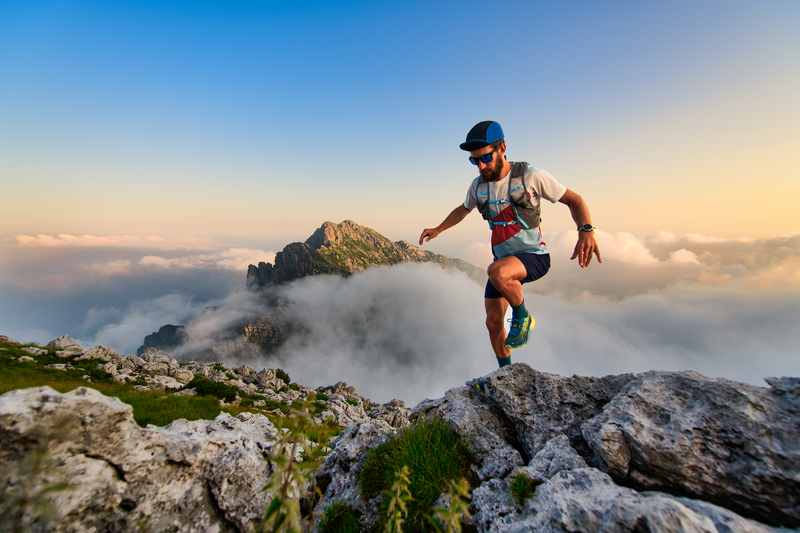
94% of researchers rate our articles as excellent or good
Learn more about the work of our research integrity team to safeguard the quality of each article we publish.
Find out more
REVIEW article
Front. Mater. , 29 November 2023
Sec. Biomaterials and Bio-Inspired Materials
Volume 10 - 2023 | https://doi.org/10.3389/fmats.2023.1220420
Periodontal disease is one of the most common oral diseases with the highest incidence world-wide. In particular, the treatment of periodontal bone defects caused by periodontitis has attracted extensive attention. Guided bone regeneration (GBR) has been recognized as advanced treatment techniques for periodontal bone defects. GBR technique relies on the application of barrier membranes to protect the bone defects. The commonly used GBR membranes are resorbable and non-resorbable. Resorbable GBR membranes are divided into natural polymer resorbable membranes and synthetic polymer resorbable membranes. Each has its advantages and disadvantages. The current research focuses on exploring and improving its preparation and application. This review summarizes the recent literature on the application of GBR membranes to promote the regeneration of periodontal bone defects, elaborates on GBR development strategies, specific applications, and the progress of inducing periodontal bone regeneration to provide a theoretical basis and ideas for the future application of GBR membranes to promote the repair of periodontal bone defects.
As one of the most prevalent and high-incident oral diseases globally, periodontal disease involves the deterioration of tooth-supporting structures and is considered the main cause of tooth loss among adults (Kocher et al., 2018). According to the latest report related to prevalence of periodontitis in the U.S. from the Centers for Disease Control and Prevention (CDC), 47.2% of adults aged 30 years and older have some form of periodontal disease. Periodontal disease increases with age, 70.1% of adults 65 years and older have periodontal disease. Periodontitis is an inflammatory disease characterized by the destruction of the alveolar bone, periodontal ligament (PDL), cementum, and gingiva as a response to insults elicited by microbial accumulations (Jensen et al., 2018). Periodontitis leads to the inflammation of soft tissue and progressive and irreversible bone resorption, which can cause tooth loss and the failure of implants around bone defects (Kavarthapu and Gurumoorthy, 2021) (Figures 1A–C). In conventional conservative and surgical therapy, the activated adjacent tissues proliferate into the defect site before the migration of osteoblasts, which constrains the regeneration of the bone and the periodontal ligament, leading to undesirable clinical results such as non-union or implant encapsulation. This has also become a major obstacle to traditional treatment strategies (Graziani et al., 2017). Therefore, clinical studies suggest that to achieve successful periodontal treatment; we should focus on two aspects: 1) to completely regenerate the supporting bone of the tooth and 2) to eliminate the interference of non-osteogenic tissue on bone regeneration (Sowmya et al., 2013). Presently, guided tissue regeneration (GTR) and guided bone regeneration (GBR) are two common periodontal regeneration treatments aimed at rebuilding damaged periodontal tissue and bone caused by periodontitis. Periodontal regeneration, often referred to as GTR, is a process in which all deep periodontal tissues (alveolar bone, periodontal ligament, and cementum) need to be regenerated. GBR is a regenerative surgery for the purpose of bone regeneration and is often used in the field of dental implants. It is considered to be one of the most successful methods for the reconstruction of alveolar bone and the treatment of bone defects around implants (Benic and Hämmerle, 2014).
FIGURE 1. Schematic of GBR application through the use of bone filler and a barrier membranes (A–E) and the mechanism of therapying periodontal bone defects with GBR membranes (F).
The GBR procedure creates and maintains a space for bone regeneration by placing the material as a barrier and filler for defects to achieve lateral and longitudinal bone expansion (Figures 1D, E). As a temporary matrix for cell proliferation, extracellular matrix differentiation, and mineralization, the biomaterial’s characteristics determine the technology’s therapeutic effect. For this purpose, researchers have developed various forms of biomaterials, including membranes, sponges, hydrogel composites, glass ceramic scaffolds, or bovine xenograft materials (Mellonig et al., 1998). In the above materials, the membranes can prevent epithelial and connective tissue cells from growing and proliferating into the defects, thus avoiding inadequate bone formation. Membrane treatment has become the first choice for repairing periodontal bone defects (Gruber et al., 2017; Shahdad et al., 2020). As a barrier, the GBR membrane covers the periodontal defect around the tooth root, isolates the periodontal bone defect from the gingival connective tissue, prevents the rapid growth of epithelial cells, fibers and gingival tissue from growing into the defect, ensures the stability of blood clots, and promotes bone regeneration through cell rejection, thus realizing periodontal regeneration (Villar and Cochran, 2010). At present, given to the differences in the degradability and absorbability of the materials used to prepare GBR membrane, they can be divided into two categories: non-degradable absorption membranes and degradable absorption membranes (Ul Hassan et al., 2021). Non-biodegradable membranes need to be removed by supplementary surgery and can lead to inflammation at the surgical site (Shahdad et al., 2020). Therefore, various biodegradable polymer membranes have been developed to eradicate the need for follow-up surgery (Gao et al., 2022). However, the degradable resorbable membranes often have defects such as insufficient mechanical properties and a fast degradation rate, which often lead to the failure of the control of non-osteogenic tissue invasion in the clinical application process, and ultimately lead to the failure of surgery and the incapacity to attain effective periodontal bone regeneration (Sun et al., 2018). Many studies have shown that the GBR membranes applied in the regeneration of periodontal bone defects should have adequate mechanical and physical stability, biocompatibility, cell occlusion, and can prevent the invasion of soft tissue to the defects before bone regeneration without causing the intervention of bacteria and microorganisms (Almeida et al., 2019; Gao et al., 2022).
To solve these problems, researchers have prepared a variety of new GBR membranes by modifying GBR membranes and combining them with other small molecules, which have improved the properties of GBR membranes and further enhanced their functions of isolating soft tissue and inducing bone and vascular regeneration. In addition to the improvement of the preparation strategy, many studies have also explored the application of GBR membranes, mainly including its promotion of bone regeneration, angiogenesis and antibacterial ability. However, the current research on GBR membrane is still not mature enough. In addition, the latest preparation strategies to improve the performance and the GBR membrane’s specific application and progress of the GBR membranes in the induction of periodontal bone defects are not completely clear.
Herein we reviewed the relevant literature on the application of GBR membranes to promote the regeneration of periodontal bone defects in recent years. This paper mainly describes the preparation of commonly used natural and synthetic resorbable membranes, as well as the latest preparation strategies of new hybrid membranes. In addition, the specific application and progress of inducing periodontal bone regeneration were described in detail, so as to provide theoretical basis and ideas for further application of GBR membrane to promote periodontal bone defect repair in the future.
In 1982, Nyman et al. (1982) formed a new attachment to the tooth in humans by directing periodontal tissue regeneration using a barrier membrane. Subsequently, the study of dental regeneration has attracted attention. Surgical protocols such as GBR have been widely accepted for the management of periodontal regeneration (An et al., 2022).
The principle of GBR is based on the shielding function of the membrane, which maintains space for tissue regeneration and selectively guides periodontal ligament-derived cells or bone formation cells into the defect area (Ahn et al., 2020). GBR membranes can hinder epithelial and connective cells into bone defect areas to increase the ability of damaged periodontal tissue to regenerate, with new bone, periodontal ligament, and cementum formation (Zhou et al., 2021). It has been reported that periodontal tissue regeneration requires continuous membrane shielding for 4–6 weeks, while bone regeneration enhancement requires continuous membrane shielding for 16–24 weeks (Hoornaert et al., 2016; Caballé-Serrano et al., 2017). As a result, GBR membrane needs to remain between the gum and alveolar bone longer. In addition, an ideal GBR membrane should have the following characteristics: biocompatibility (prevent adverse reactions with the surrounding tissue and with the organism; prompts the membrane to integrate into the tissue without triggering an inflammatory response); dimensional stability (positioning and shape of the membranes should remain unaltered till degradation); tissue integration (favor the embedding in the surrounding tissue and allow a progressive integration of collagen fibers); handling (the membranes should be managed and easily placed over the defect); selective permeability (membranes should be able to exclude unwanted epithelial cells while allowing osteogenic cells to proliferate); space-forming function (to provide space for a stable blood clot, to allow bone regeneration) (Calciolari et al., 2018).
In the clinical treatment of periodontal bone defects, a non-resorbable membrane is the earliest barrier membrane used in GBR. Its excellent biological characteristics can provide a durable barrier effect (Zelikman et al., 2022). The first non-resorbable membrane made of polytetrafluoroethylene (PTFE) for clinical application has been shown to have excellent mechanical strength, good biocompatibility, significant chemical and thermal stability, and low surface friction, which can effectively induce periodontal bone regeneration (Xie et al., 2020). However, non-resorbable membranes require a second surgical removal at a later stage of injury healing, which is more invasive for the patient (Lin and Chiu, 2021). In addition, the non-resorbable membranes cannot be exposed to the oral environment. If the membranes accidently exposed early, it may lead to more bacterial contamination and a greater incidence of dehiscence, compromising the regeneration outcome and increasing the technical difficulty of its application during surgery (Di Raimondo et al., 2021). Researchers introduced resorbable barrier membranes to overcome the limitation of non-resorbable membranes made from degradable materials. Most resorbable membranes are made of collagen, and collagen membrane (CM) is degraded into carbon dioxide and water by endogenous collagenase in the body (Bunyaratavej and Wang, 2001). Apart from the unnecessary second surgical intervention to eliminate the membrane, bioresorbable membranes present added advantages: improved soft tissue healing, the integration of the membranes by the host tissues (subject to material properties), and quick resorption in case of exposure, thus eliminating open microstructures prone to bacterial contamination and self-limiting infection (Ul Hassan et al., 2021). Turri et al. (2016) have shown that CMs act as bioactive compartments rather than passive barriers. They attract cells into the bone defect area, which secrete signals for bone regeneration and remodeling, and promote the expression of chemotactic factors, thus modulating the overall osteogenic process. Moreover, CM may adsorb mediators and growth factors released by bone and cells, thereby enhancing the effect of GBR. However, the main shortcomings of resorbable membranes are their fast degradation rate and poor mechanical properties (Di Raimondo et al., 2021). Studies in several animal models have shown that native CMs have markedly reduced their thickness between 2, 4 weeks after implantation and are completely absorbed between 4, 12 weeks, which may limit their barrier properties, especially in large bone defects (Schwarz et al., 2006; Schwarz et al., 2008). In addition, the poor mechanical properties of the resorbable membranes may fail to withstand the pressure of overhanging gingiva, resulting in plastic deformation to compress the space for bone regeneration and compromise the efficiency of bone regeneration (Meinig, 2010). Therefore, resorbable membranes are often combined with dental implants or bone graft materials in clinical applications. Resorbable membranes usually require a large amount of filling material to maintain space; they may even require micro-star screws to fix the membranes to the defect to reduce the risk of membrane collapse and improve its retention and stability (Masoudi Rad et al., 2017; Jabbari et al., 2019). However, the micro-star screws needs to be removed a second time and cannot be implanted minimally. So while this increases the success rate of treatment, it also increases the cost of surgery [7–9]. Additionally, most resorbable membranes used in clinical practice are of animal origin and therefore cannot completely eliminate the risk of infection by transmission of unknown animal diseases (Thieu et al., 2021; Zhang et al., 2021).
In recent years, to further improve the application of GBR membranes, a new generation of synthetic biodegradable polymer membrane has been developed in bone tissue engineering, aiming to expand the physical properties and biodegradation rate of the resorbable membrane. Synthetic biodegradable polymer membranes usually include polyester, polywater, polyurethanes, etc. This new class of GBR membrane has many advantages such as controlled biodegradation, adequate mechanical strength, low stiffness, manageability, processability, and drug encapsulation, and it has been widely used in GBR. However, pure polymers may have drawbacks such as hydrophobic properties or relatively low bioactivity in the application of GBR (Abdelaziz et al., 2021). Therefore, currently, developing novel GBR membranes with desirable properties by blending various polymers and/or combining them with other substances such as bioactive components has become a research hotspot in recent years (Aprile et al., 2020; Ward, 2022).
In summary, the GBR technique is the most commonly used method to guide periodontal bone tissue regeneration and shows an excellent therapeutic effect. This technique is based on the rejection of soft tissue by the GBR membranes to maintain an optimum spatial environment for bone regeneration. Currently, the commonly used GBR membranes include non-resorbable membranes and resorbable membranes. Among them, non-resorbable membranes need to be prevented from early exposure to the oral environment and require to be removed by a second operation later, increasing the difficulty of operation and the risk of complications such as infection. Therefore, the development of resorbable membranes has become the focus of the application of GBR technology. However, the resorbable membranes made from natural polymers have low mechanical properties and a fast degradation rate. While the resorbable membranes made from synthetic polymers have controllable degradation rates, mechanical properties, high processing flexibility, and drug embedding ability, so the synthetic biodegradable polymer membranes have been widely used. In addition, resorbable polymers are often used in combination with other bioactive components or inorganic substances to obtain a better ability to induce periodontal bone tissue regeneration.
