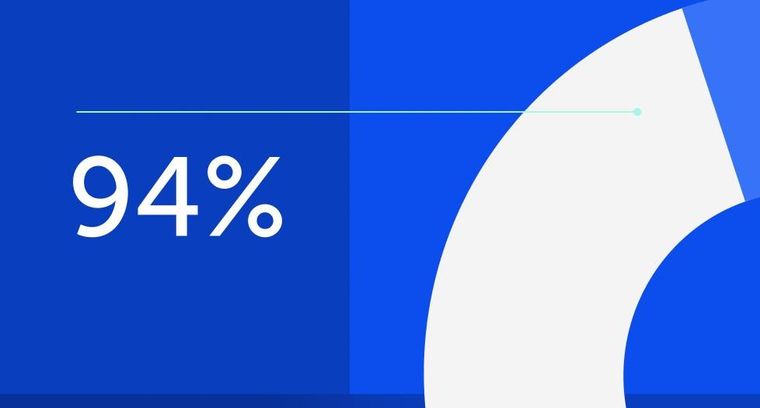
94% of researchers rate our articles as excellent or good
Learn more about the work of our research integrity team to safeguard the quality of each article we publish.
Find out more
ORIGINAL RESEARCH article
Front. Mater., 27 July 2023
Sec. Carbon-Based Materials
Volume 10 - 2023 | https://doi.org/10.3389/fmats.2023.1242507
This article is part of the Research TopicRenewable Biosourced Carbon Materials Derived From Biomass and Their Biocomposites Fabrication for Innovative ApplicationsView all 4 articles
This study focuses on the development of environmentally friendly and chemically recyclable thermosets using or a renewable based monomer, the triglycidyl ether of phloroglucinol (TGPh), or a commercial non-toxic tris(4-hydroxyphenyl) methane triglycidyl ether (THPMTGE) monomer. The recyclable polyester thermosets were prepared by crosslinking the two monomers with hexahydro-4-methylphthalic anhydride (HMPA) or methyl nadic anhydride The TGPh-based formulations exhibited lower reaction temperatures and narrower reaction intervals. Additionally, these systems showed higher tan δ values (189°C–199°C), higher crosslinking densities (7.6–7.8 mmol cm−3) and compact networks, crucial for high-performance industries. Tensile tests demonstrated the remarkable mechanical properties of the thermosets, including high Young modulus (1.3–1.4 GPa), tensile stress (55–69 MPa), and an elongation at break around 3%–8%. Moreover, the thermosets exhibited complete dissolution at a temperature of 170°C, with depolymerization times of approximately 2.5 h for TGPh-based resins and 4.5 h for THPMTGE-based formulations. In conclusion, this study shows that sustainable and eco-friendly thermosets with excellent physico-chemical and thermo-mechanical properties, low hydrophilicity, and rapid dissolution capacity can be developed. These thermosets offer a viable alternative to non-recyclable and toxic resins in high-end industrial applications.
Plastic materials represent one of the most versatile and challenging human innovation, covering multitude of characteristics and therefore facilitating myriad of applications (Geyer et al., 2017). In 2020, the global plastic production was estimated at around 367 million tons (Mt), Europe generating about 55 Mt of the total worldwide production. Although plastics were initially viewed as harmless products, nowadays their massive production and disposal in the environment made them one of the main polluting wastes negatively affecting the ecosystem (Okunola A et al., 2019).
Epoxy-based materials are representing 75% of industrial and domestic needs in thermosetting resins, due to a wide range of valuable properties such as strong chemical and corrosion resistance, low shrinkage on curing, high mechanical properties, high temperature stability, outstanding adhesion to a variety of substrates, etc., (Sukanto et al., 2021). Usually, epoxy resins are used in various application areas such as structural adhesives, surface coatings, electrical engineering, electronic encapsulation, construction, and high performance applications like construction, automotive, aerospace and space or naval industries (Capricho et al., 2020). Although epoxy materials are extremely needed in all these industries, the chemical compounds used in their production are not the most environmentally friendly. For example, about 90% of epoxy materials are based on diglycidyl ether of bisphenol A (DGEBA), a petro-based molecule derived from bisphenol A (BPA). BPA has been classified as carcinogen, mutagen and reprotoxic (CMR), being prohibited in many countries (Moreman et al., 2017). Due to the unpredictable fluctuations of the oil price and production, the non-renewable nature of fossil derivatives, as well as the growing concerns regarding environmental protection, academic and industrial research have turned their attention to the development of materials based on natural and renewable compounds that are environmentally-safe (Wang et al., 2020; Derradji et al., 2021; Zhang et al., 2022; Briou et al., 2023).
The main objective of this study is the development of epoxy resins starting from natural and renewable resources, for high-tech industries as targeted fields of application. These biobased resins will be compared with homologous resins in which a commercial non-toxic petro-based compound is used as epoxy monomer. The biobased resins were designed starting from phloroglucinol (1,3,5-trihydroxybenzene) which can be naturally extracted from marine brown algae (Ecklonia Cava), from bark of fruit, or can be chemically synthesized by benzene (Chowdhury et al., 2011). The phloroglucinol is mostly used in medical field, but it can be a sustainable alternative to fossil compounds in applications such as thermosetting materials (Genua et al., 2020; Guzmán et al., 2020; Santiago et al., 2020). So a phloroglucinol tris-epoxy based monomer (TGPh) was laboratory synthesized and compared with tris(4-hydroxyphenyl)methane triglycidyl ether (THPMTGE), a tris-aromatic tris-epoxide monomer, which is a commercial and non-toxic product, used in cosmetics, paints, coatings, inks for printing or writing, etc.
