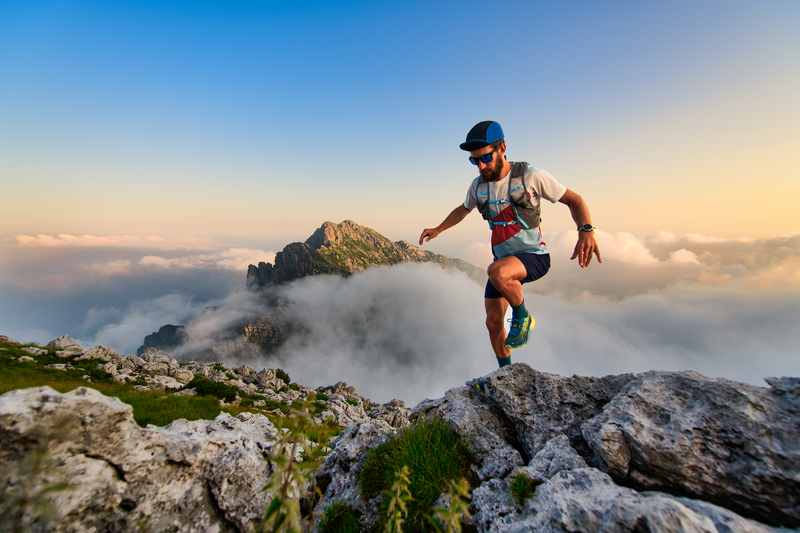
94% of researchers rate our articles as excellent or good
Learn more about the work of our research integrity team to safeguard the quality of each article we publish.
Find out more
MINI REVIEW article
Front. Mater. , 12 January 2024
Sec. Biomaterials and Bio-Inspired Materials
Volume 10 - 2023 | https://doi.org/10.3389/fmats.2023.1285948
Decellularized dermal matrices (dDMs) have emerged as effective biomaterials that can revolutionize regenerative medicine, particularly in the field of wound healing and tissue regeneration. Derived from animal or human skin, dDMs offer great biocompatibility, remarkable biochemistry, and a macromolecular architecture equivalent to the native tissue. Notably, among the biomimetic extracellular matrix (ECM)-based scaffolds, dDMs stand out due to their inherent dermal microenvironment, holding high value for skin regeneration and reconstructive surgery. The integration of dDMs as a biomaterial base for bioinks in advanced manufacturing technologies opens promising avenues for crafting precise, biomimetic tissue engineering (TE) constructs with optimized recellularization properties. This mini review outlines the main sources, differential decellularization techniques applied to dDMs, and their significance intissue engineering and regenerative medicine. It subsequently delves into the different categories of decellularized materials obtained, their unique physical and biochemical attributes, as well as their applications to promote wound healing and regenerating skin and soft tissues. Additionally, the currently available market products based on dDMs are examined and the main outcomes are compared. Finally, the article highlights current barriers in the field and anticipates the future challenges and applications of dDMs-based therapies.
The field of tissue engineering and regenerative medicine has progressively advanced over the years, as researchers strive to restore, repair, and replace damaged tissues and organs that go beyond the body’s natural self-healing capability. The use of stem cells therapies and/or scaffolding materials to recreate the tissue extracellular matrix (ECM) opens up promising possibilities (Yao et al., 2019). Among them, decellularized extracellular matrix (dECM)-based biomaterials have shown increased potential due to their origin in native tissues, which inherently contain numerous structural proteins, glycoproteins, glycosaminoglycans, and cytokines, while maintaining biophysical and topographical cues that can direct cell fate and stimulate its metabolic activity (Gierek et al., 2022; Solarte David et al., 2022). dECMs consist of tissues, from allogeneic or xenogeneic origin, from which the cellular content has been removed (Nakamura et al., 2017; Yang et al., 2022). The removal of cells and cellular debris, results in the absence of an immune response after implantation (Hussein et al., 2016; Kasravi et al., 2023). Thus, they present great biocompatibility and biofunctionality which can boost cell adhesion, proliferation, and differentiation for successful tissue repair and regeneration (Capella-Monsonís and Zeugolis, 2021). Considering the significant impact of the ECM on cell behavior, the unique composition of decellularized matrices/scaffolds makes them advantageous when compared to other polymeric-based biomaterials in tissue engineering (Yao et al., 2019). The properties of biomimetic dECM-based biomaterials, including bioactivity and preservation of native architecture, result in tissue specificity, which allows the creation of biomaterials that closely resemble a target tissue. This level of cell recognition for replicating specific tissues is challenging to attain with polymeric biomaterials. Consequently, dECM biomaterials have demonstrated effectiveness, particularly when it comes to recellularizing scaffolds (Huang et al., 2017; Rana et al., 2017; Taylor et al., 2018).
While various decellularized scaffolds have been developed for creating artificial tissues and organs, such as heart, lung, kidney, and skin (McInnes et al., 2022), this article specifically focuses on the progress made towards the development of decellularized dermal matrices (dDMs) and their applications in regenerative medicine. DDMs are primarily composed of dermal-specific ECM, which is mainly comprised of collagen type I and III, elastin, fibronectin, and laminin (Rippa et al., 2019). The dermis is particularly interesting for skin transplantation, as it exhibits skin tissue-specific properties including high physical strength, flexibility and an extensive vasculature avoiding scar tissue formation, tissue granulation and vascular contraction. Moreover, it is very accessible and abundant, being obtained as a by-product from the agro-food industry perfectly aligning with the sustainability principles (Rajabimashhadi et al., 2023). Thus, the dDMs stand out among the other types of decellularized tissues due to their availability, versatility, structural integrity, and easy handling, being suitable for a wide range of applications in regenerative medicine.
It is possible to divide the dDMs into two groups based on their source: human or animal (Milan et al., 2020; Gierek et al., 2022). While human tissue would be optimal in the interest of avoiding animal diseases spreading and immunogenicity, its availability is very limited (Porzionato et al., 2018; Zhang et al., 2021). Human tissues and organs can be obtained from cadavers or from surgery wastes. The use of xenogeneic tissues has become a widespread practice in current tissue engineering applications, with a large variety of tissues being used (Zhang et al., 2021). The most common animal sources are bovine and porcine. Although they compensate for the lack of human tissue, they can carry the risk of diseases, such as bovine spongiform encephalopathy. Furthermore, there are also limitations due to religious beliefs. Nevertheless, dDMs are considered a versatile biomaterial with potential to be explored in its native state, i.e., maintaining the integrity and architecture to be directly implanted in the human body (Olga et al., 2021). They enclose the possibility of preserving the channels where blood vessels used to be, now available to host new endothelial cells and potentially generate a new vasculature network inside the intact dermal tissue (Gierek et al., 2022). Simultaneously, an intact decellularized dermis provides a scaffold by itself and can be applied directly in surgical procedures. This type of product is used in soft tissue reconstruction surgery, such as reconstructive breast and gynecological procedures and hernia repair, or in wound healing, namely, burn wounds, where it is particularly beneficial due to the lack of compatible donors with abundant and healthy tissue (Gierek et al., 2022; Solarte David et al., 2022). Alternatively, the dDMs have been proposed as powder-like ECMs processed into three-dimensional (3D) scaffolds for tissue engineering strategies (Zhang et al., 2021). These scaffolds can be used alone (Won et al., 2019; Belviso et al., 2020; Bo et al., 2020), or as hybrid matrices by the combination with synthetic/natural materials aiming to upgrade the mechanical properties, add bioactive components or manipulate the stability of regenerative implants (Huang et al., 2021; Jin et al., 2021; Gao et al., 2023). Moreover, recent advancements in the development of dDMs-based living tissue substitutes include recellularization strategies with patient-derived cells, which represented important progresses in clinical practice (Hillebrandt et al., 2019; Sotnichenko et al., 2021).
Given its wide range of clinical applications, regenerative potential, biomimicry, availability, cost-effectiveness and recellularization potential, dDMs are an extremely compeling biomaterial. The increasing literature about dDMs, highlights the necessity to synthesize the knowledge acquired, as well as identify research gaps. For instance, there are no studies on the correlation between the dermal matrix’ sources and its influence on the different tissue engineering and regenerative medicine applications. Thus, this article reviews the recent refining approaches in decellularizing dermal matrices along with the ultimate recellularization strategies for improved clinical practice. The current and future clinical applications of dDMs in regenerative medicine are overviewed, together with the many commercial dDMs products explored in the market.
The dermis, located between the epidermis and the subcutaneous tissue, is obtained from full-thickness and split-thickness sections of skin from a donor source. The dDMs are characterized by its advantageous dermal ECM microarchitecture being obtained from animal or human sources. Since the 90s, dDMs have been proposed for several tissue engineering and regenerative medicine applications, including skin, soft tissues and mucous membranes’ repair (Gierek et al., 2022). Furthermore, the decellularization protocols that allow to obtain cell- and nuclear-free ECMs, are relatively new lab processes but have been improving in the last years in order to preserve as much as possible the tissue to achieve engineered functional constructs (Choudhury et al., 2020). The different sources, decellularization methods and applications are illustrated in Figure 1.
FIGURE 1. Schematic diagram of the various dermal sources, decellularization methods and ultimate applications of dDMs.