In recent years, the research on the advantages and disadvantages of different GBR membranes and their performance optimization have attracted extensive attention (Abe et al., 2020; Guo et al., 2020). It is still a significant challenge to fabricate a GBR membrane with all the expected properties because the structure morphology and material composition are the key factors affecting the physicochemical and biological properties of the GBR membrane (Raina et al., 2019). Due to problems such as uncontrolled early exposure, bacterial contamination, and a higher incidence of dehiscence that may jeopardize regeneration outcomes in non-resorbable membranes, bio-resorbable membranes have emerged the first choice for GBR procedures (Abe et al., 2020). At present, researchers mainly focus on the development and the improvement of the application in periodontal bone defects of natural polymer membranes resorbable membranes, synthetic polymer membranes resorbable membranes, and novel GBR membranes, aiming to obtain an ideal GBR membrane that can effectively achieve barrier function and accelerate the reconstruction of periodontal bone defects.
Resorbable membranes can be fabricated from natural polymers, including proteins (e.g., collagen, silk, fibrinogen, and elastin) and polysaccharides (e.g., cellulose, chitin, and glycosaminoglycans). Resorbable membranes can be prepared from natural polymers, including proteins (e.g., collagen, silk, fibrinogen, and elastin) and polysaccharides (e.g., cellulose, chitin, and glycosaminoglycans) (Mano et al., 2007). Collagen is the main organic component of natural bone, which has good biocompatibility and tissue repair ability. Collagen is an ideal material for preparing GBR membranes (Chu et al., 2017). The natural collagen membrane is the most popular choice amongst patients and dentists due to the ease of operation and the fact that it does not require follow-up surgery. This method is mainly used to correct a small range of bone defects, including fenestration and fracture (Chiapasco and Casentini, 2018; Neto et al., 2020). Natural polymers are generally considered safer, more biocompatible, and more biodegradable than synthetic polymers. They also can present receptor-binding ligands to cells in addition to susceptibility to cell-triggered proteolytic degradation and natural remodeling (Pişkin, 1995; Sbricoli et al., 2020). Recently, the most widely used CMs are those made of native porcine collagen, shown in both small and large animal models, good barrier capability with the concomitant promotion of cell migration and angiogenesis (Schwarz et al., 2008; Sanz et al., 2019). The success of GTR membranes depends on achieving the repair of the large area through osteoconductivity, mechanical stability, and equilibrium between the degradation rate of membranes and tissue regeneration to restrict epithelial cells’ ingrowth (Sheikh et al., 2016; Sun et al., 2017). Poor mechanical properties and fast degradation rate are the main reasons for the failure of natural polymer resorbable membranes. Therefore, the improvement methods have been explored and made effective progress in several studies (Luo et al., 2021; Chen et al., 2022).
Regarding the effect of the type of additional fixation on the process of bone formation, it is known that intermediate tissues, such as fibrous tissue, cartilage, and woven bone, precede final bone formation, with the mechanical loading affecting the regeneration process and different stress distribution supporting or inhibiting differentiation of particular tissue phenotypes. During the repair and regeneration of periodontal bone defects, it is known that intermediate tissues, such as fibrous tissue, cartilage, and woven bone, precede final bone formation. GBR membranes not only need to withstand the forces generated during surgery but also need more time to cope with the stresses generated by tissue growth and physiological activities (Lacroix and Prendergast, 2002; Tejeda-Montes et al., 2014). In addition, it is necessary to cut and shape the membranes according to periodontal defects with different morphology during the placement of the membranes into the bone defect. At this stage, membrane hardness is of great importance (Rowe et al., 2016). Achieving a balance between stiffness and flexibility in membrane formulations developed for GBR is necessary and important for the success of the GBR procedure (Rowe et al., 2016). Appropriate mechanical strength makes GBR membranes not only prevent membranes collapse from maintaining tissue living space, give full play to its barrier role, but also easy to operate in vivo to avoid damage to surrounding tissues (Bottino et al., 2012). In addition, the mechanical stability of GBR membranes also plays an important role. Studies have shown that micromovements between bone and any implanted material prevent bone formation, resulting in the development of fibrous tissue (Ducheyne et al., 1977). Adequate stability and minimal stress are required to allow the early tissue infiltrating through the pores to differentiate into the bone by direct or appositional bone formation (Gutta et al., 2009). It has been observed that bone formation is significantly enhanced when the resorbable membrane is tightly attached and immobilized to the bone surface (Amano et al., 2004). To maximize membrane stability, the use of membrane-fixing pins has been suggested. However, the corresponding mechanical load on the process of bone formation is due to different additional fixation methods and then affects bone regeneration (Amano et al., 2004; Dimitriou et al., 2012). Studies have shown that high shear strain and fluid flow stimulate fibrous tissue formation, whereas lower levels stimulate cartilage formation, and even lower levels favor ossification (Stetzer et al., 2002). It has been demonstrated in vivo that there is more rapid and more organized new bone formation stimulated by appropriate mechanical load imposed by resorbable CM-covered plate bone internal fixation compared to non-rigidly fixed defects (Stetzer et al., 2002). In addition, the formation of the new vascular network necessary for bone regeneration is affected by mechanical loading, and appropriate stress stimulation promotes vascular remodeling (Boerckel et al., 2011). Many studies have shown that the success rate of GBR surgery in repairing periodontal bone defects is closely related to the membranes’ spatial maintenance ability and stability. Sufficient mechanical strength and stability are the key factors for the effective induction of bone regeneration by GBR membranes (Wessing et al., 2018; Shahdad et al., 2020). The evaluation of mechanical properties includes many aspects, and tensile tests are often used as the first method to obtain information about the mechanical properties of membranes (Zhou et al., 2021; Chen et al., 2022). The tensile strength of pure PCL membrane was 1.63 ± 0.67 MPa. The value of pure fish collagen was 6.72 ± 0.44 Mpa. The maximum tensile stress of common commercial membranes was also different: Bio-Gide (4.8 MPa), Collprotect (13.1 MPa)and Jason (13.0 MPa). GBR membranes that induce periodontal bone regeneration have been reported to have ultimate tensile strength values between 1 and 20 MPa (Ortolani et al., 2015; Moonesi Rad et al., 2019). In addition to evaluating the strength of the membrane, the strain capacity, elastic modulus, and elongation rate of the membrane are also commonly used to assist in the evaluation of mechanical properties. However, increasing the strength usually leads to a decrease in elongation. For example, the Elastic modulus of pure PCL membrane is 0.18 ± 0.04 MPa, and the failure strain is 106.14 ± 23.29. A new type of PCL membrane was prepared by adding Silica Gel to the PCL membrane. The results showed that the strength and elastic modulus of the hybrid membrane containing 40% silica Gel were 5 and 8 times higher than those of the pure PCL membrane, respectively. The failure strain was 28.92 ± 8.41 (Lee et al., 2016). In addition, studies have shown that the thickness of common commercial collagen membranes varies from 0.2 to 0.4 mm. The thickness of the membrane is closely related to the cell adhesion and swelling properties of the membrane, and it changes instably during tissue regeneration. The change of the thickness of the membrane will have a certain impact on the mechanical properties of the membrane. Therefore, relevant comparisons should be made as comprehensively as possible in the evaluation and reinforcement of membrane mechanical properties (Lee et al., 2016; Pouroutzidou et al., 2022).
Recent clinical studies have shown that the hybrid CMs prepared by blending multi-component natural substances can effectively strengthen the membrane’s mechanical properties and ensure its spatial maintenance ability (Luo et al., 2021). Luo et al. (2021) reported that a composite membrane with good mechanical properties was prepared by mixing Silk fibroin (SF) and collagen using green papermaking. Silk fibroin (SF) from Bombyx mori, a versatile natural fibrous protein, has been extensively used in bone tissue engineering applications owing to its low immunogenicity, slow degradability, and feasible mechanical properties (Melke et al., 2016). Studies have shown that a hybrid membrane consisting of a large amount of SF(75%) and a small amount of collagen (25%) can maintain long-term mechanical properties after implantation. SF provides a remarkable combination of strength and toughness to maintain enough stability (Wu et al., 2017). In addition, the collagen content blended with SF membranes can be selected depending on the level of mechanical property and biodegradation time required of the regenerating tissue, then GBR membranes with specific mechanical strength and stability are prepared (Luo et al., 2021). The good mechanical properties of the composite membranes can also be attributed to its new preparation method. Traditionally, the main and common methods such as electrospinning, rapid prototyping, particulate leaching, and solvent-casting have been applied to fabricate GBR membranes (Masoudi Rad et al., 2017; Jian et al., 2019). However, the protein conformation (composed mainly of β-sheet) and the parallel-fibrillar structure of SF fibers may be disrupted by directly solvent casting and electrospinning, resulting in the mechanical degradation of SF membranes (Keten et al., 2010). To overcome this limitation, tannic acid (TA), a low-cost natural polyphenolic compound extracted from plants, was used to modify electrospun SF nanofiber membranes. The large polyphenolic groups in TA were combined with SF protein to induce the conformation of SF molecule into a tight β sheet, and the mechanical properties of SF membranes were successfully improved (Luo et al., 2020; Zheng et al., 2020). In addition, recent studies have reported that there will be less damage to SF molecular structure and better mechanical properties of nanofibers if the nanofibers are extracted directly from SF by the physical shearing method (Ang et al., 2020; Li et al., 2021). Chen et al. (2022) also successfully obtained SF membranes with good mechanical properties through this method. However, collagen cross-linking is the most common way to improve the mechanical properties of the most widely used native CM (Khorsand et al., 2019; Liu et al., 2020). Ahn et al. (2020) directly used 1-ethyl-3-(3-dimethyl aminopropyl) carbodiimide (EDC) with low cytotoxicity as a cross-linking agent and successfully obtained GBR membranes with good mechanical properties. Studies have shown that the interaction between collagen molecules and the structural integrity of collagen molecules have been improved, finally strengthening the mechanical properties of the membranes (Speer et al., 1980).
In summary, the success rate of GBR surgery is closely related to the mechanical properties of GBR membranes in repairing periodontal bone defects. Good mechanical strength and stability can prevent the collapse of GBR membranes to maintain the space for bone regeneration and give full play to the barrier function of membranes, effectively promoting bone formation. In current studies, the mechanical properties of GBR membranes can be effectively strengthened by blending multi-component natural materials, and even the customized mechanical strength and stability of GBR membranes can be obtained by adjusting the ratio of different components. In addition to changing the material formulations of GBR membranes, many studies have proved that the mechanical properties of natural resorbable membranes can also be effectively improved by modifying some compounds, such as TA. Many novel preparation methods, such as physical shear or EDC-collagen cross-linking, can enhance the mechanical properties of natural resorbable membranes. However, although several strategies effectively improve membrane mechanical properties, their clinical performance has not been systematically tested and compared, and further research and testing are still needed.
Similar to CM, most natural polymer-resorbable membranes have the advantage of having a small inflammatory response and no cytotoxicity. However, due to the macrophage and polymorpho-nuclear leukocyte-derived enzymatic activities, the degradation of the barrier membrane starts soon after implantation. The rapid degradation of the barrier membrane results in the inability to achieve its desired barrier effect, which ultimately leads to surgical failure (Mir-Mari et al., 2017; Meyer, 2019). Several studies have shown that membranes’ integrity was well maintained during the first 14 days. However, there is a significant reduction in membrane thickness from 14 to 30 days of healing and a significant reduction in the total amount of collagen. Nevertheless, bone formation was occurring incorporating fragments of the degraded collagen fibers at 30 days (Moses et al., 2008; Kozlovsky et al., 2009). It has been suggested that a 1-month barrier function time for each millimeter of bone regeneration is needed (Guarnieri et al., 2015). Depending on the size of the periodontal bone defects and the graft materials, the time required for bone regeneration and healing varies greatly. Generally, bone grafts in the mouth heal after 3–9 months, whereas larger alveolar bone defects require longer to regenerate and heal (Triplett and Schow, 1996; Laurencin et al., 2006). Ideally, the membranes’ biodegradation rate should match the new tissue formation rate with no residual materials left (Sbricoli et al., 2020). The regeneration rate of bone tissue is much slower than that of connective tissue in periodontal bone defects. Hence, the barrier membranes’ degradation rate should match the bone healing rate. In order to provide mechanical support during bone formation, the integrity and stability of membranes should be guaranteed in the range from 4 weeks, when the bone remodeling phase starts, up to several months (Sánchez-Fernández et al., 2021). The degradation time of natural resorbable membranes depends on the tissue origin and manufacture process. For example, Collatape, made of bovine collagen, presents a barrier effect of 1–2 weeks, whereas Botiss Jason, made of the porcine pericardium, and Copios Extend, made of the porcine dermis, present a barrier effect of 8–12 and 24–36 weeks, respectively (Sbricoli et al., 2020). To improve the degradation resistance and prolong the barrier effect of CM and other natural resorbable membranes, researchers have proposed various methods, such as cross-linking or larger and thicker membranes (Jiménez Garcia et al., 2017). Multiple studies have cross-linked existing collagen fibers based on physical (UV irradiation), chemical (glutaraldehyde, hexamethylene diisocyanate, diphenyl phosphoryl azide), and enzymatic (ribose) interactions produce resorbable cross-linked CM effectively improving CM persistence (Petite et al., 1994; Olde Damink et al., 1995; Weadock et al., 1995; Behring et al., 2008; Aprile et al., 2020). Moses et al. (2008) reported that the improvement of the persistence of cross-linked CM may be due to its resistance to enzymatic degradation during bone regeneration, and the persistence of CM is prolonged with the increase of cross-linking ratio. However, the enzyme activity in the tissue may increase under pathological conditions, affecting the regeneration of bonePLA, PCL tissue in the defect area and leading to accelerated CM degradation (Eliezer et al., 2022). Several studies have shown that compared with normal rats, the inflammatory infiltration in CM of diabetic rats is more obvious, and the number of macrophages and endothelial cells is significantly increased (Eliezer et al., 2013; Moses et al., 2016). These cells also can secrete the matrix metalloproteinase. While the activity of these proteinases likely contributes to both the host defence function of macrophages and normal tissue remodeling and repair (Campbell et al., 1991), various reports have shown that it is also the main reason for the accelerated degradation of CM (Moses et al., 2016). Eliezer et al. (2022) showed that compared with non-immersed CM, the degradation of CM could be delayed, which is immersed in cross-linked high molecular weight hyaluronic acid (CLHA) for type 1 diabetic rats. Hyaluronic acid is a natural glycosaminoglycan. It is an essential component of connective tissues and plays an important role during wound healing (Croce et al., 2001). CLHA can reduce the production of inflammatory cytokines such as interleukin (IL)-1β, showing anti-inflammatory effect (Mitsui et al., 2008). The reason for this is likely linked to a phenotypic switch macrophages undergo when they are in contact with Hyaluronic acid. M1 macrophages mainly play a role in pro-inflammatory and immune clearance by secreting pro-inflammatory factors, thereby killing pathogens and tumor cells. M2 macrophages are mainly involved in anti-inflammatory and wound healing by secreting anti-inflammatory and growth factors. Promoting phenotypic polarization of M2 macrophages can effectively promote angiogenesis and bone healing. Macrophage phenotypes depend on the molecular weight of HA. At LMW of HA, macrophages have pro-inflammatory response and at HMW of HA, macrophages have anti-inflammatory and pro-resolving responses. Therefore, the immersion of CM in CLHA before implantation may slow down the degradation of CM by reducing the number of infiltrating macrophages, thus prolonging the maintenance time of CM thickness and collagen density, then better preserving the barrier function of CM (Eliezer et al., 2022). In addition to reducing the release of enzymes directly by reducing the number of inflammatory factors such as macrophages by anti-inflammatory substances, reducing the susceptibility of native membranes to enzymatic degradation by macrophage-released lysozyme can also slow the rate of membrane degradation. Murali et al. (2021) prepared modified electrospun CM including acetic anhydride (AA) and hexanoic anhydride (HA) using electrospun chitosan membranes (ESCM) modified with short-chain fatty acids. Studies have shown that compared with HA membranes, AA membranes are more hydrophilic and able to absorb more fluid, leading to more cell infiltration and faster membrane degradation. HA membranes have a slower degradation rate which will lead to a better barrier effect. HA membranes are more suitable for the regeneration of large periodontal bone defects. This may be due to the increased hydrophobicity of the membranes with increasing length of short-chain fatty acids, which slows the hydrolysis of HA from the polymer chains thereby reducing their susceptibility to enzymatic degradation by macrophage-released lysozyme (Murali et al., 2021).