To develop the resins, we considered the industrial manufacturing requirements of an easy and cost-competitive production protocol. The two epoxy monomers were crosslinked with hexahydro-4-methylphthalic anhydride (HMPA) or methyl nadic anhydride (MNA). Physico-chemical and thermo-mechanical properties of the developed thermosets were investigated by differential scanning calorimetry (DSC), thermogravimetric analysis (TGA), dynamic mechanical analysis (DMA), Shore hardness tests, tensile tests, water absorption and gel content. The recyclability, but also the biobased carbon content (BCC) and biobased organic carbon (BOC) of the designed thermosets were also investigated to accurately frame the potential application of these resins for high tech industries.
The triglycidyl ether of phloroglucinol (TGPh, DP = 0.229, Mw = 348.92 g/mol) was laboratory synthetized. The tris(4-hydroxyphenyl) methane triglycidyl ether (THPMTGE), the hexahydro-4-methylphthalic anhydride (HMPA; 96%), the methyl nadic anhydride (MNA; ≥95%) and the 2-ethyl-4-methylimidazole (2E4M) were purchased from Sigma Aldrich (France) and used as received.
Thermosets were obtained by mixing the epoxy monomer with the hardener, in the presence of initiator. The formulations were crosslinked by applying a thermal treatment established by DSC studies: 3 h at 120°C and 1 h at 160°C, followed by a post-curing (1 h at 180°C) program.
The thermal behaviors were conducted on a DSC 3 Mettler Toledo apparatus. The fresh mixtures of uncured formulations were analyzed in dynamic conditions, from 25°C to 250°C at 10°C min−1. To determine the glass transition (Tg) of the thermosets was employed a cycle of two heating/cooling between 0 and 280°C at a rate of 10°C min−1. The Tg values were recorded as the inflection point of the DSC curves in the second heating scan.
The density of rectangular samples (50 × 8 × 4 mm3) was analyzed by calculating the ratio of mass to volume. To avoid potential errors, an average of five samples was considered as the final value for each system.
Thermo-mechanical behavior of the samples was investigated using a Mettler Toledo DMA 1 apparatus equipped with a three-point bending clamp. Mechanical properties were determined under heating from −50°C to 280°C at a heating rate of 3°C min−1 with a 1.0 Hz oscillating stress and 20 µm amplitude.
The materials’ stiffness was studied using a Zwick Roell 3116 hardness tester equipped with a Shore D hardness device, using a loading force of 50 N. The stiffness values were measured in accordance with ISO 7619-1, ASTM D2240 and ISO 868 standards. Each system was evaluated five times, the hardness value being determined as their average.
Mechanical properties of the developed thermosets were investigated based on ASTM D638-08 standard using an Instron 34SC-5 device equipped with a 5 kN load cell. Measurements were performed on dog-bone V-type specimens using a crosshead speed of 5 mm min−1. Ten measurements for each system were realized and averaged for better accuracy of the results.
Thermal stability of the thermosets was conducted under oxidative or inert atmosphere on a Mettler Toledo TGA 2 instrument. Samples of 10–13 mg were placed into 70 µL alumina pans and analyzed by heating from 25°C to 1,000°C, at a heating rate of 10°C min−1. The degradation temperature was considered the temperature at which the sample lost 5% of their mass (T5%).
The water absorption behavior was investigated based on the ASTM D570 standard test. The conditioned specimens (50°C, 24 h) (W0) were immersed in distilled water at room temperature. Every 24 h, the samples were extracted from water, wiped with filter paper, and weighed again (Wd). The percentage of water absorbed by the thermosets was calculated using the equation (ASTM D570-982018, 2018):
The gel content was determined according to ASTM D2765-16. The GC percentage was calculated (ASTM-D2765-16, 2016):
where W0 - initial mass, and Wf - mass of the dried samples after toluene immersion.
The developed thermosets were chemically recycled by immersion of samples in a 0.30 mol/L solution of 1,5,7-triazabicyclo [4.4.0 ]dec-5-ene (TBD) in ethylene glycol (EG). The polymer content was ∼5 wt% of the total mass of the solution. These so prepared immersions were maintained in oven at 170°C and normal pressure until the thermosets were completely dissolved.
The tensile fracture morphology of the thermoset resins was investigated by SEM. The analyzed samples were first coated with platinum and then studied in a Tescan Vega 3 XMU SEM device using an acceleration voltage of 5 kV.
The evolution of the heat flows during the dynamic heating of the four crosslinking formulations are displayed in Figure 1, while the reaction enthalpy (ΔH), the temperature interval of the reaction (Tonset-Tend), but also the maximum reaction peak temperature (Tpeak) are given in Table 1.
FIGURE 1. Dynamic DSC thermograms of the epoxy-anhydride curing systems during heating at 10°C min−1.