In the late 80s the first allogenic composite skin grafts were transplanted in rats as models for treating burn wounds. Positive effects were achieved in terms of inhibiting scar tissue formation reaching a certain level of wound healing (Heck et al., 1985). However, the presence of cellular components on the allogenic composite graft caused immune reaction, which triggered the first research studies on decellularized allogenic dermal matrices (Sedmak and Orosz, 1991). The allogenic dDMs (human-derived) are in most cases obtained from human cadavers as they represent an ethically acceptable source for therapeutic application (Sobti and Liao, 2016; Groth et al., 2021). AlloDermTM stands as a pioneering example of cadaveric dermal allografts in the field of regenerative medicine (Wainwright, 1995; Lattari et al., 1997; Achauer et al., 1998). It revolutionized the approach of tissue grafting by providing a readily available and structurally intact acellular human dermal matrix for several reconstructive and cosmetic procedures (Lynch et al., 2015; Ma et al., 2022). The introduction of this product in the market marked significantly the advancements in tissue engineering, offering a versatile solution for wound care and burns (Ayaz et al., 2021), breast reconstruction (Lynch et al., 2015), and other medical applications (Park et al., 2018). Dermal tissue obtained from abdominoplasties and mammoplasties are also interesting alternative sources of human skin with promising outcomes in terms of decellularization effectiveness and biocompatibility (Nafisi et al., 2017; Louri et al., 2022). Nafisi et al. (Nafisi et al., 2017), proposed in an experimental study an acellular breast dermal matrix (ABDM) using human breast skin from patients subjected to mammoplasties. The application of this novel ABDM showed promising outcomes for breast reconstruction in a sheep model, providing the total coverage of the tissue and a promising prospective to be used clinically in post-mastectomy breast reconstruction. An alternative approach was proposed by Groth et al. (Groth et al., 2021), for developing dDMs by using human skin from abdominoplasties. Authors were able to develop different abdominoplasty skin-derived dDMs as novel dressings for wound closure and scar maturation. From the different decellularization protocols tested, all showed promising outcomes in removing the cellular content of the skin to different extents of purity, collagen preservation, and therapeutic properties, which is interesting to better understand the wound healing mechanisms induced after the application of different abdominoplasty skin-derived dDMs. Thus, given the frequency and relative abundance of resected skin from mammoplasties and abdominoplastic surgeries, these can be considered a viable, safe and sustainable source of dDM for regenerative purposes.
Xenogenic dDMs (animal-derived) are a viable possibility for tissue transplantation being highly available and cost-effective (Kim et al., 2019). These are mainly obtained from porcine and bovine sources (Dadlani, 2021; Petrie et al., 2022), and their differences in terms of collagen fibril architecture and intrinsic mechanical properties can directly influence the application and clinical outcome (Adelman et al., 2014). The porcine dDMs are usually composed of pure collagen types I and III and elastin, used as a stable matrix that does not need to be artificially crosslinked or subjected to additional chemical treatments (Dadlani, 2021). Different researchers have shown that porcine-derived dDMs can positively affect the cell growth of different cell types, e.g., fibroblasts, osteoblasts and endothelial cells, which is crucial for recellularization (Pabst et al., 2016; Papi and Pompa, 2018). Thus, porcine-derived dDMs have been proposed for wound dressing (Chou et al., 2020) and in vascularization strategies (Zhang et al., 2011) for engineering tissues. More recently, the combination of porcine-derived dDMs with bioactive molecules was suggested to increase the antibacterial potential and mechanical properties of these matrices in scaffolding strategies (Wang L. et al., 2020). Bovine-derived dDMs present superior mechanical properties and have motivated their exploitation for certain tissue regeneration strategies, including partial-to full-thickness wound healing (Mansour et al., 2023), diabetic foot ulcers (Lantis et al., 2021), or breast reconstruction (Gravina et al., 2019). Different authors compared the outcomes in using SurgiMedTM fetal bovine and AlloDermTM human cadaveric dDMs for implant-based breast reconstruction, showing no significant differences in complication rates after implantation between the two commonly used products (Butterfield, 2013; Ricci et al., 2016).
Given the successful application of animal-derived dDMs in tissue reconstruction, their use has been expanded to different animal species, including fish (Li et al., 2021), goat (Kumar V. et al., 2022), sheep (Kumar et al., 2022a), rabbit (Kumar N. et al., 2021; Kumar et al., 2022b; Oliveira et al., 2023), or mouse (Han et al., 2016). Rabbit-derived dermal matrices hold an architecture and collagen-based ECM composition close to that of human dermis, which may be advantageous for avoiding post-transplantation complications. Moreover, the similarities between the mechanical properties of decellularized rabbit skin and native human skin can be an asset for tissue engineering strategies involving the use of intact dDMs (Joodaki and Panzer, 2018; Kumar et al., 2022b). Our group has been working on the first systematic study to effectively isolate and decellularize rabbit dermis while preserving its ECM composition and architecture for direct application in regenerative medicine strategies (Oliveira et al., 2023). In our provisional patent application (submitted in XX 2023), different decellularization methods were applied to assess their efficacy in removing the cellular content within the rabbit dermal matrices and the physicochemical properties were characterized and compared to that of human skin. Moreover, the responsible management of rabbit skin as an industrial by-product represents an advantageous aspect in terms of sustainability and circular economy. In a different context, mouse fetal-derived dDMs were explored for wound healing applications and also showed structural (i.e., collagen density and orientation) and biomechanical (i.e., stiffness) similarities to the normal human adult dermis (Han et al., 2016). Thus, the enormous potential of dDMs is constantly being explored into different regenerative applications. Nonetheless, further research is needed to more effectively assimilate the unique structural characteristics inherent to each dermal source. This customization is essential to align with the precise requirements of the target tissue and scaffolding methodologies.
A decellularization process has as main goal to remove the higher amount of cells and cell debris possible, while retaining the surrounding native protein-based ultrastructure. The resulting matrix has to be capable of being sterilized and used as a functional biomaterial, in close contact with the living tissue. Presently, the established benchmarks for determining an appropriate level of cell removal have been outlined by Crapo and Badylak et al (Crapo et al., 2011). These guidelines stipulate that the maximum permissible presence of DNA residues should not exceed 50 ng of double strained DNA (dsDNA) per mg of dry ECM weight. Moreover, the fragment length is advised to remain below 200 bp. Lastly, the absence of discernible nuclear material in tissue sections must be validated through staining with 4′,6-diamidino-2-phenylindole (DAPI) or hematoxylin and eosin (H&E) stain. To obtain a decellularized matrix there are plenty of methods described in the literature (Yang et al., 2022). However, increasing efforts have been made to improve the efficacy of the processes and the environmental impact of the classic standard methods, by creating innovative technologies capable of removing the cellular content of the tissues to acceptable immunological levels, and while preserving the 3D structure, architecture and matrix components for further recellularization and/or direct implantation strategies (Choudhury et al., 2020). Thus, according to the final purpose of the decellularized matrices and the characteristics of the tissue being processed, different decellularization methods and processing steps can be selected.
The decellularization protocols typically include three main stages: i) initial tissue pre-processing and pre-treatments, ii) the core decellularization process itself, and iii) post-processing, including sterilization (Duarte et al., 2022).
The foremost stage of tissue processing typically includes the excision of undesirable tissue layers, such us adipose tissue, and application of pre-treatments to enhance the permeability of the tissue and facilitate the decellularization agents’ action. For skin-specific decellularization protocols, pre-treatments like de-epithelization and hair follicle removal are performed. De-epithelization consists in the removal of the epidermis and is a crucial step to isolate the dermis and obtain a dDM (Mendibil et al., 2020). Chemical or biological reagents, alongside with mechanical removal, can be used at this stage, ensuring epidermis removal with minimal dermal damages. Hair removal can be done by shaving and subsequent hair follicle removal during the de-epithelization and decellularization treatments (Heath, 2019; Olga et al., 2021).
Afterwards, the decellularization steps encompasses cell lysis and removal. The traditional methods can be broadly categorized into three groups: biological, chemical, and physical treatments.
Biological interventions hinge upon enzymes, such as proteases, DNases, and RNases, that break cell adhesive proteins and digest residual genetic material. While these enzymes do not significantly affect the matrix’s collagen content, a prolongated exposure can weaken collagen fibers and eliminate laminin, fibronectin, elastin, and glycosaminoglycans (GAGs) (Heath, 2019; Olga et al., 2021).
Chemical treatments include tissue immersion in solutions containing acid or alkaline agents, alcohol, chelators, or detergents. Acidic solutions, like peracetic acid, and alkaline solutions, such as ammonium hydroxide, sodium sulfide, and calcium hydroxide, can solubilize cellular components and remove DNA by breaking nucleic acids (Capella-Monsonís and Zeugolis, 2021). However, they might also denature ECM components, particularly GAGs, and reduce tissue strength. Peracetic acid has shown to be more effective in disrupting cells while preserving important biomolecules and growth factors, namely, growth factor-β, which is essential for fibroblast and endothelial cell growth (Heath, 2019). Alcohols can lyse cells through dehydration, aiding the removal of residual DNA and solubilization of lipids (Ventura et al., 2019), but they can also decrease the levels of structural proteins of the ECM. Chelating agents, such as EDTA, disrupt cell adhesion by sequestering divalent cations needed for cell binding, such as calcium and magnesium (Emami et al., 2021). Finally, detergents (ionic, non-ionic, or zwitterionic) affect lipid-lipid and lipid-protein interactions, influencing cell membrane integrity. On the other hand, they can have a significant impact on the content of GAGs, laminin, and fibronectin, as well as affect collagen integrity (White et al., 2017). Triton X-100, benzalkonium chloride, and polyethylene glycol (TBP) stands out for being less harsh on the ECM components (Gupta et al., 2018).
Physical methods utilize temperature, pressure, or force to lyse the cells, and are often combined with other approaches to optimize agent distribution. Additionally, they can be used to facilitate the removal of cell debris and aid in rinsing off chemical or biological agents. Freeze-thaw cycles are applied to generate intracellular ice crystals capable of enhancing cell lysis and detachment (Zhang et al., 2022). This method can create a more porous structure in the tissue, promoting the diffusion of the decellularization agents. When increasing the tissue size up to whole organs the use perfusion methods become essential for assuring decellularization. These allow for the entrance of chemical/biological agents on the inner sections of tissues for cell debris removal, using the vasculature channels, while preserving the tissue architecture. Ultimately, the mechanical agitation method is typically applied in smaller and fragile organ sections submerged in decellularization solutions (Rabbani et al., 2021). Regardless of the applied decellularization method, key factors like pH and temperature, are critical in determining the efficiency of the treatment and the level of ECM damage (Choudhury et al., 2020). Moreover, post-decellularization treatments, including wash cycles, sterilization, and shelf-life are critical for a successful implantation without acute reactions and inflammation. Thus, the success of a standard decellularization is always dependent on the combined efficacy of different physical, chemical and biological methods, while creating the minimum impact on the ECM integrity. Although standard methods have proven to be effective in cell removal, they are not optimal in preserving the ECM components.