In summary, GBR membranes must have a degradation rate that matches bone tissue formation to achieve effective regeneration of periodontal bone defects. It is of great significance to select the appropriate material sources and preparation methods for applying GBR membranes to promote bone regeneration because the degradation rate of natural polymer absorbable membranes is affected by the origins and manufacturing process of the tissue. In addition, the material sources of the natural membrane itself, the natural structure and properties, and the properties of the surrounding environment have an important impact on membrane degradation. It is the most common and effective way to improve the degradation rate of natural polymer membranes by using different degrees of collagen cross-linking to enhance CM’s molecular structure and enzymatic degradation resistance. However, the internal environment of the tissue under pathological conditions causes many inflammatory cells to infiltrate into the implanted GBR membranes, leading to rapid membrane degradation. Studies have shown that improving the hydrophobicity of membranes by anti-inflammatory factor infiltration or short-chain fatty acid molecular modification can effectively reduce the release of inflammatory factors or improve their susceptibility to enzymatic degradation, ultimately achieving the improvement of membrane degradation rate. However, the clinical application of these membranes needs to be further evaluated, such as their potential to induce bone healing in the presence of graft materials or periodontal bone defects of different sizes.
Synthetic absorbable barrier membranes can be made of aliphatic polyesters, e.g.,.poly (glycolic acid) (PGA), poly (lactic acid) (PLA), and their copolymer poly (lactide-coglycolide) (PLGA) (Van et al., 2022). Compared with CMs, studies have shown that synthetic polymers such as PLA have more advantages as membrane structures in terms of early volume changes of bone defect healing. In the middle of healing, a synthetic absorbable barrier membrane can produce an ideal isolation effect due to its slow degradation rate (Won et al., 2016). In the later stage, bone tissue repair may be derived from the physical and chemical properties of the material itself or the degradation products of the synthetic polymer resorbable membranes (Zhang et al., 2020). Studies have shown that the GBR membranes made of PLA or its copolymers have good biocompatibility, high mechanical strength, slow degradation rate, and no risk of animal-derived infection or cross-species immune response. It has a significant osteogenic effect when applied to periodontal bone and mandibular alveolar bone defects (He et al., 2019; Xia et al., 2019; Abdelaziz et al., 2021). In addition, the key reason that synthetic resorbable membranes could appear as an alternative to CMs and synthetic non-resorbable membranes is that their physicochemical properties can be regulated by synthetic materials and the preparation methods for effective control, including the size, shape, porosity, mechanical properties, degradability, depending on the nature of the application and the specific requirements (Zahid et al., 2019; Aprile et al., 2020). However, studies have shown that most synthetic polymers have high hydrophobicity, which leads to poor cell adhesion behaviors and limits bone tissue regeneration (Aprile et al., 2020; Abdelaziz et al., 2021). Although it has been studied that PLGA membranes modified with RGD adhesion peptide can effectively improve cell adhesion, most studies are still devoted to improving membrane hydrophobicity. In addition, studies have shown that the osteogenic activity of synthetic polymer membranes is often lower than that of native membranes, which also limits their clinical application (Tryba et al., 2022). Therefore, improving the hydrophobicity, cell adhesion, and osteogenic activity of synthetic polymer resorbable membranes is the main challenge for clinical development and application.
In contrast to the enzymatic degradation of native membranes, synthetic membranes are often reabsorbed by non-enzymatic (hydrolytic) cleavage to form pyruvate and lactate (Bottino et al., 2012). Thus, although synthetic polymers are biodegradable and non-cytotoxic, their rapid degradation may release acidic by-products and cause localized foreign body reactions, characterized by presence of macrophages and intracellular remnants of the polymer, which may cause an immune response (Liu and Kerns, 2014). However, too slow degradation of the synthetic polymer resorbable membranes is not conducive to realizing the GBR program due to the high hydrophobicity (Miroshnichenko et al., 2019). For example, PLA is more hydrophobic than PGA, and degradation time is much longer. In vivo degradation of PLA lasts more than 4 years, but copolymerization with PGA or PCL reduces resorption time to less than 1 year, which is better for GBR (Gentile et al., 2011). One should not confound resorption time and persistence of barrier effect, which is always at least 2 times shorter. Most membranes provide an effective barrier during at least 6 weeks and up to 24 weeks (Wang et al., 2016). In addition, the hydrophilicity and hydrophobicity of the membrane molecules and the wettability of the membrane surface affect the adsorption of extracellular matrix proteins, deposition of platelets, formation of blood clots, and adhesion and morphology of progenitor cells with osteogenic differentiation potential (Hunter and Ma, 2013; Wang et al., 2016). Higher hydrophobicity limits the interaction between polymer and cells, reducing the adhesion of the GBR membrane surface to osteoblasts and fibroblasts and hindering bone tissue regeneration (Hunter and Ma, 2013). The synthesized polymer membranes should have appropriate hydrophobicity and degradation rates to achieve membrane degradation that matches tissue healing. PCL is a semicrystalline hydrophobic biodegradable polyester with good mechanical and degradation properties and tunable microstructure. It is a widely used GBR membrane preparation material in clinical practice (Wissing et al., 2017). As with most synthetic polymers, the high hydrophobicity of PCL results in poor cell adhesion and other behaviors compared to native extracellular matrices (Croll et al., 2004). In order to improve the hydrophilicity of pure PCL membranes, Tais et al. (Costa Salles et al., 2020) modified the hydrophobic surface of PCL membranes using plant extracts Pterodon pubescens Benth (P. pubescens) and Arrabidaea chica Verlot (A. chica). Fibrous membranes associated with plant extracts P. Pubescens, and A. chica showed hydrophilic properties, higher wettability, and zero water contact angles compared with controls. Water contact angle measurement has been shown to provide information about hydrophilicity and hydrophobicity of the membrane surface. The lower the contact angle, the higher the surface hydrophilicity. When the contact angle was lower than 80, osteoblasts’ and fibroblast adhesion was maximum (Shah et al., 2019). Studies have shown that there are free hydroxyls (OH) in the A. chica extract, which leads to its being highly hydrophilic (Aro et al., 2013). When PCL was combined with P. Pubescens, the release of A. chica was increased, which positively affected the adhesion and proliferation of fibroblasts, proving the potential application value of these modified membranes in guiding the regeneration of periodontal bone tissue (Costa Salles et al., 2020). Not only by using plant extracts, hydrophilicity can also be improved by adding bioactive inorganic particles such as hydroxyapatite (HAp), tricalcium phosphate, and bioactive glass (BGs) (Jo et al., 2009; Zhang et al., 2009). Another fast degradable component with good biocompatibility and hydrophilicity can improve the biodegradability and hydrophobicity of the PCL matrix. However, Several synthetic bioresorbable polymers, such as PLA, PCL, and PLGA, have been used to balance resistance and stiffness. The addition of bioactive inorganic particles to these polymers leads to a significant increase in stiffness, and the resultant composites present high elastic module values (Castro et al., 2018; Zhang et al., 2020). The elastic modulus of Jason membrane is 178.9MPa, and its maximum tensile strain (%) is 17.9. In contrast, the Bio-Gide film has an elastic modulus of 15.7 MPa and a maximum tensile strain (%) of 46.8 (Zitzmann et al., 1997). High elastic module values may impair the handling, making their adaptation in different surgical site configurations difficult and may lead to clinical drawbacks like soft tissue dehiscence (Rakhmatia et al., 2013). Therefore, when combining with other hydrophilic substances to modify synthetic polymers, it is necessary to pay attention to the selection of materials and whether the modification destroys the original physical structure and chemical properties of synthetic materials. Studies have found that the polybutylene adipate terephthalate (PBAT), a biodegradable aliphatic-aromatic polyester composed of adipic acid, 1,4-butanediol, and terephthalic acid, is highly flexible compared with conventional synthetic polymers. PBAT allows the incorporation of inorganic particles into its structure without compromising its handling (Jones, 2015; Kashani Rahimi et al., 2017; Wang and Yeung, 2017). Gabriela et al. (Balbinot et al., 2021) successfully prepared a PBAT/BAGNb composite GBR membrane with tailored surface properties and osteogenic induction activity by adding niobium-containing bioactive glasses (BAGNb) to PBAT. The addition of BAGNb moderately strengthened the membrane’s stiffness, reduced the membrane’s water contact angle, and promoted higher interaction between the membrane, blood, and surrounding cells (Verné et al., 2009). Compared with traditional surface modification strategies, coating surface modification can significantly improve cells’ hydrophobicity and adhesion properties. The solid hydrophobic substrates allowed the silk proteins to form a coating of various thicknesses. Previous studies have successfully used recombinant spider silk proteins to coat hydrophobic polymers for biomedical applications and improved their wetting capacity (Harris et al., 2016; Tasiopoulos et al., 2020). The PTFE is a hydrophobic material described by low surface energy and high contact angle (>110°) to water-based compounds. The water contact angle further increases due to the porosity of extended PTFE and can reach up to 160°. Harsh as well as milder surfactants have also been used to improve the wetting and make PTFE substrates more favorable to protein-based solutions. In order to achieve a complete wetting of the porous PTFE membrane, a liquid that intrinsically has a low contact angle (<90°) on the material has to be utilized and then gradually being replaced by water, without introducing entrapped air. The lowest contact angle toward PTFE is reported to be possessed by nonpolar aprotic liquids. This result is because membranes modified with coatings such as natural polymers can better mimic the physiological conditions of the specific cell niche. However, it remains a significant challenge to precisely mimic the physiological state of the particular cell niche in clinical applications (Ejeian et al., 2020). Therefore, some studies use metal-organic framework materials (MOF), which have a wide range of physical and mechanical properties of MOF materials and structural flexibility, as a viable platform for multifactorial control of cell-substrate interaction. And they have achieved good application results (Ejeian et al., 2020). Fatemeh et al. (Ejeian et al., 2020) used the zeolitic imidazolate framework-8 (ZIF-8) commonly used in MOF to crystallize in situ on the polydopamine (PDA) modified membranes, which significantly improved the wettability of the substrate and increased the primary attachment of human dental pulp stem cells (hDPSCs). Previous studies demonstrated the physical properties of the ZIF-8 compact layer. The thickness of this compact layer is about 800 nm, and it has a significant adsorption capacity for silver particles. The polypropylene (PP) membranes modified by PDA/ZIF-8 showed a significant increase in surface hydrophilicity, which can effectively support the growth and proliferation of hDPSCs. In the same way, Julia et al. (Higuchi et al., 2019) used hydroxyapatite nanoparticles (HANPs) coating to modify poly (D, Polymer blends of Poly (D, L-lactic acid) (PDLLA)/PLGA electrospun membranes can effectively improve their hydrophilicity. Moreover, nHA may prevent the diffusion of intermediate degradation products out of the polymer, slowing down the pH drop (Cao et al., 2012). As a result, the acidic autocatalytic degradation of the polymer is slowed down, so that possible inflammation reactions could be avoided or be less severely present, and promoting the membranes to exhibit satisfactory mechanical properties. This study also compares the two methods:sonocoating and electrospraying of nHA suspensions. The results showed that sonocoating was more effective in improving osteocyte adhesion, structural stability, harmful pH changes, and wetting properties and may prolong membranes degradation time, which may be used in the treatment of periodontal bone defects (Higuchi et al., 2019).
In summary, the hydrophilicity/hydrophobicity of synthetic polymer resorbable membranes is of great significance in their preparation and application. Too hydrophilic or hydrophobic polymers may adversely affect the successful implementation of the GBR process since synthetic polymers need to be degraded by hydrolysis. Due to the high hydrophobicity of most synthetic polymers, which leads to poor cell adhesion and other behaviours, recent studies have paid increasing attention to reducing the hydrophobicity of the prepared membranes. In current studies, the degradation and hydrophobicity of synthetic polymer matrix can be effectively improved by copolymerizing a variety of polymers or adding other hydrophilic components. However, it is necessary to pay attention to avoid affecting the excellent mechanical properties and other chemical properties of the synthetic membrane itself when applied with other substances. In addition, many studies have shown that compared with other traditional methods, surface coating modification is a better way to improve the hydrophobicity of composite membranes. However, various methods to enhance the hydrophobicity of synthetic polymer membranes are still not widely used in practice. Therefore, further exploration and comparison are needed in the future to obtain more acceptable preparation or modification methods.