From Figure 1 it can be observed a similar curing profile for both epoxy monomers with the selected anhydrides. The crosslinking reaction of the epoxy/anhydride systems appears as quite narrow (excepting THPMTGE/MNA system) and well-defined exothermic peak. The TGPh-based formulations display a lower onset temperature of reactions at around 58°C, compared with the homologous formulations with THPMTGE which are ranged between 61–65°C. At the same time, the biobased monomer formulations have a smaller interval of temperature curing than the commercial one, the exothermic event returning at a quasi-linear response at about 201–212°C vs. 233–236°C for the THPMTGE-based systems. Another parameter giving information on the compounds reactivity is the Tpeak value of the reaction exothermic peak, lower the Tpeak value, higher is the formulation reactivity (Liu et al., 2009; Ma et al., 2014). From Table 1 we can notice that the TGPh monoaromatic epoxy monomer generates systems with higher reactivity than the formulations developed with the tris-aromatic epoxide. Also, depending on the anhydride used as hardener, the systems crosslinked with HMPA are more reactive than those with MNA. The reaction enthalpy (ΔH) of the studied systems was determined by integrating the areas under the exothermic event, the obtained values being reported in Table 1. We can notice again similar values of enthalpy for both epoxies cured with the same anhydride.
Based on these thermodynamic results, the TGPh epoxy monomer has a slightly higher reactivity compared with THPMTGE-based systems, while the formulations with HMPA exhibit also higher reactivities than those with MNA.
The thermomechanical properties of the designed thermosets investigated by DMA are displayed in Figure 2, and the related parameters are given in Table 2. For both TGPh- and THPMTGE-based thermosets, the storage modulus (E’) curves show the classical pattern for thermosets behavior during heating, with the three regions. Depending on the monomer nature, the value of E′ at room temperature is slightly higher for the systems based on the tris-aromatic epoxy (3–3.5 GPa) compared to those of mono-aromatic TGPh (2.9–3.1 GPa). Then, the systems crosslinked with MNA are stiffer at 25°C than the ones with HMPA anhydride. The storage modulus in the rubbery region is directly proportional with the crosslink density (ν) which was calculated according to rubber-elasticity theory: (Flory, 1953):
where E’ - rubbery modulus, R - gas constant, and T - absolute temperature (at ∼ tan δ + 90°C). The average mass of segments between crosslinks (Mc) was calculates according with Tobolsky equation (Tobolsky, 1960)
where ρ is the calculated density.
FIGURE 2. Storage moduli (E′) and damping factors (tan δ) in function of temperature curves obtained by DMA analysis.
We can observe that the mono-aromatic TGPh epoxide leads to the development of a more compact polymeric network, with a lower mobility of the chains, compared with THPMTGE. The ν of TGPh-thermosets is ranged between 7.6 and 8 mmol cm−3, while those with THPMTGE vary between 4.7 and 6.4 mmol cm−3. This behavior can be seen also from the Mc values that are lower for TGPh-thermosets, ∼144–152 g mol−1, than the commercial one (220–248 g mol−1). Then, among the two anhydrides the highest stiffness was obtained by the system copolymerized with MNA.
The α relaxations (glass transitions) of the designed thermosets were recorded by DMA at the maximum temperature of the damping factor (tan δ), and by DSC (Tg-DSC) as the inflection points in the DSC thermograms at the second heating scans (Table 2). Both epoxy monomers led to the development of thermoset resins with very high glass transitions, superior to 180°C. TGPh-based thermosets have higher tan δ values, with lower amplitude of the peaks compared to those developed with THPMTGE (Figure 2). The presence of a mono-aromatic ring in the composition of TGPh led to the development of more confined networks with shorter segment chains between crosslinks, generating stiff materials with higher mechanical properties compared to those of tris-aromatic based epoxy thermosets. Also, the anhydride chemical structure is influencing the final properties of the designed thermosets. The less stiff thermoset is the THPMTGE-HMPA one, with a tan δ ∼ 179°C, whereas the stiffer is the TGPh-MNA thermoset: tan δ ∼ 199°C.
The Young modulus, tensile stress, and elongation at break of the designed thermoset materials were measured in tensile mode at room temperature and the obtained values are tabulated in Table 3. The stress-strain curves are displayed in Figure 3A.
FIGURE 3. (A) Representative stress-strain curves for the epoxy/anhydride thermosets, and (B) the Young’s modulus and brittleness of the designed materials.
The tensile properties of the TGPh-based thermosets are different to those of materials obtained with THPMTGE monomer. As can be seen from the stress-strain curves (Figure 3A), the TGPh-based materials present a more ductile character (higher strain and area below the curve) compared to the THPMTGE-based system with the same anhydride, which tend to be more rigid and brittle. The tensile stress of the TGPh-based thermosets ranges between 55 and 70 MPa with an elongation at break of about 6–8%, while the tris-aromatic-based materials present lower values, the stress being ∼30–55 MPa, and respectively 3–6% elongation at break. The hardener nature also influences the tensile properties of the systems; the MNA-based resins revealing both higher stress and strain values. These low values of the elongation at break can be attributed to the high crosslinking density of the systems (Table 2) which limits the mobility in the polymeric network.
The Young modulus values for the materials with mono-aromatic TGPh-based monomer are slightly lower (∼1.3 GPa) than those based on the tris-aromatic THPMTGE that have values of about 1.4 GPa. Based on these results, we can appreciate that all the developed thermosets have a stiff character similar with the commercial resins used in high-performance areas such as Master Bond EP21TDCHT-LO: σ = 24.1 MPa & Young modulus = 1.21 GPa; Henkel Loctite® ECCOBOND EO7021: σ = 48 MPa & Young modulus = 1.45 GPa; Milliken® Veloxx LVP: σ = 37.9 MPa & Young modulus = 1.52 GPa, etc.