Newer approaches are emerging to improve tissues’ decellularization focusing on gentler, biofriendly, residueless, targeted, and specific methods and protocols (Zhang et al., 2023). For instance, there is an ongoing exploration for detergent-free methods due to the concerns about their adverse effects on the ECM and difficulty to wash them, despite their great effectivity in removing cells (Duarte et al., 2022). In this sense, Bera et al. (Bera et al., 2022), created a detergent-free minimalistic approach for goat dermis decellularization. Their protocol was based on the utilization of hypotonic/hypertonic sodium chloride solutions and was compared with three established methods, trypsin/Triton X-100, trypsin/SDS/Triton X-100, and trypsin/NaOH. The authors’ protocol showed to be equally successful in removing the cellular content, although it suffered a decrease in the collagen content. This method was able to better preserve the GAGs as compared the traditionally established methods and supported excellent cell attachment, proliferation, stretching, and migration. Farrokhi et al. (Farrokhi et al., 2018), also explored a different detergent-free murine dermis decellularization protocol based on the utilization of latrunculin B. This natural compound derived from marine sponges is known for its ability to disrupt actin cytoskeletal of cells and can be used for decellularization purposes (Crapo et al., 2011; Ramalingam and Häkkinen, 2023). The authors compared their protocol with detergent-based methods and concluded that their method was the only one able to effectively decellularize the tissue while preserving GAGs, elastin content, and maintain better biomechanical properties. Although this reagent can be a good option to substitute detergents, its removal from the tissue must be efficient, due to the inherent toxicity of latrunculin B. For this reason, the author’s protocol involves a significant number of washing step, which appeared to be successful based on their good results of cytocompatibility and biocompatibility.
A highly promising technique is the decellularization through supercritical CO2 (scCO2). This fluid is characterized by high diffusivity, and low density, viscosity and surface tension, leading to a high mass transfer capability and a potent solvent strength (Wang C.-H. et al., 2020; Duarte et al., 2022). Although the precise mechanism of scCO2 decellularization remains uncertain, the most relevant hypothesis is by supercritical extraction with a contribution from the high pressure which is able to induce cell bursting. ScCO2 has affinity for lipids, but in the cell membrane and in nucleic acid there are polar molecules that can be corrupted by the use of co-solvents (e.g., ethanol) enhancing the fluid’s solvating power (Chou et al., 2020; Duarte et al., 2022). This method has important advantages, i.e., low EMC damages, is non-toxic, environmentally friendly, does not leave residues, odors and is efficient in eliminating chemical residues (Chou et al., 2020; Giang et al., 2022). Wang et al. (Wang C.-H. et al., 2020), have already produced a porcine acellular dermal matrix using scCO2 as decellularization method, resulting in a non-toxic and biocompatible material capable of accelerating wound healing in a full-thickness in vivo model. Another study conducted by Giang et al. (Giang et al., 2022), proposed the decellularization of human dermis using scCO2 with ethanol as co-solvent. Even though they did not reach the acellular conditions establish by Crapo and Badylak et al. (Crapo et al., 2011), of less than 50 ng of dsDNA per mg of dry sample, they were able to remove the majority of the cells. The resultant matrix demonstrated to have excellent biomechanical properties, showing similarities to the native skin, and a very promising content of growth factors and anti-inflammatory cytokines (Giang et al., 2022). These results confirm the potential of this technology to remove cells without substantially alter the ECM’s bioactivity and biofunctionality.
Bioreactors are a useful tool that can enhance the reproducibility, automatization, and scale-up of decellularization protocols. Perfusion and immersion-agitation decellularization bioreactors are the prime contenders, existing a variety of parameters that can be adjusted to optimize the process (Choudhury et al., 2020).
Perfusion-based bioreactors have been highly explored in the literature and are mainly used to decellularize whole organs. An example of a successful perfusion bioreactor was constructed by Poornejad et al. (Poornejad et al., 2016), for porcine kidney decellularization. This equipment allowed the optimization of the exposure time to harsh detergents, namely, SDS, to maximize its potential for tissue decellularization while minimize its side effects. This method resulted in a 30,5% increase in the preservation of GAGs and 22% increase in the preservation of collagen in comparison to the control method.
Regarding the immersion-agitation bioreactors, these are easier to use, cost-effective, and simpler, allowing to decellularize several samples at once. Nevertheless, they can be more susceptible to shear forces and collisions. Carbonaro et al. (Carbonaro et al., 2020), presented a novel 3D printed sample holder for agitation-based decellularization of multiple specimens, designed to increase the homogeneity, reproducibility, and efficiency of the decellularization process in such bioreactors. The sample holder loaded with the tissue samples was immersed in the decellularization reagent solution within a beaker and placed on a magnetic stirrer that spinned the whole apparatus. Velocity parameters were able to be controlled and standardized using human skin samples for comparing the procedure with and without the sample holder. This method was able to reduce the protocol time from 36 h to 24 h, obtaining a highly preserved and homogeneous ECM.
Currently, there are already a few decellularization bioreactors being commercialized, namely, Harvard Apparatus: ORCA and the Ebers Tubular Chamber Bioreactor (Choudhury et al., 2020). When considering bioreactors for dermal decellularization, there remains a need for deeper exploration to bridge the gap between laboratory-scale setups and scalable platforms.
The utilization of dDMs as scaffolding structures holds an enormous potential in the tissue engineering and regenerative medicine field, capitalizing the unique properties of the acellular dermis as a biomaterial for tissue repair and regeneration. Moreover, there is a range of possibilities in its use as an intact matrix or as a powder further processed into a scaffold through different and innovative technologies (Groth et al., 2021; Kim et al., 2021). Standard or more innovative scaffolding strategies can be applied to process dDMs to use it as a biomaterial or for further enhancement of biomechanical and bioactivity performance (Kim et al., 2018; Chou et al., 2020). The current literature has been exploring the clinical utility of dDMs across a broad range of applications (Debels et al., 2015; Gierek et al., 2022; Petrie et al., 2022), examining their successful integration into medical practices and their potential to revolutionize patient care. Table 1 summarizes some examples of dDMs found in the literature, their dermis source, decellularization methodologies, scaffolding strategies and tissue regeneration applications.
TABLE 1. List of examples of dDMs developed found in the literature, their source, decellularization method, scaffolding strategy, and application.
The dDMs are characterized by the advantageous ECM microstructure in terms of three-dimensionality, fibrous architecture and mechanical properties. For this reason it has been highly explored as an intact full-matrix applied to repair skin and other soft tissue defects. In fact, its potential has been recognized as a regenerative tissue matrix with different sources of exploitation (human and animal) and decellularization strategies capable of promoting an homogenous cell removal and maintain intact the ECM structural properties (Choudhury et al., 2020). Such capacity is detrimental to guarantee the quality of the dDMs to be directly used as a scaffold in tissue engineering and regenerative medicine strategies (Petrie et al., 2022). Tissue engineered skin scaffolds are intended to stimulate tissue healing, re-epithelialization and neovascularization. In this regard, the choice of an appropriate scaffold architecture is important. From the different manufacturing techniques used for scaffolds’ processing, freeze-drying and chemical cross-linking methods are some of the most used, being the latter capable of providing enhanced mechanical strength to the processed biomaterials (Heath, 2019). However, freeze-dried scaffolds not always represent the desired tissue architecture, while some of the crosslinked methods can negatively influence clinical results (Zhang et al., 2023). For instance, Melman el at compared the biocompatibility of five different biologic scaffolds, between them 3 were examples of commercially available crosslinked and non-crosslinked dDMs (AlloDerm, Permacol, and Strattice), after being used in a porcine model of ventral hernia repair (Melman et al., 2011). The author’s data suggested that crosslinking has a negative influence in cellular infiltration, ECM deposition, scaffold degradation, and neovascularization, while the integrity and strength of the repair site were not significantly impacted by the crosslinking process.
Non-crosslinked materials are in principle more prone to interact with the biological environment and stimulate cells for ECM deposition and neovascularization, which makes them a better choice to be incorporated into the tissue (Melman et al., 2011). After decellularization, dermal tissue scaffolds hold unique properties as they contain all the ECM components of the dermis and retain an intact dermal structure to become integrated into the native tissue and thus increasing biocompatibility for an accelerated regenerative process (Tognetti et al., 2021; Patel et al., 2022). Several other decellularized dermal full-matrices are commercially available, some derived from human cadavers, e.g., GraftJacket (Brigido, 2006) and AlloMax (Chauviere et al., 2014), others produced from porcine dermis (e.g., Strattice), or bovine dermis (e.g., MatriDerm) (Mirastschijski et al., 2013), which confirms their potential and clinical utility for several surgical specialties. As example, three distinct human-based acellular dermal matrices were proposed for implant-based breast reconstruction, showing distinct and yet appropriate incorporation into the host tissue and a favorable environment for cell infiltration and collagen deposition within the dECMs (Chien et al., 2021). In a different approach, human-derived acellular dermal matrices were conjugated with split thickness skin grafts as an additional thick layer for promoting support and wound healing in I-stage exposed tendons in the foot (Melandri et al., 2020). The acellular full-matrices provided support and improved the mechanical and functional properties of the split thickness skin grafts for a more efficient coverage of the exposed tendons. In a recent study (Abbasnezhad et al., 2023), an acellular fish skin was chemically modified using different rations of Ethyl-3-(3-dimethylaminopropyl) carbodiimide (EDC) in order to improve the mechanical properties, denaturation and degradability of the intact fish dermis as a biological scaffold for tissue engineering applications. Authors showed that it is possible to chemically modify the acellular matrices and still maintain intact their dermal structure/architecture for improved regenerative purposes.
Taken together, these studies demonstrate that the decellularized dermis has inherent advantageous ECM microstructure that can be preserved or minimally affected for specific tissue healing and regenerative approaches. This is demonstrated by the numerous commercially available products with remarkable clinical achievements (Table 2). Nevertheless, additional investigations remain imperative to refine decellularization techniques, aiming to generate acellular dermal matrices with intact and open microstructure suitable for recellularization in different tissue engineering contexts.