Ideally, GBR membranes require good mechanical properties in combination with space retention and handling capacity. In addition, biological activities should be combined in GBR membranes to promote cell activity and bone repair (Sbricoli et al., 2020). One of the critical factors for the success of GBR is that the membranes have good bone conductivity and osteoinductive properties. They can effectively promote bone growth while avoiding the migration of ectopic osteogenic epithelial cells. Studies have shown that although bone graft materials covered with PLA membrane can form mature bone similar to CM produced, the lack of bone conductivity of PLA membrane hinders its ability to promote bone regeneration, which is not conducive to the realization of GBR (Hwang et al., 2020). Studies have proved that most synthetic polymer materials do not show great biological activity to promote bone formation and lack adequate handling capacity. Therefore, these materials cannot meet the requirements of wide application in different clinical situations and need to be combined with granular bone grafts to enhance the bone bioactivity and bone repair of synthetic polymer membranes (Shankar et al., 2018). HAp is the main inorganic component of enamel, dentin, and bone. Synthetic HAp is widely used as a component of bone and tooth fillers due to its good biocompatibility, bioactivity, and bone conductivity. Adding HAp and other bioactive inorganic particles into the polymers can induce bone conduction properties and accelerate the healing process (Tsai et al., 2019). These bioactive materials can form an apatite-like layer at the interface in vivo by undergoing specific surface reactions, which leads to developing a strong adherent bond with the host hard/soft tissues. The apatite layer stimulates osteoblasts (bone-forming cells) and promotes new bone formation in situ (Hench, 2006). Some studies have shown that Strontium (Sr) is a divalent cation that can partially substitute Ca2+ in the crystal lattice of HAp. Zhang et al. (Shankar et al., 2018) reported that strontium-substituted hydroxyapatite (SrHAp) has a higher solubility than pure HAp due to a difference in ionic radius, which contributes to perturbations in the crystal lattice. Tsai et al. (2018) introduced SrHAp fiber fragments into PCL for the first time to prepare GBR composite membranes. The results proved that PCL-SrHANF composite membranes could produce higher bioactivity and osteogenic potential than PCL membranes alone. The above results may be due to the that the Sr2+ ions released from the PCL–SrHANF membranes enhance the expression and activity of osteogenesis-related genes and proteins through the interaction of calcium-sensing receptor (CaR) with cells to activate inositol-1,4,5-triphosphate production and mitogen-activated protein kinase signaling, which regulate osteogenic differentiation (Caverzasio, 2008). In addition to SrHAp, some studies have shown that octacalcium phosphate (OCP) can also produce better bone induction performance than HAp. OCP can serve as a inductive factor for initiating bone deposition and directing osteoblast differentiation by releasing inorganic phosphate (Pi) ions when hydrolysed (Ishiko-Uzuka et al., 2017; Sai et al., 2018). Wang et al. (2020) introduced OCP into poly (3-hydroxybutyrateco-4-hydroxybutyrate) (P (3HB-Co-4Hb) to formed P (3HB-Co-4Hb)/OCP nanofiber membrane through electrospinning for GBR, which significantly increased the osteogenic activity of P (3HB-Co-4Hb) membrane. Therefore, the introduction of OCP is expected to improve the osteogenic and bone conductivity of synthetic polymers for medical applications. However, with the development of MOF nanostructures, many recent approaches have utilized MOF nanoparticles for improving osteoconductivity/osteoinductivity of standard bone substituents, such as titanium, poly-l-lactic acid, and calcium phosphate (Ejeian et al., 2020). MOF is a porous structure whose favorable nanostructural properties can minimize the requirement for bioactive inducible molecules and provide an alternative to chemical-based cellular microenvironments (Min et al., 2019; Ejeian et al., 2020). Fatemeh et al. (Ejeian et al., 2020) prepared a PP membrane modified by PDA/ZIF-8 coating, which showed superior osteogenic biological activity and could effectively support the growth and proliferation of DPSCs. In addition, it has been reported that adding alginate, gelatin, growth factors, and other active factors can also improve the bone-inducing activity of synthetic polymer membranes (Fuji et al., 2009; Kurobane et al., 2019).
In summary, the low bone conductivity and osteogenic induction properties of synthetic polymer materials limit synthetic polymer resorbable membranes’ effective potential for realizing GBR programs. Researchers often introduce HAp, SrHAp, OCP, and other bioactive inorganic particles to improve these defects, showing superior application potential to improve osteogenic induction activity. In addition to osteogenic cations such as Sr2+, newly developed MOF nanoparticles and different bioactive compounds such as growth factors can also modify the membrane surface to improve its bioactivity. However, using bioactive inorganic particles widely may change other physical properties of the membranes. It is necessary further to explore the study of MOF and bioactive compounds.
In the resorbable GBR membranes, the natural polymer resorbable membranes have good cell adhesion and biodegradation, but their mechanical strength is low, and the degradation time is short. Synthetic polymer resorbable membranes have good biological compatibility, controllable biodegradability, and machinability. However, the hydrophobicity of synthetic polymer resorbable membranes often limits cell adhesion and proliferation, and their low electrical conductivity of bone leads to insufficient osteogenesis induction activity, leading to poor or slow bone regeneration. To combine the advantages of various polymers and overcome their limitations, blends of these polymers are commonly used in clinical practice rather than using a single polymer. Furthermore, by adding active substances such as bioactive inorganic particles, antibacterial or osteogenic drugs, and growth factors, then optimizing the ratio of components of the blends, customized GBR membranes with great mechanical and biological properties can be obtained. This is the best way to prepare novel ideal GBR membranes (Abdelaziz et al., 2021; Niu et al., 2021). For example, Dina et al. (Abdelaziz et al., 2021) prepared electrospun nanofiber membranes by combining PCL and PLA/cellulose acetate (CA) polymer. Furthermore, silver nanoparticles (AgNPs) and HANPs synthesized in green were added to improve the antibacterial activity, bone conductivity and bone binding ability, respectively, successfully preparing a promising GBR fiber membrane. In addition, there are also studies designing and preparing the bilayer structure of GBR membranes by blending multi-components, showing superior GBR ability. (Figure 1F). Niu et al. (2021) used electrospinning technology to prepare polyamide-6/PA6/CS nanofiber membranes with higher toughness and good mechanical properties. Provides enhanced mechanical properties of the composite membranes; then, they prepared porous HANPs/PA6 membranes by solvent casting and evaporation on the surface of electrospun PA6/CS nanofiber membranes, which have good biocompatibility and bone conductivity, improving the bioactivity of the composite membranes and promoting the regeneration of periodontal bone defects. There are molecular interactions among PA6, HANPs, and CS, which can maintain the connection between the bilayer structures (Niu et al., 2021). Studies have shown that more and more studies have begun to pay attention to the development of bilayer or multilayer asymmetric membranes with graded structure or composition. Such membranes with unique designs can promote bone regeneration while making GBR membrane play a barrier role, showing good GBR effect, and is considered a perfect strategy to promote bone healing in recent years (Yazdani et al., 2018). The novel asymmetric membranes generally consist of two layers with different surface properties. One part of these membranes facing the bone tissue is loose, which has the appropriate pore size and porosity, and the other one that meets the epithelial tissue is dense. The open layer facilitates osseointegration and blood clot stabilization, whereas the thick layer isolates the bone defect from infiltrating fibrous tissue and enables nutrient permeation (Ma et al., 2014). Reza et al. (Moonesi Rad et al., 2019) developed an asymmetric bilayer membrane composed of a smooth layer of non-porous CA and a rough, porous fiber layer composed of CA/Gel, adding 7% boron-modified bioactive glass (7B BG). The gradient bilayer membrane with a good barrier and the ability to promote osteogenic differentiation has been successfully prepared, which has excellent potential to induce regeneration of periodontal bone defects. Studies showed that adding 7B BG improved the surface wettability and biodegradability of the fiber membranes. And hDPSCs exhibited better attachment, diffusion, and proliferation on the bilayer membranes containing 7B BG. In addition to the asymmetric bilayer structure, a novel three-layer functionally graded shell GBR membrane with BG gradients (50%, 25%, and 0% wt) was also prepared by lyophilization (Shah et al., 2019). The intermediate layer is also intended to promote better bone tissue regeneration (after degradation of the lower layer) and contribute to the mechanical properties of the membranes, so it contains a low BG nanoparticle content (25 wt%) to make it flexible during surgical management and maintain mechanical properties. It is worth noting that the development of asymmetric GBR membranes requires strict control of polymer properties, concentrations, solvents, and reaction conditions to regulate membrane porosity and surface area to obtain ideal GBR membranes with physical and biological properties (Zahid et al., 2019). In addition, selecting suitable solvents or materials that can form molecular chemical bonds between each other to form a firm adhesion between the two layers and avoid the separation of the two membranes has an essential impact on the success of GBR.
In summary, blending natural and synthetic polymers can combine the advantages of natural and synthetic materials, overcome their application defects, and obtain an ideal GBR membrane with superior cell adhesion, biocompatibility, mechanical properties, and degradation properties. However, more and more studies believe that the GBR membranes with the dual role of barrier and promoting bone regeneration is ideal and efficient, so developing novel asymmetric GBR membranes has attracted more and more attention. Asymmetric bilayer or multilayer membranes mainly include dense and loose layers. The dense layer mainly plays a barrier role, and the loose layer can effectively promote osteogenic differentiation. In addition, the combination of asymmetric membranes and bioactive inorganic particles or other substances can achieve a better GBR effect. However, although many asymmetric bilayers show sound osteogenic effects in vitro, their structures have not been fully defined in vivo. In addition, specific experiments on animal models of alveolar bone defects are still lacking. Future studies should further test the application effect of the novel composite/blended GBR membranes in good vivo alveolar bone defect models. (Tables 1, 2).
At present, the newly designed membranes can often be used as a carrier or combined with drugs, active molecules, and other substances in some ways in the clinical application of GBR membranes to obtain good activity in promoting bone, vascular tissue regeneration, and antibacterial activity, finally achieving the recovery of periodontal bone defects.
Studies have shown that an ideal barrier membrane design should incorporate the function of a delivery vehicle for transporting drugs and osteoinductive factors to where the body is under inflammation. It can actively induce osteogenic differentiation or inhibit osteoclast resorption while playing a barrier role, promoting bone defect tissue regeneration (Zhang et al., 2017). For example, Lin and Chiu (2021) used calcium-type poly-γ glutamic acid (γ-PGA) to blend with glycerol to form a new barrier membrane, which has excellent swelling performance and can carry many drugs needed for bone repair (Li and Mooney, 2016). In addition, the use of GBR to deliver drugs can achieve local specific drug delivery and avoid repeatedly used over a long period of global drugs cause many side effects such as the development of bacterial resistance and local inadequate drug concentrations in the periodontal tissue and gingival crevicular fluid, showing a broad application prospect (Lavie et al., 2017; Magierowski et al., 2018). Generally, directly combined drugs into the GBR membranes lead to the fast release of the drugs from the membranes, subsequent in a high burst release and short release period. It often results in high local drug concentrations that inhibit osteogenesis (Slots and Ting, 2002). Therefore, achieving a controlled and slow release of drugs from GBR membranes is an important challenge (Ghavimi et al., 2020). Studies are developing sustained and controlled drug delivery systems (DDS) for GBR applications through nanotechnology. For example, with targeted and controlled release, polymer-based and lipid-based nanoparticles can reduce drug delivery time and avoid toxicity to other organs (Li et al., 2015; Wang et al., 2017). Natural polymers have become the best materials for preparing nanoparticles to avoid drug-related complications due to their biodegradable properties (Kumari et al., 2010). Zhang et al. (2017) prepared an asymmetric membrane as the aspirin local drug delivery system through cross-linked collagen containing aspirin-loaded chitosan nanoparticles (ACS) as the loose layer and chitosan, which degraded slowly as the dense layer. The results showed that ACS had an ideal controlled-release mode and significantly promoted the formation of new bone when the membranes were applied in rat cranial defect models. In addition to developing a controlled drug delivery system to promote bone regeneration, the selection of drugs delivered by the GBR membrane is also of great significance for promoting the regeneration of periodontal bone defects (Ghavimi et al., 2020). Many studies showed that some non-steroidal anti-inflammatory drugs (NSAIDs) widely used in bone tissue repair have the potential to promote osteogenic differentiation. NSAIDs can control the balance between bone formation and resorption, preventing osteoclast differentiation and maturation, then accelerating bone repair (Tang et al., 2014; Lee et al., 2016). In addition, NSAIDs can prevent tissue invasion of bone defects during GBR surgery (Zhang et al., 2017). Studies have shown that NSAIDs can reduce the severity of tissue destruction and bone loss caused by periodontal disease (Shiloah et al., 2014). However, the dose of NSAIDs is a key factor in cell proliferation, differentiation, and migration, and excessive NSAIDs may adversely affect osteoblast proliferation (Pountos et al., 2012). In addition to delivering drugs with the potential to promote osteogenic differentiation through membranes, studies have also explored the application effect of drugs with the ability to inhibit osteoclast resorption from promoting bone regeneration (Küçüktürkmen et al., 2021). Berrin et al. (Küçüktürkmen et al., 2021) developed a membrane containing Zoledronic Acid (ZA) and the in situ gel formulation containing ZA-loaded nanoparticles, which showed that the simultaneous application of both could produce better osteogenic effects. ZA is often used in drug-induced osteoporosis and bone loss and shows its key effect by inhibiting the function of osteoclast cells that conduct bone resorption. Recently, several studies have shown that membranes can effectively promote bone tissue regeneration by delivering drugs and promote osteogenesis by delivering regenerative factors sustainably. The researchers evaluated the in vitro efficacy of a collagen membrane containing recombinant platelet derived growth factor-BB (PDGF-BB) in pre-osteoblastic (MC3T3-E1) cells. A sustained release pattern of the growth factor for up to 3 weeks was observed that significantly increased the alkaline phosphatase activity and expression of key osteogenic genes such as RUNX2 in MC3T3-E1 cells (Yamano et al., 2014). However, although the delivery of growth factors by GBR membranes is effective in promoting osteogenic differentiation, there are many defects such as the inherent instability of proteins in the tissue milieu, conformational changes, degradation and the lack of an optimal delivery system that releases these factors for an adequate period of time limiting their application (Ikada, 2006). To remedy these deficiencies, Khorsand et al. (2019) attempted to convey the plasmid DNA (pDNA) encoding bone morphogenetic protein-9 (BMP-9) or chemically modified RNA (cmRNA) by membranes, rather than its form of protein. The results showed that PCM-pDNA (BMP-9) and PCM-cmRNA (BMP-9) nanocomplexes could significantly promote osteogenic differentiation in vitro and in vivo, which proved that the use of GBR membranes to deliver protein factor encoding DNA or RNA could overcome the limitations of protein delivery methods and has a strong application potential.