The energy at break of the thermosets were determined, the obtained values being given in Table 3. This parameter represents the energy absorbed by the systems at the time of failure, or more precisely, the necessary energy that must be invested in a material to be able to break. In this study, the obtained energy at break values confirms the mechanical behavior of the materials presented previously, so that materials with TGPh are more ductile, needing more energy to be broken, while materials with tris-aromatic epoxy require lower energies, describing a slightly more brittle character of the designed materials. This fragile character or not of the materials was also characterized and determined based on the studies reported by Brostow et al. (2006); Brostow. (2011); Brostow and Hagg Lobland (2010) The thermoset brittleness (B) was calculated based on the equation:
where εb is elongation at break (tensile test) and E′ is the storage modulus at room temperature and 1.0 Hz (DMA). It is known that the lower the B value, the less brittle and more resistant is the material. The lower values of B for the systems based on mono-aromatic monomer (0.044–0.059%·Pa/1010) depict a greater stability at break for these networks, compared with the networks built with the tris-aromatic THPMTGE monomers (0.052–0.104%·Pa/1010). Physical appearance and the surface morphology of the fractured samples after the tensile tests are depicted in Figure 4. These SEM images of fractures shows a sharp cutting, an homogeneous and smooth surface, which is correlated to a perfect and straight break during the tensile tests, the samples not being fragmented or torn.
FIGURE 4. (A) Physical appearance, and (B) SEM micrographs of the tensile rupture surfaces of the designed thermosets.
For a more in-depth characterization of the mechanical properties of the materials as well as for fitting them as accurately as possible into a certain field of applicability, important parameters for the industry such as specific modulus, specific strength and specific length were calculated and reported in Table 3. Specific modulus and specific strength are distinct characteristics that characterize the maximum strength of a material that can hold it at a minimum weight. Regarding the specific modulus, the biobased materials show slightly lower values (1.07–1.14 106 m2/s2) compared to those of the THPMTGE-based materials, being in opposition to the values of the specific strength which are higher for the TGPh-based thermosets (47–57 kN·m/kg) compared to THPMTGE ones (26–49 kN·m/kg). Specific length of the materials represents the maximum self-supporting length of a material before it breaks. From Table 3 it can be stated that the TGPh-thermosets are more resistant (4.83–5.76 km) than the ones with THPMTGE (2.61–4.96 km).
The superior mechanical properties of the systems based on TGPh and THPMTGE support and confirm the capability of these thermoset resins for replacing the BPA-based systems used in high technology sectors such as construction, automotive and transport, naval, or aerospace and space.
The thermal behavior of the thermosets was studied in both oxidative and inert atmospheres. Graphical representation of mass loss as function of temperature is displayed in Figure 5, and the data summarized in Table 4.
The decomposition temperature (T5%) of the tested systems was settled as the temperature at which 5 wt% of their mass is degraded. The TGPh-thermosets exhibit a high T5%, ranged between 290–310°C, very close to the THPMTGE materials (300–320°C) or other commercial ones used in high-end applications like Master Bond EP41S-6: T5% = 260°C, Aremco Aremco-Bond™ 2,330: T5% = 300°C or EPO-TEK® EK 2000: T5% = 300°C. Based on the anhydride nature, systems crosslinked with HMPA have higher thermal stability (with ∼20°C) compared to those with MNA. The heat tolerance limit temperature of the polymeric materials, called the statistical heat resistance index (Ts), was determined using the equation (Ma et al., 2014; Ma et al. (2017):
where T30% represents the temperature at which the material loses 30% of its mass. As can be seen (Table 4), the Ts values of the TGPh-thermosets are slightly lower than those obtained for the THPMTGE, but remain similar to the commercial ones, being classified as heat-resistant materials (Ts = 100–200°C). (Heat resistance index and classification of plastic profile products, n. d.) The thermal behavior of thermosets in the oxidative atmosphere shows two main stages of decomposition mechanism (Figure 5A). The first degradation step, ranged between 330–470°C with a maximum at around 380–420°C, represents the main degradation stage of the materials in which the mass loss is ∼62–73% and can be attributed to the thermolysis of the polymeric network. In the second decomposition stage, the thermo-oxidative degradation takes place between 550–750°C with a maximum degradation at ∼ 630–700°C (Pan et al., 2015).
Regarding the thermal behavior of the thermoset systems in the inert medium (Figure 5B), it is described by a single stage of pyrolysis between 340–450°C. In this case, the thermal decompositions of the TGPh-based systems are slightly lower (69–79%) than those of THPMTGE thermosets (73–81%), so less organic volatiles are produced during decomposition. Then, the crosslinked systems with MNA generate slightly more thermally stable systems compared to those with HMPA.