TABLE 2. List of dDM-based products, source, processing methodologies, storage temperature, shelf-life and intended applications.
The dDMs can be a source of powdered matrix for further processing into specific scaffold architectures for different applications (Solarte David et al., 2022). The advantage is the possibility of integrating dDMs in advanced manufacturing approaches, such as 3D bioprinting, for the development of functional bioinks (Kabirian and Mozafari, 2020).
3D bioprinting is an advanced technology that entails the fabrication of intricate three-dimensional structures through the sequential deposition of bioinks, facilitating cell viability, tissue integration, and functional restoration for enhanced regenerative therapies (Vijayavenkataraman et al., 2018; Zhe et al., 2023). The precise arrangement of cells, growth factors, and biomaterials enables the generation of functional tissues possessing specific desired properties. The selection of the bioink is a critical consideration in this process, as it is heavily reliant on the target tissue or organ (Kabirian and Mozafari, 2020). Scaffolds can be printed using a combination of dDMs, biomaterials, biomolecules, and cells, providing a versatile and tailored approach for tissue regeneration (Vijayavenkataraman et al., 2018).
Porcine skin has emerged as the primary source for producing dDMs-based bionks intended for application in skin regeneration. The dDMs-based bioinks demonstrate a substantial content of essential components, effectively providing cues which are essential for cellular activities, despite of the harshness of the prior decellularization and drying processes, employed to mitigate immune responses (Lee et al., 2020). Moreover, the preservation of crucial biological cues and the inclusion of pivotal cell adhesion proteins, notably fibronectin, collagen, and laminin, within decellularized dECMs assume an indispensable role in fostering a suitable environment for cell attachment, proliferation, and the facilitation of tissue regeneration (Zhang et al., 2022). This biological signaling assumes supreme significance when dECMs are conjoined in bioinks with alternative biomaterials lacking these specific biological attributes. The work of Won et al. (Won et al., 2019), illustrates well this potential, by developing a 3D bioprinting where human dermal fibroblasts (HDFs) were incorporated into a bioink based on dDMs from porcine skin to produce 3D layer-by-layer constructs. The presence of dDMs in the bioink facilitated the viability of HDFs post-printing and provided an optimal microenvironment that was demonstrated by heightened gene expression related to skin morphology. These findings suggest that the inclusion of dDMs in the bioink enhances the bioprinted constructs’ capacity to support HDFs and fosters their functional behavior.
Furthermore, dDMs have demonstrated promising outcomes when combined with other biomaterials. For instance, Jin et al. (Jin et al., 2021), successfully generated a functional full-thickness skin model to act as a functional skin substitute by integrating dDMs with gelatin methacrylamide (GelMA). GelMA, that possess adjustable mechanical characteristics, was utilized as a structural bioink to enhance the suboptimal printability and mechanical attributes exhibited by dDMs. Employing a 3D bioprinting methodology, the researchers not only facilitated cell viability, proliferation, and epidermis reconstruction in vitro but also observed significant wound healing and re-epithelization in vivo. The findings from these studies represent a noteworthy advancement in the field of functional skin substitutes, underscoring the potential of dDMs-based bioinks in tissue regeneration.
The integration of 3D bioprinting with dDMs has facilitated the development of pertinent in vitro skin models, driven by several factors: 1) the escalating regulatory demands and prohibition of animal experimentation for substance testing; 2) the inherent inaccuracies in predicting human responses due to genetic disparities between humans and animals; and 3) the growing emphasis on personalized medicine approaches, where therapies are tailored based on an individual’s genetics, gender, age, anatomy or other relevant characteristic (Benam et al., 2015; Litman, 2019; Schmidt et al., 2020). In response to these challenges, there has been a concerted effort to engineer in vitro human skin models, aiming to address these limitations and provide more physiologically relevant platforms for testing and studying skin-related processes and treatments (Schmidt et al., 2020). In a recent study, Kim et al. (Kim et al., 2021), used dDMs-based bioinks to engineer a diseased human skin equivalent with the aim of replicate the pathophysiological hallmarks associated with type 2 diabetes in an in vitro setting. This innovative model successfully captured the cellular and functional abnormalities observed in diabetic skin, presenting a valuable and physiologically relevant platform for investigating disease progression, discerning potential therapeutic targets, and evaluating candidate drugs in a controlled and patient-specific manner. Thus, the utilization of more mimetic in vitro models holds a considerable promise for advancing the knowledge of several pathologies and developing targeted interventions for this condition.
Notwithstanding these notable accomplishments, the clinical translation of dDMs-based bioinks from xenogeneic sources requests diligent attention to several concerns and challenges. Of particular significance are the ethical considerations and regulatory challenges arising from the use of xenogenic tissue, which remains a prominent apprehension. The regulatory approval process for xenogeneic tissue-based products is often characterized by heightened complexity and prolonged duration, primarily attributable to meticulous scrutiny necessitated by safety and ethical considerations. Consequently, these exigent evaluations may introduce delays in the developmental timeline and subsequent availability of such products (Schuurman, 2015). Furthermore, the composition of xenogeneic tissues may exhibit substantial disparities in relation to human tissues, rendering the precise alignment of xenogeneic matrix properties with those of the intended human tissue a considerable challenge. As an alternative, researchers have explored the implementation of human dDMs-based biomaterials in 3D bioprinting endeavors (Belviso et al., 2020; Jorgensen et al., 2020). By incorporating human-derived dDMs, these approaches seek to circumvent the challenges associated with xenogenic sources and hold promise for overcoming ethical, regulatory and matrix composition obstacles in tissue regeneration applications. Jorgensen et al. (Jorgensen et al., 2020), demonstrated noteworthy advancements in the field of 3D bioprinting by showcasing enhanced biological, physical, and printability properties of fibrinogen hydrogel through supplementation with human dDMs. Their study highlights the potential of dDMs as a valuable component for optimizing bioink formulations in 3D bioprinting applications, thereby contributing to the development of improved tissue-engineered constructs.
The potential of dDMs as a powder-based biomaterial has been underscored by their capacity to preserve essential components of the ECM while being able to perform as a bioink in 3D bioprinting applications. This characteristic renders dDMs particularly attractive for creating biomimetic structures to the integration of stem cells and bioactive materials, as well as providing suitable biomechanical environment that facilitates tissue regeneration. Taken together, these attributes position dDMs as a compelling candidate for advancing the field of 3D bioprinting and hold great promise for fostering innovative approaches in tissue regeneration. However, it is crucial to emphasize the diminished quantity of scientific research in this field and the importance of having further pre-clinical evidence to solidify the role of dDMs as a promising candidate for regenerative medicine applications.
The use of allograft and xenograft skin substitutes has been widely accepted in the clinics due to their preserved dermal architecture, high collagen, and elastin content. There are some examples of commercially available scaffolds from dDMs currently available (Table 2). These products are sold all over the world for use in surgery procedures like breast reconstruction or in the repair of soft tissues such as tendon and gingival tears. However, the majority of their target applications are for acute and chronic wound regeneration. These matrices can be typically acquired from a variety of sources.
The majority of commercially available tissues come from humans (i.e., allografts), while a smaller number come from other animals (i.e., xenografts), primarily porcine and then bovine. The difference in tissue origin can be associated with different clinical outcomes for the same condition. Commercial human dDMs (n = 312) were found to be more effective in healing patients with diabetic foot ulcers, with shorter mean healing times and a higher likelihood of complete healing than when compared to a standard operating care. These findings suggest that human dDMs can improve diabetic foot ulcer outcomes, reduce healthcare burden by accelerating healing, and reduce treatment duration (Luthringer et al., 2020). In complicated ventral hernias, bovine and porcine-based dDMs had somewhat higher recurrence rates than typical synthetic mesh repairs (Van Orden et al., 2022). Another study compared patients who received porcine or bovine dDMs reinforcement and found that the bovine dDM was associated with fewer wound problems and recurrences (Lightfoot et al., 2023). This could be related to the acute inflammation associated with the porcine dDM. However, further research is needed to fully understand and evaluate the risks and benefits of using xenografts as a more cost-effective alternative to human dDMs (Saricilar and Huang, 2021).
Piscean sources have also been explored, since it presents a cost-effective alternative with some architectural similarities to human skin (Rakers et al., 2010). Moreover, it has been reported properties of antiviral, antibacterial (Mil-Homens et al., 2012; Imai, 2015), inflammation regulation (Serhan, 2014) and pain management (Ko et al., 2010; Escudero et al., 2015) associated with the presence of mega-3 polyunsaturated fatty acids combined with the reduced risk of viral and prion transmission. Kerecis™ Omega3 Wound, fish skin dDM, presents preliminary clinical results that indicate improved wound healing for patients previously treated with conventional wound treatment (vacuum therapy) (Dorweiler et al., 2018).
The processing methods used to create these dDMs can impact their overall quality and performance as skin substitutes. Additionally, the choice of processing methodology may also affect the immunogenicity and biocompatibility of the dDMs, further influencing its clinical outcomes. Therefore, understanding the differences in processing methodologies is crucial when selecting the most suitable dDMs for specific applications in the medical field. These tissues undergo sterilization processes such as gamma or e-beam irradiation to ensure their safety for use in patients. Additionally, some of these decellularized grafts are produced aseptically to minimize the risk of contamination. Moreover, market-ready dDMs also vary in the decellularization protocol applied; for instance, MatrACELL-processed comprises a patent-protected decellularization method that includes the use of N-Lauroyl sarcosinate, recombinant endonuclease, and antibiotics (Wolfinbarger Jr et al., 2004). These differences can lead to different outcomes in clinical trials. A prospective cohort study evaluated the outcomes of implant-based breast reconstruction using dDMs (Sorkin et al., 2017; Kumar N. G. et al., 2021). Study participants received one of four dDMs brands and were compared to the control group. Results revealed that patients who received FlexHD and AlloMax had significantly higher rate of complications in explantation, reoperation, and infections 2 years after surgery when compared to patients who received SurgiMend, AlloDerm, or no dDM. Additional data supports differences in the safety profiles of dDMs brands, even among the dDMs from the same origin, that could be related to processing methodology; however, additional clinical data are required to assess benefits and risks (Michelotti et al., 2013; Liu et al., 2014; Martin et al., 2014; Ranganathan et al., 2015).