In summary, GBR membranes can be carriers for local-specific drug delivery. And the precise and controlled release of local drug molecules can be effectively achieved by developing GBR membranes containing namic DDS. In clinical application, GBR membranes carrying appropriate concentrations of drugs with the potential to promote osteogenic differentiation or inhibit osteoclast resorption can effectively promote osteogenic differentiation and accelerate bone reconstruction. In addition, using GBR membranes to deliver regenerative factors and their coding proteins DNA or RNA can also effectively stimulate the expression of osteogenic genes and accelerate bone regeneration, which has a strong application potential. It is necessary to compare the limitations and practicability of GBR membranes to achieve more efficient bone tissue regeneration and promote the healing of periodontal bone defects.
Angiogenesis is a required step for new bone formation, and the pore size of the GBR membranes is the key to facilitating angiogenesis (Liu and Kerns, 2014). The pores of 25–50 μm allow invasion of cells and vessels on the external surface, while larger pore sizes (50–100 and 100–150 μm) allow mature vascularized tissue formation through the material structure (Chiu et al., 2011). On the contrary, pore sizes of the barrier membranes should be small and occlusive to prevent the penetration of gingival tissue cells into the defect space and gain nutrients and blood supply from the host bone. The membrane consists of micropores (under 100 μm) that might occlude the cells and inhibit the penetration of fibroblasts and soft tissue from the mucoperiosteal flap into the defect during the bone regeneration period, promoting the GBR membrane to play its role (Song et al., 2020). In the semi-rigid barrier system, both shell and covering membranes are less than 40 μm, sufficient to perform barrier functions and suitable for angiogenesis and vascular penetration into the defect area. In comparison, the high-density PTFE (d-PTFE) has tiny porosity of 0.2 μm and causes avascular tissue underneath (Lee et al., 2018). Therefore, unlike the promotion of bone regeneration, the angiogenesis of periodontal bone defects by GBR membranes does not depend on the delivery of drugs, active molecules, and other substances but on the porous structure of the membrane itself and other physical properties. Xue et al. (2021) developed an electrospun asymmetric bilayer PCL/Col membrane modified with MOF crystals, which had a significant ability to promote angiogenesis. The high porosity of ZIF-8 crystals in the GBR asymmetric membrane developed in this study ensures adequate gas and nutrient exchange for angiogenesis (Simon-Yarza et al., 2018). In addition, MOF crystals can provide pH-responsive to store Zn2+ ions as a pool under neutral physiological conditions and release them under acidic conditions, stimulating and regulating stem cell proliferation and osteogenic differentiation and promoting endothelial cell angiogenesis in vitro. In another study, silica mesoporous nanoparticles were added to the fiber PLGA membrane. Increasing the polymer concentration yielded fibers with better dispersion of the silica-based mesoporous nanocarriers, combining increased porosity and large fiber diameter, promoting angiogenesis (Pouroutzidou et al., 2022). More attention has been paid to modifying GBR membranes to improve their function of blocking and osteogenesis. However, promoting angiogenesis by GBR membranes is often used for intensive treatment in pathological conditions. For example, tissue regeneration in patients with hyperglycemia’s periodontal bone defect area is obviously limited due to insufficient blood vessel formation and vascular network lesions (Liu et al., 2022). Some studies have developed a CaP/Gel bilayer asymmetric membrane to promote the expression of HIF-1α through calcium release and create a microenvironment for angiogenesis, which can significantly promote microvascular regeneration and new bone formation in the diabetic rat cranial critical defect model and overcome the limitations of bone tissue regeneration in the pathological environment (Njegic et al., 2021; Liu et al., 2022). It also shows the potential of the inorganic mineral-modified membrane to promote angiogenesis in the treatment of periodontal bone defects. In addition, due to the excellent properties of boosting the angiogenesis of inorganic bioactive substances such as BGs, adding them to modify GBR membranes to promote angiogenesis in periodontal bone defects also has good application prospects (Wu et al., 2011; Moonesi Rad et al., 2019).
In summary, angiogenesis is a crucial step in healing periodontal bone defects. Angiogenesis mainly depends on the pore size of the GBR membrane, and appropriate porosity can effectively promote angiogenesis. In recent studies, modified GBR membranes with MOF can significantly promote angiogenesis, which may be due to the influence of MOF crystal layer structure. Therefore, unlike bone regeneration, the ability of the GBR membranes to promote vascular regeneration mainly depends on the design of the GBR membrane itself. In addition, since chronic diseases such as diabetes often require intensive treatment to promote bone regeneration, enhancing the ability of GBR membranes to promote angiogenesis becomes particularly important in this pathological setting.
In recent years, researchers have paid more attention to the antibacterial properties of GBR membranes in treating periodontal bone defects. The reasons may include two aspects: 1) periodontitis is the most important factor causing periodontal bone defects, while the bacterial infection is the leading cause of periodontitis (Abdelaziz et al., 2021). 2) During treatment with GBR membranes, the environment provided by the mouth is highly exposed to infection, which can lead to the failure of the GBR procedures (Lian et al., 2019; Porrelli et al., 2021). Therefore, treating periodontal bone defects with antimicrobial therapy is necessary while inducing bone tissue regeneration. Antibiotics and other drugs are often added to the membranes to develop GBR membranes with antimicrobial activity through the delivery function of GBR membranes in many studies at present. These drugs can be dissolved in polymer solutions, grafted onto nanofibers, or loaded into nanoparticles for antimicrobial treatment (Xue et al., 2015; He et al., 2017; Shi et al., 2019). For example, Georgia et al. (Pouroutzidou et al., 2022) prepared a composite membrane by adding Moxifloxacin (MOX) loaded mesoporous nanocarriers to PLGA. MOX is the fourth generation of fluoroquinolone antibiotics and provides excellent antibacterial activity against a wide variety of putative periodontal pathogens, such as Porphyromonas gingivalis, Tannerella forsythia, Peptostreptococcus spp., etc. (Landersdorfer et al., 2009; Flemmig et al., 2011). The research shows that the composite membrane has a good application prospect because it can be used for extended controlled drug release (Pouroutzidou et al., 2022). Although antibiotics are the standard procedure for antimicrobial therapy, the application challenges of antibiotics delivery by GBR membranes continue to arise due to the development of multidrug-resistant bacteria (Liu et al., 2017; Lian et al., 2019). Therefore, broad-spectrum antimicrobial compounds and strategies have been developed to meet these challenges in recent years. Davide et al. (Porrelli et al., 2021) successfully obtained bioactive and antibacterial GBR membranes using lactose-modified chitosan and AgNPs to functionalize PCL electrospun membranes. AgNPs can interfere with cell membrane permeability and transport and even kill bacterial cells by interacting with molecules such as DNA and proteins that contain sulfur and phosphorus. AgNPs interfere with the cell membrane permeability and transport functions of the bacterial cells. They can also kill bacterial cells by interacting with sulfur and phosphorus-containing molecules such as DNA and proteins (Sambhy et al., 2006). Studies have shown that AgNPs are not toxic to the cells; on the other hand, they exert antibacterial activity without causing the development of resistant bacterial strains. In addition, AgNPs emerged as a valid strategy as they can be easily prepared and included in biomaterials. These conclusions suggest that AgNPs are a compelling new strategy for preparing GBR membranes with antimicrobial activity (Lansdown, 2010; Liu et al., 2017). In addition to improving the antimicrobial activity of GBR membranes by replacing antibiotics with compounds that do not produce resistant strains, studies have developed controlled “supply on demand” drug release systems to avoid the development of antimicrobial resistance caused by the overuse of antibiotics. For example, Sunil et al. (Boda et al., 2020) used chitosan, a naturally derived mucin-adhering PH-responsive polysaccharide, as the primary material of the membranes to prepare the GBR membranes for PH-controlled oral antimicrobial peptide delivery. It shows good antibacterial properties and application potential.
In summary, the antibacterial properties of GBR membranes were increasingly developed while improving their abilities to promote bone regeneration, which is vital for repairing periodontal bone defects in recent years. Although the GBR membrane can be used as a vehicle to deliver antibiotics and other drugs to achieve antimicrobial treatment, it has certain limitations due to the development of multidrug-resistant bacteria. Therefore, novel antimicrobial strategies need to be developed to overcome this defect. Several studies have shown that functionalizing GBR membranes with AgNPs that do not produce resistant strains is an effective and straightforward method. In addition, delivery of antimicrobial peptides and improvement of drug release using PH response to avoid antibiotic abuse are also valid strategies to improve the antimicrobial properties of GBR membranes.
GBR is a standard method for the maintenance and repair of periodontal bone defects. This technique mainly relies on the application of GBR membranes to protect the bone defects and prevents the growth and proliferation of epithelial cells and connective tissue cells into the defects to damage the adequate new bone formation. The commonly used GBR membranes include degradable and non-degradable resorption, among which the degradable resorption membranes have become the first choice for the clinical application of GBR because they do not require a second surgical removal. Among the degradable membranes, using natural polymer resorbable membranes is often limited by the disadvantages of low mechanical strength and short degradation time. In contrast, synthetic polymer resorbable membranes are often limited by poor cell adhesion due to the high hydrophobicity and poor biological activities such as low bone conductivity. Therefore, in addition to studying various methods to improve the defects of the above two kinds of membranes, more attention is paid to preparing new composite membranes by blending the two types of membranes. The new membrane can combine the advantages of natural and synthetic membranes to achieve practical barrier function and accelerate bone reconstruction function. In addition, applying the GBR membrane as a carrier of drugs, active molecules, and other substances has become a research hotspot in regenerating periodontal bone defects. The application of GBR membranes in combination with various drugs, growth factors, and bioactive inorganic particles to promote bone regeneration, angiogenesis, and effective antibacterial properties is the central aspect of clinical application at present, and it varies according to the type of periodontal bone defects and environments (Figure 2).
FIGURE 2. The schematic of membranes and bone defect compartments showing potential stragies of improving the performances GBR membranes.
However, there are still many shortcomings in current studies, such as: 1) model questions: the choice of animal species, the size of the periodontal bone defect, and the time of implantation can also influence the effects of the barrier membrane in vivo. Bone defect models in rats and dogs are the most commonly used in the study of GBR membrane application. Because they are small and easy to operate. However, the main drawback of these animals is the low similarity to human bones, which can lead to limited relevance of studies. The selection of an animal model should be based on similarity to the intended clinical application. Therefore, future in vivo studies will have to consider more relevant large animal models of bone defects before clinical translation. Further studies are needed to better understand the exact mechanisms and adverse effects of GBR membrane application. 2) Selection criteria: GBR membranes for the treatment of periodontal bone defects have been extensively developed. Various types of membranes have different advantages and characteristics. The size, severity and etiology of periodontal bone defects vary greatly in clinical treatment. Due to these differences of membranes and bone defects, the clinical application of GBR membranes is also different. And most materials cannot meet the requirements of wide application in different clinical situations. Therefore, it is necessary to establish a more comprehensive evaluation and application plan. Further clinical tests will be conducted to generate the best recommended criteria for membrane application to ensure the safe and effective implementation of GBR technology. 3) Clinical application: most studies focus on the development and improvement of membranes with different properties, but less attention has been paid to the specific efficacy of membranes in clinical application. Therefore, more and more extensive research is needed to translate the potential clinical application of GBR membrane into its practical application. With the rapid development of artificial intelligence and other technologies in recent years, nano-dentistry is trying its best to apply new signs of progress in tissue engineering and dental practice in the feld of periodontal therapies. With the growth of advanced investigations and deeper understanding of electrospinning sets-ups, it is probable to attain future “smart bone healing devices” proficient in treating all features of bone defects for real clinical uses. In conclusion, future research is still needed to explore and solve related problems. In the context of the rapid development of science and technology, more in-depth research should be conducted to achieve more effective, safer and cheaper periodontal bone defect regeneration treatment. Therefore, the study of GBR membranes is a potentially hot issue in the future.
Conceptualization, JG and JW; Writing–original draft preparation, DW and XZ; Writing–review and editing, HC; Funding acquisition, HZ and DW; Supervision, JG and JW. All authors contributed to the article and approved the submitted version.
This work was supported by National Natural Science Foundation of China (No. 91731900046 to HZ; No.81972085 and No.82172465 to DW) and Dean’s Research Fund of Southern University of Science and Technology Hospital (No:2022-D6 to JW).
The authors declare that the research was conducted in the absence of any commercial or financial relationships that could be construed as a potential conflict of interest.
All claims expressed in this article are solely those of the authors and do not necessarily represent those of their affiliated organizations, or those of the publisher, the editors and the reviewers. Any product that may be evaluated in this article, or claim that may be made by its manufacturer, is not guaranteed or endorsed by the publisher.