The carbon residue at 850°C in nitrogen atmosphere (Cy850) was determined and used to calculate the Limit Oxygen Index (LOI) based on the equation (van Krevelen, 1975; Kumar and T’Ien, 2012):
From Table 4, we can notice that the Cy850 values of the TGPh-thermosets are superior to those of THPMTGE ones, and the MNA-based thermosets present higher char yield values compared to those crosslinked with HMPA. It is known that the layer formed of carbon residue can act as a protective barrier against fire, thus giving the material good flame retardant properties (van Krevelen, 1975). The good fireproofing performance of the thermoset materials was confirmed by the obtained LOI values. Materials with LOI > 26% are classified as “self-extinguishing” systems (Tobias Weiss et al., 2011). As can be seen in Table 4, all the designed systems, except THPMTGE-HMPA (LOI = 25.36%), have values superior to 26%. Then, the TGPh thermosets exhibit higher flame-retardant properties (LOI = 26–30%) compared to the non-toxic commercial-based epoxy systems (LOI = 25–28%).
The moisture behavior of the polymers can lead to various reversible or irreversible changes in the materials properties that could affect their performances. The material’s water absorption capacity can be influenced by a multitude of factors, both physical (crosslinking protocol, parameters, microcracks, voids) and chemical (presence of polar groups and free hydroxyl) (Nogueira et al., 2001; Bouvet et al., 2016; Pereira and D’Almeida, 2016; Toscano et al., 2016; Capiel et al., 2018).
In the case of the thermosets produced in this study, the water uptake values after 24 h of immersion are very low, being ranged between 0.46–0.52% for TGPh thermosets and 0.32–0.39% for THPMTGE ones (Figure 6A).
FIGURE 6. Graphical representation of (A) absorbed water % after 24h of immersion and (B) gel content % by solvent method (in toluene).
It could also be observed that the systems with MNA have a higher hydrophilicity than those with HMPA. The designed bio-resins present a very good hydrostability being comparable with commercial products used for aerospace and electronics areas (Cookson Group STAYCHIP® 3,105 = 1.5%; Henkel Loctite® ABLESTIK 2053S Epoxy = 1%; Hexcel® F161 Epoxy Resin = 2.8%, etc.).
The gel content of the designed systems was determined by solvent method. The obtained values were superior to 99.5% for all the systems, proving the proper and complete crosslinking of the thermoset materials.
The biobased carbon content (BCC) of the designed systems was determined in accordance to the Pan et al. (2011) using the equation:
where W100% is the mass of the 100% biobased constituent, TC100% - total carbon of the 100% biobased component, W0% - mass of the 0% biobased constituent, and TC0% - total carbon of the 0% biobased component. In this study, the TGPh epoxy monomer is made from renewable raw materials being 100% biobased, while the THPMTGE, MNA and the initiator (2E4M) are currently totally made by fossil derivatives being 0% biobased. The biobased organic carbon (Norton and Devlin, 2006) (BOC) was also calculated:
The phthalic anhydride (and its derivatives as HMPA) is still produced from petrochemical derivatives, (Hester E. Andrews, 1920), but intensive studies have led to the development and even the implementation (at the laboratory level, kilograms) of new natural and renewable routes to produce it (Mahmoud et al., 2014; Thiyagarajan et al., 2015; Giarola et al., 2016). Therefore, the BCC and BOC of the designed systems were calculated (Table 5) considering both situations for the HMPA hardener: as being petroleum-based (Merck, Sigma Aldrich), and biobased (by new methods).
As can be seen in Table 5, in the first case where the monomer and the hardeners are fossil-based, the BCC and BOC are 0% (THPMTGE-based thermosets), while when the monomer is biobased (TGPh), the BCC values are about 55–58% and BOC = 74–77%. Based on regulation, (NCS-16785, 2016), materials which have biobased carbon content >30% are classified as biobased.
If we consider the HMPA as a biobased hardener, the BCC and BOC are close to 100% in the systems developed with biobased TGPh, obtaining values of 99% BCC and 99.5% BOC.
It is known that, generally, the thermosets do not have the recycling characteristics of some thermoplastics (Pascault and Williams, 2010; Parameswaranpillai et al., 2021). In this study, the designed thermosets were subjected to depolymerization by dynamic bond exchange reaction (BER) in 1,5,7-triazabicyclo [4.4.0]dec-5-ene (TBD)-ethylene glycol (EG) solution. Generally, these types of bonds are stable in normal conditions and become dynamic under the action of different stimuli (Chakma and Konkolewicz, 2019). The TBD/EG solution was used to depolymerize the polyester thermosets through transesterification reactions between ester linkages and hydrohyl groups (Scheme 1). Thus, when thermoset samples are introduced into a TBD/EG solution under temperature influence, reactive hydroxyl groups present in alcohol-catalyzed molecules diffuse into the network and swell the polymer structure; once entered the polymeric network, the ‒OH groups attack the ester bonds and break the long polymeric chains into short segments thus leading to the decomposition of the thermoset network (Kuang et al., 2018a; 2018b; Shi et al., 2019; Mu et al., 2022; Wu et al., 2022; Jung et al., 2023).
Figure 7 is represented the physical appearance of the epoxy-based thermosets before and after dissolution test at 170°C. As can be seen, all the thermosets were completely decomposed in the alcoholic solution, only the duration was different.
The dissolution of the biobased thermosets was much faster, being completely depolymerized in about 2.5 h, while the complete dissolution time of the materials based on petro-based epoxy monomer was almost double (4.5 h). This behavior can be explained by the higher content in aromaticity of THPMTGE-based materials, which confers a greater stability as in the case of water absorption that was slightly low for these systems (Shi et al., 2019; Shi et al., 2022).