This highlights the importance of conducting further research to gather comprehensive data on the long-term outcomes and potential complications associated with different dDMs brands. By conducting further research and gathering this comprehensive data, healthcare professionals will have the necessary information to make informed decisions. This will also provide valuable data for researchers to further improve or produce new dermal matrices, ensuring the development of safer and more effective products.
The field of tissue engineering and regenerative medicine has witnessed continuous progress as researchers strive to address tissue and organ damage that exceeds the body’s natural healing capacity. While stem cell therapies present relevant clinical outcomes for the treatment of several challenging diseases and injuries, the rehabilitation and rebuilding of extensive damaged or diseased tissues or organs calls for the adoption of sophisticated tissue engineering approaches and innovative biomaterials. These technologies are designed to effectively mimic the intricate native tissue architecture and provide the necessary mechanical, biochemical, and topographical cues for successful integration and functional restoration. dECM-based biomaterials, derived from allogeneic or xenogeneic tissues, offer this possibility by providing an immunogenically safe extracellular environment. While various decellularized scaffolds have been developed, dDMs are amongst the most used due to its processing easiness and application versatility, being used in surgical procedures for several regenerative applications.
Commercially available options demonstrate the success of native dermal dECM materials, which show high host cells’ infiltration being employed in burn wound treatment, soft tissue defects, prosthetic coverage, and even pelvis or abdominal wall reconstruction. Human, porcine, bovine, fish, rabbit, and mouse-derived acellular dermal matrices have all shown promise in wound healing applications, maintaining stable dermal architecture for scarless repair and regeneration requirements. The potential of dDMs extends beyond direct application, as they can be transformed into powdered material for specialized scaffold architectures using advanced manufacturing techniques such as 3D bioprinting. This has allowed for the creation of better replicating skin models, potentially decreasing reliance on animal testing and opening avenues for personalized medicine applications.
Collectively, the herein presented examples underline the advantageous composition and microstructure of decellularized dermis for tissue healing and regeneration. Newer approaches such as detergent-free methods and the use of scCO2 are emerging to improve tissues’ decellularization. This also includes the use of bioreactors to enhance the reproducibility, automatization, and scale-up of these decellularization protocols, including whole organ decellularization. Sterilization assurance is also an important aspect to take into consideration mostly when considering of-the-shelf products based on dDMs and the fact that most of the standard sterilization processes will damage ECM-based products. The use of scCO2 for the simultaneous decellularization and sterilization of biological tissue is expanding this technology to a new world of possibilities in ECM processing. To validate decellularization some metrics have been commonly accepted as basis to verify the absence of cells and cell nuclei, and to assess the DNA reduction. However, no unified and quantitative standard criteria have yet been officially established to evaluate ECM decellularization and post-processing. Therefore, further multidisciplinary investigation is essential to establish the metrics and generate meaningful comparative results that can clearly point out the best processes towards better preserved and safer ECMs. This is mandatory to facilitate the translation of the most promising technologies from lab to the clinics.
MR: Writing–original draft, Writing–review and editing. IS: Writing–original draft, Writing–review and editing. JC: Writing–original draft, Writing–review and editing. VR: Supervision, Writing–original draft, Writing–review and editing. AO: Supervision, Writing–original draft, Writing–review and editing.
The author(s) declare that no financial support was received for the research, authorship, and/or publication of this article. National Funds from Fundação para a Ciência e a Tecnologia (FCT), through the project UID/Multi/50016/2020.
Authors acknowledge for the Junior Researcher contract attributed to VR by the BE@T–Bioeconomy for Textiles and Apparel, investment TC-C12-i01—Sustainable Bioeconomy, funded through the Recovery and Resilience Program (PRR). Authors are also grateful for the distinction attributed by FCT to JC with an individual Junior Researcher contract (2022.02781.CEECIND), under the Individual Scientific Employment Stimulus, and to IS for the attribution of a Doctoral Research Grant (2021.05919.BD).
The authors declare that the research was conducted in the absence of any commercial or financial relationships that could be construed as a potential conflict of interest.
All claims expressed in this article are solely those of the authors and do not necessarily represent those of their affiliated organizations, or those of the publisher, the editors and the reviewers. Any product that may be evaluated in this article, or claim that may be made by its manufacturer, is not guaranteed or endorsed by the publisher.
Abbasnezhad, S., Biazar, E., Aavani, F., Kamalvand, M., Heidari Keshel, S., and Pourjabbar, B. (2023). Chemical modification of acellular fish skin as a promising biological scaffold by carbodiimide cross-linker for wound healing. Int. Wound J. 20, 1566–1577. doi:10.1111/iwj.14012
Achauer, B. M., Vanderkam, V. M., Celikoz, B., and Jacobson, D. G. (1998). Augmentation of facial soft-tissue defects with Alloderm dermal graft. Ann. plastic Surg. 41, 503–507. doi:10.1097/00000637-199811000-00009
Adelman, D. M., Selber, J. C., and Butler, C. E. (2014). Bovine versus porcine acellular dermal matrix: a comparison of mechanical properties. Plastic Reconstr. Surg. Glob. Open 2, e155. doi:10.1097/GOX.0000000000000072
Ayaz, M., Najafi, A., and Karami, M. (2021). Thin split thickness skin grafting on human acellular dermal matrix scaffold for the treatment of deep burn wounds. Int. J. Organ Transplant. Med. 12, 44–51.
Belviso, I., Romano, V., Sacco, A. M., Ricci, G., Massai, D., Cammarota, M., et al. (2020). Decellularized human dermal matrix as a biological scaffold for cardiac repair and regeneration. Front. Bioeng. Biotechnol. 8, 229. doi:10.3389/fbioe.2020.00229
Benam, K. H., Dauth, S., Hassell, B., Herland, A., Jain, A., Jang, K.-J., et al. (2015). Engineered in vitro disease models. Annu. Rev. Pathology Mech. Dis. 10, 195–262. doi:10.1146/annurev-pathol-012414-040418
Bera, A. K., Sriya, Y., and Pati, F. (2022). Formulation of dermal tissue matrix bioink by a facile decellularization method and process optimization for 3D bioprinting toward translation research. Macromol. Biosci. 22, 2200109. doi:10.1002/mabi.202200109
Bo, Q., Yan, L., Li, H., Jia, Z., Zhan, A., Chen, J., et al. (2020). Decellularized dermal matrix-based photo-crosslinking hydrogels as a platform for delivery of adipose derived stem cells to accelerate cutaneous wound healing. Mater. Des. 196, 109152. doi:10.1016/j.matdes.2020.109152
Brigido, S. A. (2006). The use of an acellular dermal regenerative tissue matrix in the treatment of lower extremity wounds: a prospective 16-week pilot study. Int. wound J. 3, 181–187. doi:10.1111/j.1742-481X.2006.00209.x
Butterfield, J. L. (2013). 440 Consecutive immediate, implant-based, single-surgeon breast reconstructions in 281 patients: a comparison of early outcomes and costs between SurgiMend fetal bovine and AlloDerm human cadaveric acellular dermal matrices. Plastic Reconstr. Surg. 131, 940–951. doi:10.1097/PRS.0b013e3182865ab3
Capella-Monsonís, H., and Zeugolis, D. I. (2021). Decellularized xenografts in regenerative medicine: from processing to clinical application. Xenotransplantation 28, e12683. doi:10.1111/xen.12683
Carbonaro, D., Putame, G., Castaldo, C., Di Meglio, F., Siciliano, K., Belviso, I., et al. (2020). A low-cost scalable 3D-printed sample-holder for agitation-based decellularization of biological tissues. Med. Eng. Phys. 85, 7–15. doi:10.1016/j.medengphy.2020.09.006
Chauviere, M. V., Schutter, R. J., Steigelman, M. B., Clark, B. Z., Grayson, J. K., and Sahar, D. E. (2014). Comparison of AlloDerm and AlloMax tissue incorporation in rats. Ann. plastic Surg. 73, 282–285. doi:10.1097/SAP.0b013e31827a2d00
Chien, P. N., Zhang, X. R., Nilsu, D., Faruq, O., Le Thi Van, A., Nam, S.-Y., et al. (2021). In vivo comparison of three human acellular dermal matrices for breast reconstruction. vivo 35, 2719–2728. doi:10.21873/invivo.12556
Chou, P.-R., Lin, Y.-N., Wu, S.-H., Lin, S.-D., Srinivasan, P., Hsieh, D.-J., et al. (2020). Supercritical carbon dioxide-decellularized porcine acellular dermal matrix combined with autologous adipose-derived stem cells: its role in accelerated diabetic wound healing. Int. J. Med. Sci. 17, 354–367. doi:10.7150/ijms.41155