Abdelaziz, D., Hefnawy, A., Al-Wakeel, E., El-Fallal, A., and El-Sherbiny, I. M. (2021). New biodegradable nanoparticles-in-nanofibers based membranes for guided periodontal tissue and bone regeneration with enhanced antibacterial activity. J. Adv. Res. 28, 51–62. doi:10.1016/j.jare.2020.06.014
Abe, G. L., Sasaki, J. I., Katata, C., Kohno, T., Tsuboi, R., Kitagawa, H., et al. (2020). Fabrication of novel poly(lactic acid/caprolactone) bilayer membrane for GBR application. Dent. Mater 36 (5), 626–634. doi:10.1016/j.dental.2020.03.013
Ahn, J. J., Kim, H. J., Bae, E. B., Cho, W. T., Choi, Y., Hwang, S. H., et al. (2020). Evaluation of 1-ethyl-3-(3-dimethylaminopropyl) carbodiimide cross-linked collagen membranes for guided bone regeneration in beagle dogs. Mater. (Basel) 13 (20), 4599. doi:10.3390/ma13204599
Almeida, A. L. G., Freitas, G. P., Lopes, H. B., Gimenes, R., Siessere, S., Sousa, L. G., et al. (2019). Effect of stem cells combined with a polymer/ceramic membrane on osteoporotic bone repair. Braz Oral Res. 33, e079. doi:10.1590/1807-3107BOR-2019.vol33.0079
Amano, Y., Ota, M., Sekiguchi, K., Shibukawa, Y., and Yamada, S. (2004). Evaluation of a poly-l-lactic acid membrane and membrane fixing pin for guided tissue regeneration on bone defects in dogs. Oral Surg. Oral Med. Oral Pathol. Oral Radiol. Endod. 97 (2), 155–163. doi:10.1016/j.tripleo.2003.09.009
An, Y. Z., Strauss, F. J., Park, J. Y., Shen, Y. Q., Thoma, D. S., and Lee, J. S. (2022). Membrane fixation enhances guided bone regeneration in standardized calvarial defects: a pre-clinical study. J. Clin. Periodontol. 49 (2), 177–187. doi:10.1111/jcpe.13583
Ang, S. L., Shaharuddin, B., Chuah, J. A., and Sudesh, K. (2020). Electrospun poly(3-hydroxybutyrate-co-3-hydroxyhexanoate)/silk fibroin film is a promising scaffold for bone tissue engineering. Int. J. Biol. Macromol. 145, 173–188. doi:10.1016/j.ijbiomac.2019.12.149
Aprile, P., Letourneur, D., and Simon-Yarza, T. (2020). Membranes for guided bone regeneration: a road from bench to bedside. Adv. Healthc. Mater 9 (19), e2000707. doi:10.1002/adhm.202000707
Aro, A. A., Freitas, K. M., Foglio, M. A., Carvalho, J. E., Dolder, H., Gomes, L., et al. (2013). Effect of the Arrabidaea chica extract on collagen fiber organization during healing of partially transected tendon. Life Sci. 92 (13), 799–807. doi:10.1016/j.lfs.2013.02.011
Balbinot, G. S., Bahlis, E., Visioli, F., Leitune, V. C. B., Soares, R. M. D., and Collares, F. M. (2021). Polybutylene-adipate-terephthalate and niobium-containing bioactive glasses composites: development of barrier membranes with adjusted properties for guided bone regeneration. Mater Sci. Eng. C Mater Biol. Appl. 125, 112115. doi:10.1016/j.msec.2021.112115
Behring, J., Junker, R., Walboomers, X. F., Chessnut, B., and Jansen, J. A. (2008). Toward guided tissue and bone regeneration: morphology, attachment, proliferation, and migration of cells cultured on collagen barrier membranes. A systematic review. Odontology 96 (1), 1–11. doi:10.1007/s10266-008-0087-y
Benic, G. I., and Hämmerle, C. H. (2014). Horizontal bone augmentation by means of guided bone regeneration. Periodontol 66 (1), 13–40. doi:10.1111/prd.12039
Boda, S. K., Fischer, N. G., Ye, Z., and Aparicio, C. (2020). Dual oral tissue adhesive nanofiber membranes for pH-responsive delivery of antimicrobial peptides. Biomacromolecules 21 (12), 4945–4961. doi:10.1021/acs.biomac.0c01163
Boerckel, J. D., Uhrig, B. A., Willett, N. J., Huebsch, N., and Guldberg, R. E. (2011). Mechanical regulation of vascular growth and tissue regeneration in vivo. Proc. Natl. Acad. Sci. U. S. A. 108 (37), E674–E680. doi:10.1073/pnas.1107019108
Bottino, M. C., Thomas, V., Schmidt, G., Vohra, Y. K., Chu, T. M., Kowolik, M. J., et al. (2012). Recent advances in the development of GTR/GBR membranes for periodontal regeneration--a materials perspective. Dent. Mater 28 (7), 703–721. doi:10.1016/j.dental.2012.04.022
Bunyaratavej, P., and Wang, H. L. (2001). Collagen membranes: a review. J. Periodontol. 72 (2), 215–229. doi:10.1902/jop.2001.72.2.215
Caballé-Serrano, J., Sawada, K., Miron, R. J., Bosshardt, D. D., Buser, D., and Gruber, R. (2017). Collagen barrier membranes adsorb growth factors liberated from autogenous bone chips. Clin. Oral Implants Res. 28 (2), 236–241. doi:10.1111/clr.12789
Calciolari, E., Ravanetti, F., Strange, A., Mardas, N., Bozec, L., Cacchioli, A., et al. (2018). Degradation pattern of a porcine collagen membrane in an in vivo model of guided bone regeneration. J. Periodontal Res. 53 (3), 430–439. doi:10.1111/jre.12530
Campbell, E. J., Cury, J. D., Shapiro, S. D., Goldberg, G. I., and Welgus, H. G. (1991). Neutral proteinases of human mononuclear phagocytes. Cellular differentiation markedly alters cell phenotype for serine proteinases, metalloproteinases, and tissue inhibitor of metalloproteinases. J. Immunol. 146 (4), 1286–1293. doi:10.4049/jimmunol.146.4.1286
Cao, L., Duan, P. G., Wang, H. R., Li, X. L., Yuan, F. L., Fan, Z. Y., et al. (2012). Degradation and osteogenic potential of a novel poly(lactic acid)/nano-sized β-tricalcium phosphate scaffold. Int. J. Nanomedicine 7, 5881–5888. doi:10.2147/ijn.S38127
Castro, A. G. B., Diba, M., Kersten, M., Jansen, J. A., van den Beucken, J., and Yang, F. (2018). Development of a PCL-silica nanoparticles composite membrane for guided bone regeneration. Mater Sci. Eng. C Mater Biol. Appl. 85, 154–161. doi:10.1016/j.msec.2017.12.023
Caverzasio, J. (2008). Strontium ranelate promotes osteoblastic cell replication through at least two different mechanisms. Bone 42 (6), 1131–1136. doi:10.1016/j.bone.2008.02.010
Chen, Y., Chen, M., Gao, Y., Zhang, F., Jin, M., Lu, S., et al. (2022). Biological efficacy comparison of natural tussah silk and mulberry silk nanofiber membranes for guided bone regeneration. ACS Omega 7 (23), 19979–19987. doi:10.1021/acsomega.2c01784
Chiapasco, M., and Casentini, P. (2018). Horizontal bone-augmentation procedures in implant dentistry: prosthetically guided regeneration. Periodontol. 2000 77 (1), 213–240. doi:10.1111/prd.12219
Chiu, Y. C., Cheng, M. H., Engel, H., Kao, S. W., Larson, J. C., Gupta, S., et al. (2011). The role of pore size on vascularization and tissue remodeling in PEG hydrogels. Biomaterials 32 (26), 6045–6051. doi:10.1016/j.biomaterials.2011.04.066
Chu, C., Deng, J., Sun, X., Qu, Y., and Man, Y. (2017). Collagen membrane and immune response in guided bone regeneration: recent progress and perspectives. Tissue Eng. Part B Rev. 23 (5), 421–435. doi:10.1089/ten.TEB.2016.0463
Costa Salles, T. H., Volpe-Zanutto, F., de Oliveira Sousa, I. M., Machado, D., Zanatta, A. C., Vilegas, W., et al. (2020). Electrospun PCL-based nanofibers Arrabidaea chica Verlot - Pterodon pubescens Benth loaded: synergic effect in fibroblast formation. Biomed. Mater 15 (6), 065001. doi:10.1088/1748-605X/ab9bb1
Croce, M. A., Dyne, K., Boraldi, F., Quaglino, D., Cetta, G., Tiozzo, R., et al. (2001). Hyaluronan affects protein and collagen synthesis by in vitro human skin fibroblasts. Tissue Cell 33 (4), 326–331. doi:10.1054/tice.2001.0180
Croll, T. I., O'Connor, A. J., Stevens, G. W., and Cooper-White, J. J. (2004). Controllable surface modification of poly(lactic-co-glycolic acid) (PLGA) by hydrolysis or aminolysis I: physical, chemical, and theoretical aspects. Biomacromolecules 5 (2), 463–473. doi:10.1021/bm0343040
Dimitriou, R., Mataliotakis, G. I., Calori, G. M., and Giannoudis, P. V. (2012). The role of barrier membranes for guided bone regeneration and restoration of large bone defects: current experimental and clinical evidence. BMC Med. 10, 81. doi:10.1186/1741-7015-10-81
Di Raimondo, R., Sanz-Esporrín, J., Sanz-Martin, I., Plá, R., Luengo, F., Vignoletti, F., et al. (2021). Hard and soft tissue changes after guided bone regeneration using two different barrier membranes: an experimental in vivo investigation. Clin. Oral Investig. 25 (4), 2213–2227. doi:10.1007/s00784-020-03537-5
Donos, N., Kostopoulos, L., and Karring, T. (2002). Alveolar ridge augmentation using a resorbable copolymer membrane and autogenous bone grafts. An experimental study in the rat. Clin. Oral Implants Res. 13 (2), 203–213. doi:10.1034/j.1600-0501.2002.130211.x
Ducheyne, P., De Meester, P., Aernoudt, E., Martens, M., and Mulier, J. C. (1977). Influence of a functional dynamic loading on bone ingrowth into surface pores of orthopedic implants. J. Biomed. Mater Res. 11 (6), 811–838. doi:10.1002/jbm.820110603
Ejeian, F., Razmjou, A., Nasr-Esfahani, M. H., Mohammad, M., Karamali, F., Ebrahimi Warkiani, M., et al. (2020). ZIF-8 modified polypropylene membrane: a biomimetic cell culture platform with a view to the improvement of guided bone regeneration. Int. J. Nanomedicine 15, 10029–10043. doi:10.2147/ijn.S269169
Eliezer, M., Nemcovsky, C., Romanos, G., Kozlovsky, A., Tal, H., Kolerman, R., et al. (2013). Opposing effects of diabetes and tetracycline on the degradation of collagen membranes in rats. J. Periodontol. 84 (4), 529–534. doi:10.1902/jop.2012.120188
Eliezer, M., Sculean, A., Miron, R. J., Nemcovsky, C., Bosshardt, D. D., Fujioka-Kobayashi, M., et al. (2022). Cross-linked hyaluronic acid slows down collagen membrane resorption in diabetic rats through reducing the number of macrophages. Clin. Oral Investig. 26 (3), 2401–2411. doi:10.1007/s00784-021-04206-x
Flemmig, T. F., Petersilka, G., Völp, A., Gravemeier, M., Zilly, M., Mross, D., et al. (2011). Efficacy and safety of adjunctive local moxifloxacin delivery in the treatment of periodontitis. J. Periodontol. 82 (1), 96–105. doi:10.1902/jop.2010.100124
Fuji, T., Anada, T., Honda, Y., Shiwaku, Y., Koike, H., Kamakura, S., et al. (2009). Octacalcium phosphate-precipitated alginate scaffold for bone regeneration. Tissue Eng. Part A 15 (11), 3525–3535. doi:10.1089/ten.TEA.2009.0048
Gao, X., Al-Baadani, M. A., Wu, M., Tong, N., Shen, X., Ding, X., et al. (2022). Study on the local anti-osteoporosis effect of polaprezinc-loaded antioxidant electrospun membrane. Int. J. Nanomedicine 17, 17–29. doi:10.2147/ijn.S341216
Gentile, P., Chiono, V., Tonda-Turo, C., Ferreira, A. M., and Ciardelli, G. (2011). Polymeric membranes for guided bone regeneration. Biotechnol. J. 6 (10), 1187–1197. doi:10.1002/biot.201100294
Ghavimi, M. A., Bani Shahabadi, A., Jarolmasjed, S., Memar, M. Y., Maleki Dizaj, S., and Sharifi, S. (2020). Nanofibrous asymmetric collagen/curcumin membrane containing aspirin-loaded PLGA nanoparticles for guided bone regeneration. Sci. Rep. 10 (1), 18200. doi:10.1038/s41598-020-75454-2
Gottlow, J. (1993). Guided tissue regeneration using bioresorbable and non-resorbable devices: initial healing and long-term results. J. Periodontol. 64 (11 Suppl. l), 1157–1165. doi:10.1902/jop.1993.64.11s.1157
Graziani, F., Karapetsa, D., Alonso, B., and Herrera, D. (2017). Nonsurgical and surgical treatment of periodontitis: how many options for one disease? Periodontol. 2000 75 (1), 152–188. doi:10.1111/prd.12201
Gruber, R., Stadlinger, B., and Terheyden, H. (2017). Cell-to-cell communication in guided bone regeneration: molecular and cellular mechanisms. Clin. Oral Implants Res. 28 (9), 1139–1146. doi:10.1111/clr.12929
Guarnieri, R., DeVilliers, P., and Belleggia, F. (2015). GBR using cross-linked collagen membrane and a new highly purified bovine xenograft (Laddec) in horizontal ridge augmentation: case report of clinical and histomorphometric analysis. Quintessence Int. 46 (8), 717–724. doi:10.3290/j.qi.a34178
Guo, H., Xia, D., Zheng, Y., Zhu, Y., Liu, Y., and Zhou, Y. (2020). A pure zinc membrane with degradability and osteogenesis promotion for guided bone regeneration: in vitro and in vivo studies. Acta Biomater. 106, 396–409. doi:10.1016/j.actbio.2020.02.024
Gutta, R., Baker, R. A., Bartolucci, A. A., and Louis, P. J. (2009). Barrier membranes used for ridge augmentation: is there an optimal pore size? J. Oral Maxillofac. Surg. 67 (6), 1218–1225. doi:10.1016/j.joms.2008.11.022