In this study, environmentally friendly and chemically recyclable thermosets were developed starting or from a natural and renewable resource, such as triglycidyl ether of phloroglucinol (TGPh), or from a commercial non-toxic tris(4-hydroxyphenyl) methane triglycidyl ether (THPMTGE). Their physico-chemical and thermo-mechanical properties were investigated and compared. According to DSC investigation it was found that the systems developed with the biobased epoxy monomer have both the maxim temperature of the reaction and the interval of the reaction shifted towards lower temperatures compared to the THPMTGE-based ones, these criteria being critical for industrial manufacturing. Based on DMA results, the biobased TGPh-thermosets have higher tan δ values (189–199°C), higher crosslinking densities (7.6–7.8 mmol cm−3) and lower average molecular mass between crosslinks (144–152 g/mol) compared to the THPMTGE-resins, thus generating compact and stiff networks, crucial properties in the high-performance industry. The remarkable mechanical properties of these thermosets were also supported by the results of tensile tests, where Young’s modulus ranged from 1.3 to 1.4 GPa, tensile stress of about 55–69 MPa, and an elongation at break around 3–8%. The brittleness factor was also calculated, thus demonstrating the lower presence of fragile character in the developed resins: for TGPh B = 0.044–0.059%·Pa/1010), and for THPMTGE B = 0.052–0.104%·Pa/1010). Also, the developed thermosets were completely dissolute in TBD/EG solution at temperature of 170°C, the time required for the depolymerization of TGPh-based resins being of about 2.5 h, and for the formulations based on THPMTGE monomer was around 4.5 h.
Concluding, sustainable and eco-friendly thermosets were developed, with excellent physico-chemical and thermomechanical properties, with low hydrophilicity and with a fast dissolution capacity. These thermosets are real competitors for the non-recyclable and toxic resins used in high-end industry.
The raw data supporting the conclusion of this article will be made available by the authors, without undue reservation.
RD and AM contributed to conception and design of the study, methodology, investigation, and validation; RD: data curation; RD and AM: writing—original draft preparation; RD, UL, OD, and AM: writing—review and editing. All authors contributed to the article and approved the submitted version.
This work received financial support from the French government, managed by the National Research Agency under the “Investissements d’Avenir UCAJEDI” project with Reference No. ANR-15-IDEX-01 and from the European Space Agency under ESA Contract 4000134653.
The authors declare that the research was conducted in the absence of any commercial or financial relationships that could be construed as a potential conflict of interest.
All claims expressed in this article are solely those of the authors and do not necessarily represent those of their affiliated organizations, or those of the publisher, the editors and the reviewers. Any product that may be evaluated in this article, or claim that may be made by its manufacturer, is not guaranteed or endorsed by the publisher.
ASTM D570-98 (2018). Water absorption of plastics. Pennsylvania, United States: ASTM. doi:10.1520/D0570-98R18
ASTM-D2765-16 (2016). Determination of gel content and swell ratio of crosslinked ethylene plastics. Pennsylvania, United States: ASTM.
Bouvet, G., Dang, N., Cohendoz, S., Feaugas, X., Mallarino, S., and Touzain, S. (2016). Impact of polar groups concentration and free volume on water sorption in model epoxy free films and coatings. Prog. Org. Coatings 96, 32–41. doi:10.1016/j.porgcoat.2015.12.011
Briou, B., Jégo, L., De Dios Miguel, T., Duguet, N., and Caillol, S. (2023). Eco-friendly synthesis of cardanol-based AB monomer for formaldehyde-free phenolic thermosets. RSC Sustain 1, 994–1005. doi:10.1039/D3SU00058C
Brostow, W., and Hagg Lobland, H. E. (2010). Brittleness of materials: Implications for composites and a relation to impact strength. J. Mater. Sci. 45, 242–250. doi:10.1007/s10853-009-3926-5
Brostow, W., Hagg Lobland, H. E., and Narkis, M. (2006). Sliding wear, viscoelasticity, and brittleness of polymers. J. Mater. Res. 21, 2422–2428. doi:10.1557/jmr.2006.0300
Brostow, W., Hagg Lobland, H. E., and Narkis, M. (2011). The concept of materials brittleness and its applications. Polym. Bull. 67, 1697–1707. doi:10.1007/s00289-011-0573-1
Capiel, G., Uicich, J., Fasce, D., and Montemartini, P. E. (2018). Diffusion and hydrolysis effects during water aging on an epoxy-anhydride system. Polym. Degrad. Stab. 153, 165–171. doi:10.1016/j.polymdegradstab.2018.04.030
Capricho, J. C., Fox, B., and Hameed, N. (2020). Multifunctionality in epoxy resins. Polym. Rev. 60, 1–41. doi:10.1080/15583724.2019.1650063
Chakma, P., and Konkolewicz, D. (2019). Dynamic covalent bonds in polymeric materials. Angew. Chem. - Int. Ed. 58, 9682–9695. doi:10.1002/anie.201813525
Chowdhury, M. T. H., Bangoura, I., Kang, J. Y., Park, N. G., Ahn, D. H., and Hong, Y. K. (2011). Distribution of phlorotannins in the Brown alga Ecklonia cava and comparison of pretreatments for extraction. Fish. Aquat. Sci. 14, 198–204. doi:10.5657/FAS.2011.0198
Derradji, M., Mehelli, O., Liu, W., and Fantuzzi, N. (2021). Sustainable and ecofriendly chemical design of high performance bio-based thermosets for advanced applications. Front. Chem. 9, 691117–691118. doi:10.3389/fchem.2021.691117
Extrusionplastic (2021). Heat resistance index and classification of plastic profile products. Available at: https://www.extrusionplastic.cn/news/plastic-profile-products.html (Accessed July 26, 2021).