Choudhury, D., Yee, M., Sheng, Z. L. J., Amirul, A., and Naing, M. W. (2020). Decellularization systems and devices: state-of-the-art. Acta biomater. 115, 51–59. doi:10.1016/j.actbio.2020.07.060
Crapo, P. M., Gilbert, T. W., and Badylak, S. F. (2011). An overview of tissue and whole organ decellularization processes. Biomaterials 32, 3233–3243. doi:10.1016/j.biomaterials.2011.01.057
Dadlani, S. (2021). Porcine acellular dermal matrix: an alternative to connective tissue graft—a narrative review. Int. J. Dent. 2021, 1–7. doi:10.1155/2021/1652032
Debels, H., Hamdi, M., Abberton, K., and Morrison, W. (2015). Dermal matrices and bioengineered skin substitutes: a critical review of current options. Plastic Reconstr. Surg. Glob. open 3, e284. doi:10.1097/GOX.0000000000000219
Dorweiler, B., Trinh, T., Dünschede, F., Vahl, C., Debus, E., Storck, M., et al. (2018). The marine Omega3 wound matrix for treatment of complicated wounds: a multicenter experience report. Gefasschirurgie 23, 46–55. doi:10.1007/s00772-018-0428-2
Duarte, M. M., Silva, I. V., Eisenhut, A. R., Bionda, N., Duarte, A. R. C., and Oliveira, A. L. (2022). Contributions of supercritical fluid technology for advancing decellularization and postprocessing of viable biological materials. Mater. Horizons 9, 864–891. doi:10.1039/D1MH01720A
Emami, A., Talaei-Khozani, T., Vojdani, Z., and Zarei Fard, N. (2021). Comparative assessment of the efficiency of various decellularization agents for bone tissue engineering. J. Biomed. Mater. Res. Part B Appl. Biomaterials 109, 19–32. doi:10.1002/jbm.b.34677
Escudero, G. E., Romañuk, C. B., Toledo, M. E., Olivera, M. E., Manzo, R. H., and Laino, C. H. (2015). Analgesia enhancement and prevention of tolerance to morphine: beneficial effects of combined therapy with omega-3 fatty acids. J. Pharm. Pharmacol. 67, 1251–1262. doi:10.1111/jphp.12416
Farrokhi, A., Pakyari, M., Nabai, L., Pourghadiri, A., Hartwell, R., Jalili, R., et al. (2018). Evaluation of detergent-free and detergent-based methods for decellularization of murine skin. Tissue Eng. Part A 24, 955–967. doi:10.1089/ten.tea.2017.0273
Gao, X., Jiang, G., Ruan, L., Sun, Y., Yunusov, K. E., Jing, Y., et al. (2023). Electrospun porcine acellular dermal matrix and polycaprolactone composite nanofibrous scaffolds for accelerating wound healing. Fibers Polym. 24, 589–601. doi:10.1007/s12221-023-00077-z
Giang, N. N., Trinh, X. T., Han, J., Chien, P. N., Lee, J., Noh, S. R., et al. (2022). Effective decellularization of human skin tissue for regenerative medicine by supercritical carbon dioxide technique. J. Tissue Eng. Regen. Med. 16, 1196–1207. doi:10.1002/term.3359
Gierek, M., Łabuś, W., Kitala, D., Lorek, A., Ochała-Gierek, G., Zagórska, K. M., et al. (2022). Human acellular dermal matrix in reconstructive surgery—a review. Biomedicines 10, 2870. doi:10.3390/biomedicines10112870
Gravina, P. R., Pettit, R. W., Davis, M. J., Winocour, S. J., and Selber, J. C. (2019). Evidence for the use of acellular dermal matrix in implant-based breast reconstruction. Seminars Plastic Surg. 33, 229–235. doi:10.1055/s-0039-1696986
Groth, D., Poplawska, I., Tynecka, M., Grubczak, K., Holl, J., Starosz, A., et al. (2021). Abdominoplasty skin-based dressing for deep wound treatment—evaluation of different methods of preparation on therapeutic potential. Pharmaceutics 13, 2118. doi:10.3390/pharmaceutics13122118
Gupta, S. K., Mishra, N. C., and Dhasmana, A. (2018). Decellularization methods for scaffold fabrication. Decellularized Scaffolds Organog. Methods Protoc. 1577, 1–10. doi:10.1007/7651_2017_34
Han, X., Liu, H., Chen, M., Gong, L., Pang, H., Deng, X., et al. (2016). Acellular dermal matrix from one-day-old mouse skin on adult scarless cutaneous wound repair by second harmonic generation microscopic imaging. Rsc Adv. 6, 71852–71862. doi:10.1039/C6RA11179C
Heath, D. E. (2019). A review of decellularized extracellular matrix biomaterials for regenerative engineering applications. Regen. Eng. Transl. Med. 5, 155–166. doi:10.1007/s40883-018-0080-0
Heck, E. L., Bergstresser, P. R., and Baxter, C. R. (1985). Composite skin graft: frozen dermal allografts support the engraftment and expansion of autologous epidermis. J. Trauma Acute Care Surg. 25, 106–112. doi:10.1097/00005373-198502000-00002
Hillebrandt, K. H., Everwien, H., Haep, N., Keshi, E., Pratschke, J., and Sauer, I. M. (2019). Strategies based on organ decellularization and recellularization. Transpl. Int. 32, 571–585. doi:10.1111/tri.13462
Huang, C.-C., Chen, Y.-J., and Liu, H.-W. (2021). Characterization of composite nano-bioscaffolds based on collagen and supercritical fluids-assisted decellularized fibrous extracellular matrix. Polymers 13, 4326. doi:10.3390/polym13244326
Huang, Z., Godkin, O., and Schulze-Tanzil, G. (2017). The challenge in using mesenchymal stromal cells for recellularization of decellularized cartilage. Stem Cell Rev. Rep. 13, 50–67. doi:10.1007/s12015-016-9699-8
Hussein, K. H., Park, K.-M., Kang, K.-S., and Woo, H.-M. (2016). Biocompatibility evaluation of tissue-engineered decellularized scaffolds for biomedical application. Mater. Sci. Eng. C 67, 766–778. doi:10.1016/j.msec.2016.05.068
Imai, Y. (2015). Role of omega-3 PUFA-derived mediators, the protectins, in influenza virus infection. Biochimica Biophysica Acta (BBA)-Molecular Cell Biol. Lipids 1851, 496–502. doi:10.1016/j.bbalip.2015.01.006
Jin, R., Cui, Y., Chen, H., Zhang, Z., Weng, T., Xia, S., et al. (2021). Three-dimensional bioprinting of a full-thickness functional skin model using acellular dermal matrix and gelatin methacrylamide bioink. Acta Biomater. 131, 248–261. doi:10.1016/j.actbio.2021.07.012
Joodaki, H., and Panzer, M. B. (2018). Skin mechanical properties and modeling: a review. Proc. Institution Mech. Eng. Part H J. Eng. Med. 232, 323–343. doi:10.1177/0954411918759801
Jorgensen, A. M., Chou, Z., Gillispie, G., Lee, S. J., Yoo, J. J., Soker, S., et al. (2020). Decellularized skin extracellular matrix (dsECM) improves the physical and biological properties of fibrinogen hydrogel for skin bioprinting applications. Nanomaterials 10, 1484. doi:10.3390/nano10081484
Kabirian, F., and Mozafari, M. (2020). Decellularized ECM-derived bioinks: prospects for the future. Methods 171, 108–118. doi:10.1016/j.ymeth.2019.04.019
Kasravi, M., Ahmadi, A., Babajani, A., Mazloomnejad, R., Hatamnejad, M. R., Shariatzadeh, S., et al. (2023). Immunogenicity of decellularized extracellular matrix scaffolds: a bottleneck in tissue engineering and regenerative medicine. Biomaterials Res. 27, 10–24. doi:10.1186/s40824-023-00348-z
Kim, B. S., Ahn, M., Cho, W.-W., Gao, G., Jang, J., and Cho, D.-W. (2021). Engineering of diseased human skin equivalent using 3D cell printing for representing pathophysiological hallmarks of type 2 diabetes in vitro. Biomaterials 272, 120776. doi:10.1016/j.biomaterials.2021.120776
Kim, B. S., Kwon, Y. W., Kong, J.-S., Park, G. T., Gao, G., Han, W., et al. (2018). 3D cell printing of in vitro stabilized skin model and in vivo pre-vascularized skin patch using tissue-specific extracellular matrix bioink: a step towards advanced skin tissue engineering. Biomaterials 168, 38–53. doi:10.1016/j.biomaterials.2018.03.040
Kim, Y. H., Hwang, K. T., Kim, K. H., Sung, I. H., and Kim, S. W. (2019). Application of acellular human dermis and skin grafts for lower extremity reconstruction. J. Wound Care 28, S12–S17. doi:10.12968/jowc.2019.28.Sup4.S12
Ko, G. D., Nowacki, N. B., Arseneau, L., Eitel, M., and Hum, A. (2010). Omega-3 fatty acids for neuropathic pain: case series. Clin. J. pain 26, 168–172. doi:10.1097/AJP.0b013e3181bb8533
Kumar, N., Kumar, V., Purohit, S., Gangwar, A. K., Shrivastava, S., Maiti, S. K., et al. (2021a). “Decellularization of skin tissue,” in Decellularization methods of tissue and whole organ in tissue engineering. Editor A Kajbafzadeh (Berlin, Germany: Springer), 165–191.
Kumar, N., Purohit, S., Kumar, V., Gangwar, A. K., Shrivastava, S., Saxena, S., et al. (2022a). “Tissue scaffolds derived from sheep skin,” in Tissue scaffolds. Editors N Kumar, V Kumar, S Shrivastava, AK Gangwar, and S Saxena (Berlin, Germany: Springer), 57–64.
Kumar, N., Purohit, S., Sharma, A. K., Mathew, D. D., Gangwar, A. K., Kumar, V., et al. (2022b). “Tissue scaffolds derived from rabbit skin and clinical applications,” in Tissue scaffolds (Berlin, Germany: Springer), 29–45.
Kumar, N. G., Berlin, N. L., Kim, H. M., Hamill, J. B., Kozlow, J. H., and Wilkins, E. G. (2021b). Development of an evidence-based approach to the use of acellular dermal matrix in immediate expander-implant-based breast reconstruction. J. Plastic, Reconstr. Aesthetic Surg. 74, 30–40. doi:10.1016/j.bjps.2020.10.005
Kumar, V., Kumar, N., Asodiya, F. A., Purohit, S., Sharma, A. K., Mathew, D. D., et al. (2022c). “Tissue scaffolds derived from goat skin and clinical applications,” in Tissue scaffolds. Editors N Kumar, V Kumar, S Shrivastava, AK Gangwar, and S Saxena (Berlin, Germany: Springer), 47–56.