Harris, T. I., Gaztambide, D. A., Day, B. A., Brock, C. L., Ruben, A. L., Jones, J. A., et al. (2016). Sticky situation: an investigation of robust aqueous-based recombinant spider silk protein coatings and adhesives. Biomacromolecules 17 (11), 3761–3772. doi:10.1021/acs.biomac.6b01267
He, H., Lu, Y., Qi, J., Zhu, Q., Chen, Z., and Wu, W. (2019). Adapting liposomes for oral drug delivery. Acta Pharm. Sin. B 9 (1), 36–48. doi:10.1016/j.apsb.2018.06.005
He, M., Jiang, H., Wang, R., Xie, Y., and Zhao, C. (2017). Fabrication of metronidazole loaded poly (ε-caprolactone)/zein core/shell nanofiber membranes via coaxial electrospinning for guided tissue regeneration. J. Colloid Interface Sci. 490, 270–278. doi:10.1016/j.jcis.2016.11.062
Hench, L. L. (2006). The story of Bioglass. J. Mater Sci. Mater Med. 17 (11), 967–978. doi:10.1007/s10856-006-0432-z
Higuchi, J., Fortunato, G., Woźniak, B., Chodara, A., Domaschke, S., Męczyńska-Wielgosz, S., et al. (2019). Polymer membranes sonocoated and electrosprayed with nano-hydroxyapatite for periodontal tissues regeneration. Nanomater. (Basel) 9 (11), 1625. doi:10.3390/nano9111625
Hoornaert, A., d'Arros, C., Heymann, M. F., and Layrolle, P. (2016). Biocompatibility, resorption and biofunctionality of a new synthetic biodegradable membrane for guided bone regeneration. Biomed. Mater 11 (4), 045012. doi:10.1088/1748-6041/11/4/045012
Hunter, K. T., and Ma, T. (2013). In vitro evaluation of hydroxyapatite-chitosan-gelatin composite membrane in guided tissue regeneration. J. Biomed. Mater Res. A 101 (4), 1016–1025. doi:10.1002/jbm.a.34396
Hwang, C., Park, S., Kang, I. G., Kim, H. E., and Han, C. M. (2020). Tantalum-coated polylactic acid fibrous membranes for guided bone regeneration. Mater Sci. Eng. C Mater Biol. Appl. 115, 111112. doi:10.1016/j.msec.2020.111112
Ikada, Y. (2006). Challenges in tissue engineering. J. R. Soc. Interface 3 (10), 589–601. doi:10.1098/rsif.2006.0124
Ishiko-Uzuka, R., Anada, T., Kobayashi, K., Kawai, T., Tanuma, Y., Sasaki, K., et al. (2017). Oriented bone regenerative capacity of octacalcium phosphate/gelatin composites obtained through two-step crystal preparation method. J. Biomed. Mater Res. B Appl. Biomater. 105 (5), 1029–1039. doi:10.1002/jbm.b.33640
Jabbari, F., Hesaraki, S., and Houshmand, B. (2019). The physical, mechanical, and biological properties of silk fibroin/chitosan/reduced graphene oxide composite membranes for guided bone regeneration. J. Biomater. Sci. Polym. Ed. 30 (18), 1779–1802. doi:10.1080/09205063.2019.1666235
Jensen, A., Ladegaard Grønkjær, L., Holmstrup, P., Vilstrup, H., and Kilian, M. (2018). Unique subgingival microbiota associated with periodontitis in cirrhosis patients. Sci. Rep. 8 (1), 10718. doi:10.1038/s41598-018-28905-w
Jian, B., Wu, W., Song, Y., Tan, N., and Ma, C. (2019). Microporous elastomeric membranes fabricated with polyglycerol sebacate improved guided bone regeneration in a rabbit model. Int. J. Nanomedicine 14, 2683–2692. doi:10.2147/ijn.S192167
Jiménez Garcia, J., Berghezan, S., Caramês, J. M. M., Dard, M. M., and Marques, D. N. S. (2017). Effect of cross-linked vs non-cross-linked collagen membranes on bone: a systematic review. J. Periodontal Res. 52 (6), 955–964. doi:10.1111/jre.12470
Jo, J. H., Lee, E. J., Shin, D. S., Kim, H. E., Kim, H. W., Koh, Y. H., et al. (2009). In vitro/in vivo biocompatibility and mechanical properties of bioactive glass nanofiber and poly(ε-caprolactone) composite materials. J. Biomed. Mater Res. B Appl. Biomater. 91 (1), 213–220. doi:10.1002/jbm.b.31392
Jones, J. R. (2015). Reprint of: review of bioactive glass: from Hench to hybrids. Acta Biomater. 23 (Suppl. l), S53–S82. doi:10.1016/j.actbio.2015.07.019
Kashani Rahimi, S., Aeinehvand, R., Kim, K., and Otaigbe, J. U. (2017). Structure and biocompatibility of bioabsorbable nanocomposites of aliphatic-aromatic copolyester and cellulose nanocrystals. Biomacromolecules 18 (7), 2179–2194. doi:10.1021/acs.biomac.7b00578
Kavarthapu, A., and Gurumoorthy, K. (2021). Linking chronic periodontitis and oral cancer: a review. Oral Oncol. 121, 105375. doi:10.1016/j.oraloncology.2021.105375
Keten, S., Xu, Z., Ihle, B., and Buehler, M. J. (2010). Nanoconfinement controls stiffness, strength and mechanical toughness of beta-sheet crystals in silk. Nat. Mater 9 (4), 359–367. doi:10.1038/nmat2704
Khorsand, B., Elangovan, S., Hong, L., Kormann, M. S. D., and Salem, A. K. (2019). A bioactive collagen membrane that enhances bone regeneration. J. Biomed. Mater Res. B Appl. Biomater. 107 (6), 1824–1832. doi:10.1002/jbm.b.34275
Kocher, T., König, J., Borgnakke, W. S., Pink, C., and Meisel, P. (2018). Periodontal complications of hyperglycemia/diabetes mellitus: epidemiologic complexity and clinical challenge. Periodontol. 2000 78 (1), 59–97. doi:10.1111/prd.12235
Kozlovsky, A., Aboodi, G., Moses, O., Tal, H., Artzi, Z., Weinreb, M., et al. (2009). Bio-degradation of a resorbable collagen membrane (Bio-Gide) applied in a double-layer technique in rats. Clin. Oral Implants Res. 20 (10), 1116–1123. doi:10.1111/j.1600-0501.2009.01740.x
Küçüktürkmen, B., Öz, U. C., Toptaş, M., Devrim, B., Saka, O. M., Bilgili, H., et al. (2021). Development of zoledronic acid containing biomaterials for enhanced guided bone regeneration. J. Pharm. Sci. 110 (9), 3200–3207. doi:10.1016/j.xphs.2021.05.002
Kumari, A., Yadav, S. K., and Yadav, S. C. (2010). Biodegradable polymeric nanoparticles based drug delivery systems. Colloids Surf. B Biointerfaces 75 (1), 1–18. doi:10.1016/j.colsurfb.2009.09.001
Kurobane, T., Shiwaku, Y., Anada, T., Hamai, R., Tsuchiya, K., Baba, K., et al. (2019). Angiogenesis involvement by octacalcium phosphate-gelatin composite-driven bone regeneration in rat calvaria critical-sized defect. Acta Biomater. 88, 514–526. doi:10.1016/j.actbio.2019.02.021
Lacroix, D., and Prendergast, P. J. (2002). A mechano-regulation model for tissue differentiation during fracture healing: analysis of gap size and loading. J. Biomech. 35 (9), 1163–1171. doi:10.1016/s0021-9290(02)00086-6
Landersdorfer, C. B., Kinzig, M., Hennig, F. F., Bulitta, J. B., Holzgrabe, U., Drusano, G. L., et al. (2009). Penetration of moxifloxacin into bone evaluated by Monte Carlo simulation. Antimicrob. Agents Chemother. 53 (5), 2074–2081. doi:10.1128/aac.01056-08
Lansdown, A. B. (2010). A pharmacological and toxicological profile of silver as an antimicrobial agent in medical devices. Adv. Pharmacol. Sci. 2010, 1–16. doi:10.1155/2010/910686
Laurencin, C., Khan, Y., and El-Amin, S. F. (2006). Bone graft substitutes. Expert Rev. Med. Devices 3 (1), 49–57. doi:10.1586/17434440.3.1.49
Lavie, C. J., Howden, C. W., Scheiman, J., and Tursi, J. (2017). Upper gastrointestinal toxicity associated with long-term aspirin therapy: consequences and prevention. Curr. Probl. Cardiol. 42 (5), 146–164. doi:10.1016/j.cpcardiol.2017.01.006
Lee, B. S., Lee, C. C., Wang, Y. P., Chen, H. J., Lai, C. H., Hsieh, W. L., et al. (2016). Controlled-release of tetracycline and lovastatin by poly(D,L-lactide-co-glycolide acid)-chitosan nanoparticles enhances periodontal regeneration in dogs. Int. J. Nanomedicine 11, 285–297. doi:10.2147/ijn.S94270
Lee, S. Y., Wu, S. C., Chen, H., Tsai, L. L., Tzeng, J. J., Lin, C. H., et al. (2018). Synthesis and characterization of polycaprolactone-based polyurethanes for the fabrication of elastic guided bone regeneration membrane. Biomed. Res. Int. 2018, 3240571–3240613. doi:10.1155/2018/3240571
Li, J., and Mooney, D. J. (2016). Designing hydrogels for controlled drug delivery. Nat. Rev. Mater 1 (12), 16071. doi:10.1038/natrevmats.2016.71
Li, L., Yang, H., Li, X., Yan, S., Xu, A., You, R., et al. (2021). Natural silk nanofibrils as reinforcements for the preparation of chitosan-based bionanocomposites. Carbohydr. Polym. 253, 117214. doi:10.1016/j.carbpol.2020.117214
Li, W., Ding, Y., Yu, S., Yao, Q., and Boccaccini, A. R. (2015). Multifunctional chitosan-45S5 bioactive glass-poly(3-hydroxybutyrate-co-3-hydroxyvalerate) microsphere composite membranes for guided tissue/bone regeneration. ACS Appl. Mater Interfaces 7 (37), 20845–20854. doi:10.1021/acsami.5b06128
Lian, M., Sun, B., Qiao, Z., Zhao, K., Zhou, X., Zhang, Q., et al. (2019). Bi-layered electrospun nanofibrous membrane with osteogenic and antibacterial properties for guided bone regeneration. Colloids Surf. B Biointerfaces 176, 219–229. doi:10.1016/j.colsurfb.2018.12.071
Lin, C. C., and Chiu, J. Y. (2021). A novel γ-PGA composite gellan membrane containing glycerol for guided bone regeneration. Mater Sci. Eng. C Mater Biol. Appl. 118, 111404. doi:10.1016/j.msec.2020.111404
Liu, J., and Kerns, D. G. (2014). Mechanisms of guided bone regeneration: a review. Open Dent. J. 8, 56–65. doi:10.2174/1874210601408010056
Liu, J., Zou, Q., Cai, B., Wei, J., Yuan, C., and Li, Y. (2020). Heparin conjugated PCL/Gel - PCL/Gel/n-HA bilayer fibrous membrane for potential regeneration of soft and hard tissues. J. Biomater. Sci. Polym. Ed. 31 (11), 1421–1436. doi:10.1080/09205063.2020.1760700
Liu, M., Luo, G., Wang, Y., He, W., Liu, T., Zhou, D., et al. (2017). Optimization and integration of nanosilver on polycaprolactone nanofibrous mesh for bacterial inhibition and wound healing in vitro and in vivo. Int. J. Nanomedicine 12, 6827–6840. doi:10.2147/ijn.S140648
Liu, Y., Wang, J., Jiang, M., Li, X., Zhang, Q., and He, H. (2022). Osteoinductive hybrid hydrogel membranes for in situ bone regeneration in hyperglycemia. Colloids Surf. B Biointerfaces 214, 112450. doi:10.1016/j.colsurfb.2022.112450
Luo, D., Yao, C., Zhang, R., Zhao, R., Iqbal, M. Z., Mushtaq, A., et al. (2021). Silk fibroin/collagen blended membrane fabricated via a green papermaking method for potential guided bone regeneration application: in vitro and in vivo evaluation. ACS Biomater. Sci. Eng. 7 (12), 5788–5797. doi:10.1021/acsbiomaterials.1c01060
Luo, J., Yang, J., Zheng, X., Ke, X., Chen, Y., Tan, H., et al. (2020). A highly stretchable, real-time self-healable hydrogel adhesive matrix for tissue patches and flexible electronics. Adv. Healthc. Mater 9 (4), e1901423. doi:10.1002/adhm.201901423
Ma, S., Chen, Z., Qiao, F., Sun, Y., Yang, X., Deng, X., et al. (2014). Guided bone regeneration with tripolyphosphate cross-linked asymmetric chitosan membrane. J. Dent. 42 (12), 1603–1612. doi:10.1016/j.jdent.2014.08.015
Magierowski, M., Hubalewska-Mazgaj, M., Magierowska, K., Wojcik, D., Sliwowski, Z., Kwiecien, S., et al. (2018). Nitric oxide, afferent sensory nerves, and antioxidative enzymes in the mechanism of protection mediated by tricarbonyldichlororuthenium(II) dimer and sodium hydrosulfide against aspirin-induced gastric damage. J. Gastroenterol. 53 (1), 52–63. doi:10.1007/s00535-017-1323-4
Mano, J. F., Silva, G. A., Azevedo, H. S., Malafaya, P. B., Sousa, R. A., Silva, S. S., et al. (2007). Natural origin biodegradable systems in tissue engineering and regenerative medicine: present status and some moving trends. J. R. Soc. Interface 4 (17), 999–1030. doi:10.1098/rsif.2007.0220
Masoudi Rad, M., Nouri Khorasani, S., Ghasemi-Mobarakeh, L., Prabhakaran, M. P., Foroughi, M. R., Kharaziha, M., et al. (2017). Fabrication and characterization of two-layered nanofibrous membrane for guided bone and tissue regeneration application. Mater Sci. Eng. C Mater Biol. Appl. 80, 75–87. doi:10.1016/j.msec.2017.05.125
Meinig, R. P. (2010). Clinical use of resorbable polymeric membranes in the treatment of bone defects. Orthop. Clin. North Am. 41 (1), 39–47. doi:10.1016/j.ocl.2009.07.012
Melke, J., Midha, S., Ghosh, S., Ito, K., and Hofmann, S. (2016). Silk fibroin as biomaterial for bone tissue engineering. Acta Biomater. 31, 1–16. doi:10.1016/j.actbio.2015.09.005
Mellonig, J. T., Nevins, M., and Sanchez, R. (1998). Evaluation of a bioabsorbable physical barrier for guided bone regeneration. Part II. Material and a bone replacement graft. Int. J. Periodontics Restor. Dent. 18 (2), 129–137.