Genua, A., Montes, S., Azcune, I., Rekondo, A., Malburet, S., Daydé-Cazals, B., et al. (2020). Build-to-specification vanillin and phloroglucinol derived biobased epoxy-amine vitrimers. Polym. (Basel) 12, 2645. doi:10.3390/polym12112645
Geyer, R., Jambeck, J. R., and Law, K. L. (2017). Production, use, and fate of all plastics ever made. Sci. Adv. 3, e1700782. doi:10.1126/sciadv.1700782
Giarola, S., Romain, C., Williams, C. K., Hallett, J. P., and Shah, N. (2016). Techno-economic assessment of the production of phthalic anhydride from corn stover. Chem. Eng. Res. Des. 107, 181–194. doi:10.1016/j.cherd.2015.10.034
Guzmán, D., Santiago, D., Serra, A., and Ferrando, F. (2020). Novel bio-based epoxy thermosets based on triglycidyl phloroglucinol prepared by thiol-epoxy reaction. Polym. (Basel) 12, 337. doi:10.3390/polym12020337
Jung, H., Shin, G., Kwak, H., Hao, L. T., Jegal, J., Kim, H. J., et al. (2023). Review of polymer technologies for improving the recycling and upcycling efficiency of plastic waste. Chemosphere 320, 138089. doi:10.1016/j.chemosphere.2023.138089
Kuang, X., Shi, Q., Zhou, Y., Zhao, Z., Wang, T., and Qi, H. J. (2018a). Dissolution of epoxy thermosets via mild alcoholysis: The mechanism and kinetics study. RSC Adv. 8, 1493–1502. doi:10.1039/C7RA12787A
Kuang, X., Zhou, Y., Shi, Q., Wang, T., and Qi, H. J. (2018b). Recycling of epoxy thermoset and composites via good solvent assisted and small molecules participated exchange reactions. ACS Sustain. Chem. Eng. 6, 9189–9197. doi:10.1021/acssuschemeng.8b01538
Kumar, A., and T’Ien, J. (2012). Numerical modeling of limiting oxygen index apparatus for film type fuels. Int. J. Spray. Combust. Dyn. 4, 299–322. doi:10.1260/1756-8277.4.4.299
Liu, X., Xin, W., and Zhang, J. (2009). Rosin-based acid anhydrides as alternatives to petrochemical curing agents. Green Chem. 11, 1018. doi:10.1039/b903955d
Ma, S., Kovash, C. S., and Webster, D. C. (2017). Effect of solvents on the curing and properties of fully bio-based thermosets for coatings. J. Coatings Technol. Res. 14, 367–375. doi:10.1007/s11998-016-9863-8
Ma, S., Liu, X., Fan, L., Jiang, Y., Cao, L., Tang, Z., et al. (2014). Synthesis and properties of a bio-based epoxy resin with high epoxy value and low viscosity. ChemSusChem 7, 555–562. doi:10.1002/cssc.201300749
Mahmoud, E., Watson, D. A., and Lobo, R. F. (2014). Renewable production of phthalic anhydride from biomass-derived furan and maleic anhydride. Green Chem. 16, 167–175. doi:10.1039/C3GC41655K
Moreman, J., Lee, O., Trznadel, M., David, A., Kudoh, T., and Tyler, C. R. (2017). Acute toxicity, teratogenic, and estrogenic effects of bisphenol A and its alternative replacements bisphenol S, bisphenol F, and bisphenol af in zebrafish embryo-larvae. Environ. Sci. Technol. 51, 12796–12805. doi:10.1021/acs.est.7b03283
Mu, Q., An, L., Hu, Z., and Kuang, X. (2022). Fast and sustainable recycling of epoxy and composites using mixed solvents. Polym. Degrad. Stab. 199, 109895. doi:10.1016/j.polymdegradstab.2022.109895
Nogueira, P., Ramírez, C., Torres, A., Abad, M. J., Cano, J., López, J., et al. (2001). Effect of water sorption on the structure and mechanical properties of an epoxy resin system. J. Appl. Polym. Sci. 80, 71–80. doi:10.1002/1097-4628(20010404)80:1<71::AID-APP1077>3.0.CO;2-H
Norton, G. A., and Devlin, S. L. (2006). Determining the modern carbon content of biobased products using radiocarbon analysis. Bioresour. Technol. 97, 2084–2090. doi:10.1016/j.biortech.2005.08.017
Okunola A, A., Kehinde I, O., Oluwaseun, A., and Olufiropo E, A. (2019). Public and environmental health effects of plastic wastes disposal: A review. J. Toxicol. Risk Assess. 5. doi:10.23937/2572-4061.1510021
Pan, H., Sun, G., Zhao, T., and Wang, G. (2015). Thermal properties of epoxy resins crosslinked by an aminated lignin. Polym. Eng. Sci. 55, 924–932. doi:10.1002/pen.23960
Pan, X., Sengupta, P., and Webster, D. C. (2011). High biobased content epoxy-anhydride thermosets from epoxidized sucrose esters of fatty acids. Biomacromolecules 12, 2416–2428. doi:10.1021/bm200549c
J. Parameswaranpillai, S. M. Rangappa, S. Siengchin, and S. Jose (Editors) (2021). “Polymer science and technology general,” Bio-based epoxy polymers, blends, and composites - synthesis, properties, characterization, and applications (Weinheim: WILEY-VCH GmbH).
Pascault, J. P., and Williams, R. J. J. (2010). Epoxy polymers-new materials and innovations. Weinheim, Germany: WILEY-VCH Verlag GmbH and Co. KGaA. Available at: http://link.springer.com/10.1007/BF01983263.
Pereira, A. A. C., and D’Almeida, J. R. M. (2016). Effect of the hardener to epoxy monomer ratio on the water absorption behavior of the DGEBA/TETA epoxy system. Polímeros 26, 30–37. doi:10.1590/0104-1428.2106
Santiago, D., Guzmán, D., Ferrando, F., Serra, À., and De la Flor, S. (2020). Bio-based epoxy shape-memory thermosets from triglycidyl phloroglucinol. Polym. (Basel) 12, 542. doi:10.3390/polym12030542
Shi, X., He, X., Luo, C., Chung, C., Ding, Y., and Yu, K. (2022). Influences of material and processing conditions on the depolymerization speed of anhydride-cured epoxy during the solvent-assisted recycling. Polym. Guildf. 252, 124964. doi:10.1016/j.polymer.2022.124964
Shi, X., Luo, C., Lu, H., and Yu, K. (2019). Primary recycling of anhydride-cured engineering epoxy using alcohol solvent. Polym. Eng. Sci. 59, E111–E119. doi:10.1002/pen.24997
Sukanto, H., Raharjo, W. W., Ariawan, D., Triyono, J., and Kaavesina, M. (2021). Epoxy resins thermosetting for mechanical engineering. Open Eng. 11, 797–814. doi:10.1515/eng-2021-0078
Thiyagarajan, S., Genuino, H. C., Śliwa, M., Van Der Waal, J. C., De Jong, E., Van Haveren, J., et al. (2015). Substituted phthalic anhydrides from biobased furanics: A new approach to renewable aromatics. ChemSusChem 8, 3052–3056. doi:10.1002/cssc.201500511
Toscano, A., Pitarresi, G., Scafidi, M., Di Filippo, M., Spadaro, G., and Alessi, S. (2016). Water diffusion and swelling stresses in highly crosslinked epoxy matrices. Polym. Degrad. Stab. 133, 255–263. doi:10.1016/j.polymdegradstab.2016.09.004
van Krevelen, D. W. (1975). Some basic aspects of flame resistance of polymeric materials. Polym. Guildf. 16, 615–620. doi:10.1016/0032-3861(75)90157-3
Wang, Z., Ganewatta, M. S., and Tang, C. (2020). Sustainable polymers from biomass: Bridging chemistry with materials and processing. Prog. Polym. Sci. 101, 101197. doi:10.1016/j.progpolymsci.2019.101197
Weiss, T., Schuster, H., Müller, J., Schaller, K. H., Drexler, H., Angerer, J., et al. (2011). Dermal uptake and excretion of 4,4′-methylenedianiline during rotor blade production in helicopter industry—an intervention study. Ann. Occup. Hyg. 55, 886–892. doi:10.1093/annhyg/mer051
Wu, J., Pan, Y., Ruan, Z., Zhao, Z., Ai, J., Ban, J., et al. (2022). Planococcus maritimu ML1206 strain enhances stress resistance and extends the lifespan in Caenorhabditis elegans via FOXO/DAF-16. Front. Mater. 9, 1–9. doi:10.3390/md21010001
Keywords: thermosets, biobased materials, natural and renewable raw materials, high-end properties, recyclability
Citation: Dinu R, Lafont U, Damiano O and Mija A (2023) Sustainable and recyclable thermosets with performances for high technology sectors. An environmental friendly alternative to toxic derivatives. Front. Mater. 10:1242507. doi: 10.3389/fmats.2023.1242507
Received: 19 June 2023; Accepted: 17 July 2023;
Published: 27 July 2023.
Edited by:
Chang Boon Peng, Nanyang Technological University, SingaporeReviewed by:
Junheng Zhang, South-Central University for Nationalities, ChinaCopyright © 2023 Dinu, Lafont, Damiano and Mija. This is an open-access article distributed under the terms of the Creative Commons Attribution License (CC BY). The use, distribution or reproduction in other forums is permitted, provided the original author(s) and the copyright owner(s) are credited and that the original publication in this journal is cited, in accordance with accepted academic practice. No use, distribution or reproduction is permitted which does not comply with these terms.
*Correspondence: Alice Mija, QWxpY2UuTUlKQUB1bml2LWNvdGVkYXp1ci5mcg==
Disclaimer: All claims expressed in this article are solely those of the authors and do not necessarily represent those of their affiliated organizations, or those of the publisher, the editors and the reviewers. Any product that may be evaluated in this article or claim that may be made by its manufacturer is not guaranteed or endorsed by the publisher.
Research integrity at Frontiers
Learn more about the work of our research integrity team to safeguard the quality of each article we publish.