Lantis, J. C., Snyder, R., Reyzelman, A. M., Van Gils, C. C., Sigal, F., Vayser, D., et al. (2021). Fetal bovine acellular dermal matrix for the closure of diabetic foot ulcers: a prospective randomised controlled trial. J. wound care 30, S18–S27. doi:10.12968/jowc.2021.30.Sup7.S18
Lattari, V., Jones, L. M., Varcelotti, J. R., Latenser, B. A., Sherman, H. F., and Barrette, R. R. (1997). The use of a permanent dermal allograft in full-thickness burns of the hand and foot: a report of three cases. J. burn care & rehabilitation 18, 147–155. doi:10.1097/00004630-199703000-00010
Lee, S. J., Lee, J. H., Park, J., Kim, W. D., and Park, S. A. (2020). Fabrication of 3D printing scaffold with porcine skin decellularized bio-ink for soft tissue engineering. Materials 13, 3522. doi:10.3390/ma13163522
Li, D., Sun, W. Q., Wang, T., Gao, Y., Wu, J., Xie, Z., et al. (2021). Evaluation of a novel tilapia-skin acellular dermis matrix rationally processed for enhanced wound healing. Mater. Sci. Eng. C 127, 112202. doi:10.1016/j.msec.2021.112202
Lightfoot, R. W., Thrash, C., Thompson, S., and Richmond, B. K. (2023). A comparison of component separation with porcine acellular dermal reinforcement to bovine acellular dermal matrix in the repair of significant midline ventral hernia defects. Am. Surgeon™ 89, 1003–1008. doi:10.1177/00031348211050844
Litman, T. (2019). Personalized medicine-concepts, technologies, and applications in inflammatory skin diseases. Apmis 127, 386–424. doi:10.1111/apm.12934
Liu, D. Z., Mathes, D. W., Neligan, P. C., Said, H. K., and Louie, O. (2014). Comparison of outcomes using AlloDerm versus FlexHD for implant-based breast reconstruction. Ann. Plastic Surg. 72, 503–507. doi:10.1097/SAP.0b013e318268a87c
Louri, N. A., Dey, N., Alhasan, R. N., Abdulla, S. H., Elsakka, M., Gulreez, R., et al. (2022). Abdominoplasty panniculus as a source for human acellular dermis: a preliminary report. Tissue Eng. Regen. Med. 19, 727–738. doi:10.1007/s13770-022-00439-3
Luthringer, M., Mukherjee, T., Arguello-Angarita, M., Granick, M. S., and Alvarez, O. M. (2020). Human-derived acellular dermal matrix grafts for treatment of diabetic foot ulcers: a systematic review and meta-analysis. Wounds 32, 57–65.
Lynch, M. P., Chung, M. T., and Rinker, B. D. (2015). A comparison of dermal autograft and acellular dermal matrix in tissue expander breast reconstruction: long-term aesthetic outcomes and capsular contracture. Ann. Plastic Surg. 74, S214–S217. doi:10.1097/SAP.0000000000000375
Ma, T., Xu, L., Chen, Y., Zhang, J., Han, X., and Jiang, L. (2022). Full-thickness lower eyelid defect reconstruction using a pedicle rotation temporal flap and Acellular Human Dermis Graft (Alloderm). J. Plastic, Reconstr. Aesthetic Surg. 75, 3414–3419. doi:10.1016/j.bjps.2022.04.082
Mansour, R. N., Karimizade, A., Enderami, S. E., Abasi, M., Talebpour Amiri, F., Jafarirad, A., et al. (2023). The effect of source animal age, decellularization protocol, and sterilization method on bovine acellular dermal matrix as a scaffold for wound healing and skin regeneration. Artif. Organs 47, 302–316. doi:10.1111/aor.14415
Martin, J. B., Moore, R., Paydar, K. Z., and Wirth, G. A. (2014). Use of fenestrations in acellular dermal allograft in two-stage tissue expander/implant breast reconstruction. Plastic Reconstr. Surg. 134, 901–904. doi:10.1097/PRS.0000000000000598
Mcinnes, A. D., Moser, M. A., and Chen, X. (2022). Preparation and use of decellularized extracellular matrix for tissue engineering. J. Funct. biomaterials 13, 240. doi:10.3390/jfb13040240
Melandri, D., Marongiu, F., Carboni, A., Rubino, C., Razzano, S., Purpura, V., et al. (2020). A new human-derived acellular dermal matrix for 1-stage coverage of exposed tendons in the foot. Int. J. Low. Extrem. Wounds 19, 78–85. doi:10.1177/1534734619884422
Melman, L., Jenkins, E., Hamilton, N., Bender, L., Brodt, M., Deeken, C., et al. (2011). Early biocompatibility of crosslinked and non-crosslinked biologic meshes in a porcine model of ventral hernia repair. Hernia 15, 157–164. doi:10.1007/s10029-010-0770-0
Mendibil, U., Ruiz-Hernandez, R., Retegi-Carrion, S., Garcia-Urquia, N., Olalde-Graells, B., and Abarrategi, A. (2020). Tissue-specific decellularization methods: rationale and strategies to achieve regenerative compounds. Int. J. Mol. Sci. 21, 5447. doi:10.3390/ijms21155447
Michelotti, B. F., Brooke, S., Mesa, J., Wilson, M. Z., Moyer, K., Mackay, D. R., et al. (2013). Analysis of clinically significant seroma formation in breast reconstruction using acellular dermal grafts. Ann. plastic Surg. 71, 274–277. doi:10.1097/SAP.0b013e3182923dc9
Milan, P. B., Pazouki, A., Joghataei, M. T., Mozafari, M., Amini, N., Kargozar, S., et al. (2020). Decellularization and preservation of human skin: a platform for tissue engineering and reconstructive surgery. Methods 171, 62–67. doi:10.1016/j.ymeth.2019.07.005
Mil-Homens, D., Bernardes, N., and Fialho, A. M. (2012). The antibacterial properties of docosahexaenoic omega-3 fatty acid against the cystic fibrosis multiresistant pathogen Burkholderia cenocepacia. FEMS Microbiol. Lett. 328, 61–69. doi:10.1111/j.1574-6968.2011.02476.x
Mirastschijski, U., Kerzel, C., Schnabel, R., Strauss, S., and Breuing, K.-H. (2013). Complete horizontal skin cell resurfacing and delayed vertical cell infiltration into porcine reconstructive tissue matrix compared to bovine collagen matrix and human dermis. Plastic Reconstr. Surg. 132, 861–869. doi:10.1097/PRS.0b013e31829fe461
Nafisi, N., Akbari, M. E., Mahjoub, F., Mohseni, M. J., Sabetkish, S., Khorramirouz, R., et al. (2017). Application of human acellular breast dermal matrix (ABDM) in implant-based breast reconstruction: an experimental study. Aesthetic Plast. Surg. 41, 1435–1444. doi:10.1007/s00266-017-0931-y
Nakamura, N., Kimura, T., and Kishida, A. (2017). Overview of the development, applications, and future perspectives of decellularized tissues and organs. ACS Biomaterials Sci. Eng. 3, 1236–1244. doi:10.1021/acsbiomaterials.6b00506
Olga, M., Doina, R., Adrian, C., and Viorel, N. (2021). Comparative analysis of the skin decellularization methods. Moldovan Med. J. 64, 79–86. doi:10.52418/moldovan-med-j.64-2.21.14
Oliveira, A. L., Ribeiro, V. P., Rosadas, M., Silva, I. V., Sousa, A., Reis, A. H., et al. (2023). Skin substitutes, methods and uses thereof. PT patent application 118787.GB0907974D0.
Pabst, A. M., Lehmann, K.-M., Walter, C., Krüger, M., Stratul, S.-I., and Kasaj, A. (2016). Influence of porcine-derived collagen matrix on endothelial progenitor cells: an in vitro study. Odontology 104, 19–26. doi:10.1007/s10266-014-0186-x
Papi, P., and Pompa, G. (2018). The use of a novel porcine derived acellular dermal matrix (mucoderm) in peri-implant soft tissue augmentation: preliminary results of a prospective pilot cohort study. BioMed Res. Int. 2018, 1–9. doi:10.1155/2018/6406051
Park, S., Kim, Y., and Jang, S. (2018). The application of an acellular dermal allograft (AlloDerm) for patients with insufficient conjunctiva during evisceration and implantation surgery. Eye 32, 136–141. doi:10.1038/eye.2017.161
Patel, S., Ziai, K., Lighthall, J. G., and Walen, S. G. (2022). Biologics and acellular dermal matrices in head and neck reconstruction: a comprehensive review. Am. J. Otolaryngology 43, 103233. doi:10.1016/j.amjoto.2021.103233
Petrie, K., Cox, C. T., Becker, B. C., and Mackay, B. J. (2022). Clinical applications of acellular dermal matrices: a review. Scars, Burns Heal. 8, 205951312110383. doi:10.1177/20595131211038313
Poornejad, N., Momtahan, N., Salehi, A. S., Scott, D. R., Fronk, C. A., Roeder, B. L., et al. (2016). Efficient decellularization of whole porcine kidneys improves reseeded cell behavior. Biomed. Mater. 11, 025003. doi:10.1088/1748-6041/11/2/025003
Porzionato, A., Stocco, E., Barbon, S., Grandi, F., Macchi, V., and De Caro, R. (2018). Tissue-engineered grafts from human decellularized extracellular matrices: a systematic review and future perspectives. Int. J. Mol. Sci. 19, 4117. doi:10.3390/ijms19124117
Rabbani, M., Zakian, N., and Alimoradi, N. (2021). Contribution of physical methods in decellularization of animal tissues. J. Med. signals sensors 11, 1. doi:10.4103/jmss.JMSS_2_20
Rajabimashhadi, Z., Gallo, N., Salvatore, L., and Lionetto, F. (2023). Collagen derived from fish industry waste: progresses and challenges. Polymers 15, 544. doi:10.3390/polym15030544
Rakers, S., Gebert, M., Uppalapati, S., Meyer, W., Maderson, P., Sell, A. F., et al. (2010). ‘Fish matters’: the relevance of fish skin biology to investigative dermatology. Exp. Dermatol. 19, 313–324. doi:10.1111/j.1600-0625.2009.01059.x
Ramalingam, R., and Häkkinen, L. (2023). Novel decellularization method to generate cell-free extracellular matrices from three-dimensional human gingival fibroblast cultures. Research Square. doi:10.21203/rs.3.pex-2169/v1
Rana, D., Zreiqat, H., Benkirane-Jessel, N., Ramakrishna, S., and Ramalingam, M. (2017). Development of decellularized scaffolds for stem cell-driven tissue engineering. J. tissue Eng. Regen. Med. 11, 942–965. doi:10.1002/term.2061
Ranganathan, K., Santosa, K. B., Lyons, D. A., Mand, S., Xin, M., Kidwell, K., et al. (2015). Use of acellular dermal matrix in postmastectomy breast reconstruction: are all acellular dermal matrices created equal? Plastic Reconstr. Surg. 136, 647–653. doi:10.1097/PRS.0000000000001569
Ricci, J. A., Treiser, M. D., Tao, R., Jiang, W., Guldbrandsen, G., Halvorson, E., et al. (2016). Predictors of complications and comparison of outcomes using SurgiMend fetal bovine and AlloDerm human cadaveric acellular dermal matrices in implant-based breast reconstruction. Plastic Reconstr. Surg. 138, 583e–591e. doi:10.1097/PRS.0000000000002535
Rippa, A. L., Kalabusheva, E. P., and Vorotelyak, E. A. (2019). Regeneration of dermis: scarring and cells involved. Cells 8, 607. doi:10.3390/cells8060607
Saricilar, E. C., and Huang, S. (2021). Comparison of porcine and human acellular dermal matrix outcomes in wound healing: a deep dive into the evidence. Archives plastic Surg. 48, 433–439. doi:10.5999/aps.2020.02306
Schmidt, F. F., Nowakowski, S., and Kluger, P. J. (2020). Improvement of a three-layered in vitro skin model for topical application of irritating substances. Front. Bioeng. Biotechnol. 8, 388. doi:10.3389/fbioe.2020.00388
Schuurman, H.-J. (2015). Regulatory aspects of clinical xenotransplantation. Int. J. Surg. 23, 312–321. doi:10.1016/j.ijsu.2015.09.051
Sedmak, D., and Orosz, C. (1991). The role of vascular endothelial cells in transplantation. Archives pathology laboratory Med. 115, 260–265.
Serhan, C. N. (2014). Pro-resolving lipid mediators are leads for resolution physiology. Nature 510, 92–101. doi:10.1038/nature13479
Sobti, N., and Liao, E. C. (2016). Surgeon-controlled study and meta-analysis comparing FlexHD and AlloDerm in immediate breast reconstruction outcomes. Plastic Reconstr. Surg. 138, 959–967. doi:10.1097/PRS.0000000000002616
Solarte David, V. A., Güiza-Argüello, V. R., Arango-Rodríguez, M. L., Sossa, C. L., and Becerra-Bayona, S. M. (2022). Decellularized tissues for wound healing: towards closing the gap between scaffold design and effective extracellular matrix remodeling. Front. Bioeng. Biotechnol. 10, 821852. doi:10.3389/fbioe.2022.821852
Sorkin, M., Qi, J., Kim, H. M., Hamill, J. B., Kozlow, J. H., Pusic, A. L., et al. (2017). Acellular dermal matrix in immediate expander/implant breast reconstruction: a multicenter assessment of risks and benefits. Plastic Reconstr. Surg. 140, 1091–1100. doi:10.1097/PRS.0000000000003842
Sotnichenko, A., Gilevich, I., Melkonyan, K., Yutskevich, Y., Rusinova, T., Karakulev, A., et al. (2021). Comparative morphological characteristics of the results of implantation of decellularized and recellularized porcine skin scaffolds. Bull. Exp. Biol. Med. 170, 378–383. doi:10.1007/s10517-021-05071-0
Taylor, D. A., Sampaio, L. C., Ferdous, Z., Gobin, A. S., and Taite, L. J. (2018). Decellularized matrices in regenerative medicine. Acta biomater. 74, 74–89. doi:10.1016/j.actbio.2018.04.044
Tognetti, L., Pianigiani, E., Ierardi, F., Lorenzini, G., Casella, D., Liso, F. G., et al. (2021). The use of human acellular dermal matrices in advanced wound healing and surgical procedures: state of the art. Dermatol. Ther. 34, e14987. doi:10.1111/dth.14987
Van Orden, K., Santos, J., Stanfield, B., Frost, L. S., Ruditsky, A., Foster, A., et al. (2022). Bovine versus porcine acellular dermal matrix for abdominal wall herniorrhaphy or bridging. Eur. J. Trauma Emerg. Surg. 48, 1993–2001. doi:10.1007/s00068-021-01641-z
Ventura, R. D., Padalhin, A. R., Park, C. M., and Lee, B. T. (2019). Enhanced decellularization technique of porcine dermal ECM for tissue engineering applications. Mater. Sci. Eng. C 104, 109841. doi:10.1016/j.msec.2019.109841
Vijayavenkataraman, S., Yan, W.-C., Lu, W. F., Wang, C.-H., and Fuh, J. Y. H. (2018). 3D bioprinting of tissues and organs for regenerative medicine. Adv. drug Deliv. Rev. 132, 296–332. doi:10.1016/j.addr.2018.07.004
Wainwright, D. (1995). Use of an acellular allograft dermal matrix (AlloDerm) in the management of full-thickness burns. Burns 21, 243–248. doi:10.1016/0305-4179(95)93866-I
Wang, C.-H., Hsieh, D.-J., Periasamy, S., Chuang, C.-T., Tseng, F.-W., Kuo, J.-C., et al. (2020a). Regenerative porcine dermal collagen matrix developed by supercritical carbon dioxide extraction technology: role in accelerated wound healing. Materialia 9, 100576. doi:10.1016/j.mtla.2019.100576
Wang, L., Gong, J., Dan, Y., Huang, Y., Dan, N., and Dan, W. (2020b). Preparation and characterization of antibacterial porcine acellular dermal matrices with high performance. ACS omega 5, 20238–20249. doi:10.1021/acsomega.0c01940
White, L. J., Taylor, A. J., Faulk, D. M., Keane, T. J., Saldin, L. T., Reing, J. E., et al. (2017). The impact of detergents on the tissue decellularization process: a ToF-SIMS study. Acta biomater. 50, 207–219. doi:10.1016/j.actbio.2016.12.033
Wolfinbarger, L., Lange, P., Linhurst, A., Moore, E., and Nolf, B. (2004). Process for decellularizing soft-tissue engineered medical implants, and decellularized soft-tissue medical implants produced. US20090041729A1.
Won, J.-Y., Lee, M.-H., Kim, M.-J., Min, K.-H., Ahn, G., Han, J.-S., et al. (2019). A potential dermal substitute using decellularized dermis extracellular matrix derived bio-ink. Artif. Cells, Nanomedicine, Biotechnol. 47, 644–649. doi:10.1080/21691401.2019.1575842
Yang, J., Dang, H., and Xu, Y. (2022). Recent advancement of decellularization extracellular matrix for tissue engineering and biomedical application. Artif. Organs 46, 549–567. doi:10.1111/aor.14126
Yao, Q., Zheng, Y.-W., Lan, Q.-H., Kou, L., Xu, H.-L., and Zhao, Y.-Z. (2019). Recent development and biomedical applications of decellularized extracellular matrix biomaterials. Mater. Sci. Eng. C 104, 109942. doi:10.1016/j.msec.2019.109942
Zhang, W., Du, A., Liu, S., Lv, M., and Chen, S. (2021). Research progress in decellularized extracellular matrix-derived hydrogels. Regen. Ther. 18, 88–96. doi:10.1016/j.reth.2021.04.002
Zhang, X., Chen, X., Hong, H., Hu, R., Liu, J., and Liu, C. (2022). Decellularized extracellular matrix scaffolds: recent trends and emerging strategies in tissue engineering. Bioact. Mater. 10, 15–31. doi:10.1016/j.bioactmat.2021.09.014
Zhang, X., Yang, J., Li, Y., Liu, S., Long, K., Zhao, Q., et al. (2011). Functional neovascularization in tissue engineering with porcine acellular dermal matrix and human umbilical vein endothelial cells. Tissue Eng. Part C. Methods 17, 423–433. doi:10.1089/ten.tec.2010.0466
Zhang, Y., Zhang, C., Li, Y., Zhou, L., Dan, N., Min, J., et al. (2023). Evolution of biomimetic ECM scaffolds from decellularized tissue matrix for tissue engineering: a comprehensive review. Int. J. Biol. Macromol. 246, 125672. doi:10.1016/j.ijbiomac.2023.125672
Keywords: decellularization, dermal matrix, biomaterials, biological scaffolds, tissue engineering, regenerative medicine
Citation: Rosadas M, Silva IV, Costa JB, Ribeiro VP and Oliveira AL (2024) Decellularized dermal matrices: unleashing the potential in tissue engineering and regenerative medicine. Front. Mater. 10:1285948. doi: 10.3389/fmats.2023.1285948
Received: 30 August 2023; Accepted: 20 December 2023;
Published: 12 January 2024.
Edited by:
Dimitrios Kontziampasis, University of Dundee, United KingdomReviewed by:
Tahir Farooq, Government College University, Faisalabad, PakistanCopyright © 2024 Rosadas, Silva, Costa, Ribeiro and Oliveira. This is an open-access article distributed under the terms of the Creative Commons Attribution License (CC BY). The use, distribution or reproduction in other forums is permitted, provided the original author(s) and the copyright owner(s) are credited and that the original publication in this journal is cited, in accordance with accepted academic practice. No use, distribution or reproduction is permitted which does not comply with these terms.
*Correspondence: Viviana P. Ribeiro, dnByaWJlaXJvQHVjcC5wdA==; Ana L. Oliveira, YWxvbGl2ZWlyYUB1Y3AucHQ=
Disclaimer: All claims expressed in this article are solely those of the authors and do not necessarily represent those of their affiliated organizations, or those of the publisher, the editors and the reviewers. Any product that may be evaluated in this article or claim that may be made by its manufacturer is not guaranteed or endorsed by the publisher.
Research integrity at Frontiers
Learn more about the work of our research integrity team to safeguard the quality of each article we publish.