Meyer, M. (2019). Processing of collagen based biomaterials and the resulting materials properties. Biomed. Eng. Online 18 (1), 24. doi:10.1186/s12938-019-0647-0
Min, H., Wang, J., Qi, Y., Zhang, Y., Han, X., Xu, Y., et al. (2019). Biomimetic metal-organic framework nanoparticles for cooperative combination of antiangiogenesis and photodynamic therapy for enhanced efficacy. Adv. Mater 31 (15), e1808200. doi:10.1002/adma.201808200
Mir-Mari, J., Benic, G. I., Valmaseda-Castellón, E., Hämmerle, C. H. F., and Jung, R. E. (2017). Influence of wound closure on the volume stability of particulate and non-particulate GBR materials: an in vitro cone-beam computed tomographic examination. Part II. Clin. Oral Implants Res. 28 (6), 631–639. doi:10.1111/clr.12845
Miroshnichenko, S., Timofeeva, V., Permykova, E., Ershov, S., Kiryukhantsev-Korneev, P., Dvořaková, E., et al. (2019). Plasma-Coated polycaprolactone nanofibers with covalently bonded platelet-rich plasma enhance adhesion and growth of human fibroblasts. Nanomater. (Basel) 9 (4), 637. doi:10.3390/nano9040637
Mitsui, Y., Gotoh, M., Nakama, K., Yamada, T., Higuchi, F., and Nagata, K. (2008). Hyaluronic acid inhibits mRNA expression of proinflammatory cytokines and cyclooxygenase-2/prostaglandin E(2) production via CD44 in interleukin-1-stimulated subacromial synovial fibroblasts from patients with rotator cuff disease. J. Orthop. Res. 26 (7), 1032–1037. doi:10.1002/jor.20558
Moonesi Rad, R., Atila, D., Evis, Z., Keskin, D., and Tezcaner, A. (2019). Development of a novel functionally graded membrane containing boron-modified bioactive glass nanoparticles for guided bone regeneration. J. Tissue Eng. Regen. Med. 13 (8), 1331–1345. doi:10.1002/term.2877
Moses, O., Eliezer, M., Nemcovsky, C., Tal, H., and Weinreb, M. (2016). Accelerated degradation of collagen membranes in diabetic rats is associated with increased infiltration of macrophages and blood vessels. Clin. Oral Investig. 20 (7), 1589–1596. doi:10.1007/s00784-015-1635-9
Moses, O., Vitrial, D., Aboodi, G., Sculean, A., Tal, H., Kozlovsky, A., et al. (2008). Biodegradation of three different collagen membranes in the rat calvarium: a comparative study. J. Periodontol. 79 (5), 905–911. doi:10.1902/jop.2008.070361
Murali, V. P., Guerra, F. D., Ghadri, N., Christian, J. M., Stein, S. H., Jennings, J. A., et al. (2021). Simvastatin loaded chitosan guided bone regeneration membranes stimulate bone healing. J. Periodontal Res. 56 (5), 877–884. doi:10.1111/jre.12883
Neto, A. M. D., Sartoretto, S. C., Duarte, I. M., Resende, R. F. B., Neves Novellino Alves, A. T., Mourão, C., et al. (2020). In vivo comparative evaluation of biocompatibility and biodegradation of bovine and porcine collagen membranes. Membr. (Basel) 10 (12), 423. doi:10.3390/membranes10120423
Niu, X., Wang, L., Xu, M., Qin, M., Zhao, L., Wei, Y., et al. (2021). Electrospun polyamide-6/chitosan nanofibers reinforced nano-hydroxyapatite/polyamide-6 composite bilayered membranes for guided bone regeneration. Carbohydr. Polym. 260, 117769. doi:10.1016/j.carbpol.2021.117769
Njegic, A., Swiderska, A., Marris, C., Armesilla, A. L., and Cartwright, E. J. (2021). Plasma membrane calcium ATPase 1 regulates human umbilical vein endothelial cell angiogenesis and viability. J. Mol. Cell Cardiol. 156, 79–81. doi:10.1016/j.yjmcc.2021.03.011
Nyman, S., Lindhe, J., Karring, T., and Rylander, H. (1982). New attachment following surgical treatment of human periodontal disease. J. Clin. Periodontol. 9 (4), 290–296. doi:10.1111/j.1600-051x.1982.tb02095.x
Olde Damink, L. H., Dijkstra, P. J., Van Luyn, M. J., Van Wachem, P. B., Nieuwenhuis, P., and Feijen, J. (1995). Changes in the mechanical properties of dermal sheep collagen during in vitro degradation. J. Biomed. Mater Res. 29 (2), 139–147. doi:10.1002/jbm.820290202
Oliveira, E. A., Dalla-Costa, K. L., França, F. M., Kantovitz, K. R., and Peruzzo, D. C. (2022). Influence of melatonin associated with the Bio-Gide® membrane on osteoblast activity: an in vitro study. Acta Odontol. Latinoam. 35 (2), 90–97. doi:10.54589/aol.35/2/90
Ortolani, E., Quadrini, F., Bellisario, D., Santo, L., Polimeni, A., and Santarsiero, A. (2015). Mechanical qualification of collagen membranes used in dentistry. Ann. Ist. Super. Sanita 51 (3), 229–235. doi:10.4415/ann_15_03_11
Petite, H., Frei, V., Huc, A., and Herbage, D. (1994). Use of diphenylphosphorylazide for cross-linking collagen-based biomaterials. J. Biomed. Mater Res. 28 (2), 159–165. doi:10.1002/jbm.820280204
Pişkin, E. (1995). Biodegradable polymers as biomaterials. J. Biomater. Sci. Polym. Ed. 6 (9), 775–795. doi:10.1163/156856295x00175
Porrelli, D., Mardirossian, M., Musciacchio, L., Pacor, M., Berton, F., Crosera, M., et al. (2021). Antibacterial electrospun polycaprolactone membranes coated with polysaccharides and silver nanoparticles for guided bone and tissue regeneration. ACS Appl. Mater Interfaces 13 (15), 17255–17267. doi:10.1021/acsami.1c01016
Pountos, I., Georgouli, T., Calori, G. M., and Giannoudis, P. V. (2012). Do nonsteroidal anti-inflammatory drugs affect bone healing? A critical analysis. ScientificWorldJournal 2012, 1–14. doi:10.1100/2012/606404
Pouroutzidou, G. K., Lazaridou, M., Papoulia, C., Tsamesidis, I., Chrissafis, K., Vourlias, G., et al. (2022). Electrospun PLGA membranes with incorporated moxifloxacin-loaded silica-based mesoporous nanocarriers for periodontal regeneration. Nanomater. (Basel) 12 (5), 850. doi:10.3390/nano12050850
Raina, D. B., Qayoom, I., Larsson, D., Zheng, M. H., Kumar, A., Isaksson, H., et al. (2019). Guided tissue engineering for healing of cancellous and cortical bone using a combination of biomaterial based scaffolding and local bone active molecule delivery. Biomaterials 188, 38–49. doi:10.1016/j.biomaterials.2018.10.004
Rakhmatia, Y. D., Ayukawa, Y., Furuhashi, A., and Koyano, K. (2013). Current barrier membranes: titanium mesh and other membranes for guided bone regeneration in dental applications. J. Prosthodont Res. 57 (1), 3–14. doi:10.1016/j.jpor.2012.12.001
Rowe, M. J., Kamocki, K., Pankajakshan, D., Li, D., Bruzzaniti, A., Thomas, V., et al. (2016). Dimensionally stable and bioactive membrane for guided bone regeneration: an in vitro study. J. Biomed. Mater Res. B Appl. Biomater. 104 (3), 594–605. doi:10.1002/jbm.b.33430
Sai, Y., Shiwaku, Y., Anada, T., Tsuchiya, K., Takahashi, T., and Suzuki, O. (2018). Capacity of octacalcium phosphate to promote osteoblastic differentiation toward osteocytes in vitro. Acta Biomater. 69, 362–371. doi:10.1016/j.actbio.2018.01.026
Sambhy, V., MacBride, M. M., Peterson, B. R., and Sen, A. (2006). Silver bromide nanoparticle/polymer composites: dual action tunable antimicrobial materials. J. Am. Chem. Soc. 128 (30), 9798–9808. doi:10.1021/ja061442z
Sánchez-Fernández, M. J., Peerlings, M., Félix Lanao, R. P., Bender, J., van Hest, J. C. M., and Leeuwenburgh, S. C. G. (2021). Bone-adhesive barrier membranes based on alendronate-functionalized poly(2-oxazoline)s. J. Mater Chem. B 9 (29), 5848–5860. doi:10.1039/d1tb00502b
Sanz, M., Dahlin, C., Apatzidou, D., Artzi, Z., Bozic, D., Calciolari, E., et al. (2019). Biomaterials and regenerative technologies used in bone regeneration in the craniomaxillofacial region: consensus report of group 2 of the 15th European Workshop on Periodontology on Bone Regeneration. J. Clin. Periodontol. 46 (Suppl. 21), 82–91. doi:10.1111/jcpe.13123
Sbricoli, L., Guazzo, R., Annunziata, M., Gobbato, L., Bressan, E., and Nastri, L. (2020). Selection of collagen membranes for bone regeneration: a literature review. Mater. (Basel) 13 (3), 786. doi:10.3390/ma13030786
Schlegel, A. K., Möhler, H., Busch, F., and andMehl, A. (1997). Preclinical and clinical studies of a collagen membrane(Bio-Gide). Biomaterials 18 (7), 535–538. doi:10.1016/s0142-9612(96)00175-5
Schwarz, F., Rothamel, D., Herten, M., Sager, M., and Becker, J. (2006). Angiogenesis pattern of native and cross-linked collagen membranes: an immunohistochemical study in the rat. Clin. Oral Implants Res. 17 (4), 403–409. doi:10.1111/j.1600-0501.2005.01225.x
Schwarz, F., Rothamel, D., Herten, M., Wüstefeld, M., Sager, M., Ferrari, D., et al. (2008). Immunohistochemical characterization of guided bone regeneration at a dehiscence-type defect using different barrier membranes: an experimental study in dogs. Clin. Oral Implants Res. 19 (4), 402–415. doi:10.1111/j.1600-0501.2007.01486.x
Shah, A. T., Zahid, S., Ikram, F., Maqbool, M., Chaudhry, A. A., Rahim, M. I., et al. (2019). Tri-layered functionally graded membrane for potential application in periodontal regeneration. Mater Sci. Eng. C Mater Biol. Appl. 103, 109812. doi:10.1016/j.msec.2019.109812
Shahdad, S., Gamble, E., Matani, J., Zhang, L., and Gambôa, A. (2020). Randomized clinical trial comparing PEG-based synthetic to porcine-derived collagen membrane in the preservation of alveolar bone following tooth extraction in anterior maxilla. Clin. Oral Implants Res. 31 (10), 1010–1024. doi:10.1111/clr.13648
Shankar, S., Wang, L. F., and Rhim, J. W. (2018). Incorporation of zinc oxide nanoparticles improved the mechanical, water vapor barrier, UV-light barrier, and antibacterial properties of PLA-based nanocomposite films. Mater Sci. Eng. C Mater Biol. Appl. 93, 289–298. doi:10.1016/j.msec.2018.08.002
Sheikh, Z., Khan, A. S., Roohpour, N., Glogauer, M., and Rehman, I. U. (2016). Protein adsorption capability on polyurethane and modified-polyurethane membrane for periodontal guided tissue regeneration applications. Mater Sci. Eng. C Mater Biol. Appl. 68, 267–275. doi:10.1016/j.msec.2016.05.026
Shi, R., Ye, J., Li, W., Zhang, J., Li, J., Wu, C., et al. (2019). Infection-responsive electrospun nanofiber mat for antibacterial guided tissue regeneration membrane. Mater Sci. Eng. C Mater Biol. Appl. 100, 523–534. doi:10.1016/j.msec.2019.03.039
Shiloah, J., Bland, P. S., Scarbecz, M., Patters, M. R., Stein, S. H., and Tipton, D. A. (2014). The effect of long-term aspirin intake on the outcome of non-surgical periodontal therapy in smokers: a double-blind, randomized pilot study. J. Periodontal Res. 49 (1), 102–109. doi:10.1111/jre.12085
Simon-Yarza, T., Mielcarek, A., Couvreur, P., and Serre, C. (2018). Nanoparticles of metal-organic frameworks: on the road to in vivo efficacy in biomedicine. Adv. Mater 30 (37), e1707365. doi:10.1002/adma.201707365
Slots, J., and Ting, M. (2002). Systemic antibiotics in the treatment of periodontal disease. Periodontol. 2000 28, 106–176. doi:10.1034/j.1600-0757.2002.280106.x
Song, J. C., Suwanprateeb, J., Sae-Lee, D., Sosakul, T., Kositbowornchai, S., Klanrit, P., et al. (2020). Clinical and histological evaluations of alveolar ridge augmentation using a novel bi-layered porous polyethylene barrier membrane. J. Oral Sci. 62 (3), 308–313. doi:10.2334/josnusd.19-0218
Sowmya, S., Bumgardener, J. D., Chennazhi, K. P., Nair, S. V., and Jayakumar, R. (2013). Role of nanostructured biopolymers and bioceramics in enamel, dentin and periodontal tissue regeneration. Prog. Polym. Sci. 38 (10-11), 1748–1772. doi:10.1016/j.progpolymsci.2013.05.005
Speer, D. P., Chvapil, M., Eskelson, C. D., and Ulreich, J. (1980). Biological effects of residual glutaraldehyde in glutaraldehyde-tanned collagen biomaterials. J. Biomed. Mater Res. 14 (6), 753–764. doi:10.1002/jbm.820140607
Stetzer, K., Cooper, G., Gassner, R., Kapucu, R., Mundell, R., and Mooney, M. P. (2002). Effects of fixation type and guided tissue regeneration on maxillary osteotomy healing in rabbits. J. Oral Maxillofac. Surg. 60 (4), 427–436. discussion 436-427. doi:10.1053/joms.2002.31232
Sun, T., Liu, M., Yao, S., Ji, Y., Shi, L., Tang, K., et al. (2018). Guided osteoporotic bone regeneration with composite scaffolds of mineralized ECM/heparin membrane loaded with BMP2-related peptide. Int. J. Nanomedicine 13, 791–804. doi:10.2147/ijn.S152698
Sun, X., Xu, C., Wu, G., Ye, Q., and Wang, C. (2017). Poly(Lactic-co-Glycolic acid): applications and future prospects for periodontal tissue regeneration. Polym. (Basel) 9 (6), 189. doi:10.3390/polym9060189
Takata, T., Wang, H. L., and Miyauchi, M. (2001). