oil/water separation. Chem. Eng. J. 371, 769–780. doi:10.1016/j.cej.2019.04.108
Xu, X., Dong, F., Yang, X., Liu, H., Guo, L., Qian, Y., et al. (2019). Preparation and characterization of cellulose grafted with epoxidized soybean oil aerogels for oil-absorbing materials. J. Agric. food Chem. 67, 637–643. doi:10.1021/acs.jafc.8b05161
Yang, S., Xu, Z., Zhao, T., Zhang, T., and Zhao, Y. (2022). Emulsion-templated, hydrophilic and underwater oleophobic PVA aerogels with enhanced mechanical property. Colloids Surfaces A Physicochem. Eng. Aspects 653, 129979. doi:10.1016/j.colsurfa.2022.129979
Yeng, L. C., Wahit, M. U., and Othman, N. (2015). Thermal and flexural properties of regenerated cellulose (RC)/poly (3-hydroxybutyrate)(PHB) biocomposites. J. Teknol. 75, 107–112. doi:10.11113/jt.v75.5338
Yi, L., Xia, Y., Tan, Z., Fang, X., Zhao, L., Wu, H., et al. (2020). Design of tubelike aerogels with macropores from bamboo fungus for fast oil/water separation. J. Clean. Prod. 264, 121558. doi:10.1016/j.jclepro.2020.121558
Yuan, W., Zhang, X., Zhao, J., Li, Q., Ao, C., Xia, T., et al. (2017). Ultra-lightweight and highly porous carbon aerogels from bamboo pulp fibers as an effective sorbent for water treatment. Results Phys. 7, 2919–2924. doi:10.1016/j.rinp.2017.08.011
Zhai, T., Zheng, Q., Cai, Z., Turng, L.-S., Xia, H., and Gong, S. (2015). Poly (vinyl alcohol)/cellulose nanofibril hybrid aerogels with an aligned microtubular porous structure and their composites with polydimethylsiloxane. ACS Appl. Mater. interfaces 7, 7436–7444. doi:10.1021/acsami.5b01679
Zhang, H., Lyu, S., Zhou, X., Gu, H., Ma, C., Wang, C., et al. (2019). Super light 3D hierarchical nanocellulose aerogel foam with superior oil adsorption. J. colloid interface Sci. 536, 245–251. doi:10.1016/j.jcis.2018.10.038
Zhang, W., Liu, Y., Tao, F., An, Y., Zhong, Y., Liu, Z., et al. (2023). An overview of biomass-based Oil/Water separation materials. Sep. Purif. Technol. 316, 123767. doi:10.1016/j.seppur.2023.123767
Keywords: aerogel, cellulose, water remediation, oil, remediation
Citation: Romero-Montero A, Rosas-Melendez SA, Valencia-Bermúdez JL, Nuñez-Tapia I, Piña-Barba MC, Melgoza-Ramírez LJ, Leyva-Gómez G and Del Prado-Audelo ML (2023) Oil/water separation by super-hydrophobic wastepaper cellulose-candelilla wax cryogel: a circular material-based alternative. Front. Mater. 10:1308094. doi: 10.3389/fmats.2023.1308094
Received: 05 October 2023; Accepted: 04 December 2023;
Published: 14 December 2023.
Edited by:
Suresh Sagadevan, University of Malaya, MalaysiaReviewed by:
Svetlana Butylina, LUT University, FinlandJian Yu, Chinese Academy of Sciences (CAS), China
Copyright © 2023 Romero-Montero, Rosas-Melendez, Valencia-Bermúdez, Nuñez-Tapia, Piña-Barba, Melgoza-Ramírez, Leyva-Gómez and Del Prado-Audelo. This is an open-access article distributed under the terms of the Creative Commons Attribution License (CC BY). The use, distribution or reproduction in other forums is permitted, provided the original author(s) and the copyright owner(s) are credited and that the original publication in this journal is cited, in accordance with accepted academic practice. No use, distribution or reproduction is permitted which does not comply with these terms.
*Correspondence: M. L. Del Prado-Audelo, bHVpc2EuZGVscHJhZG9AdGVjLm14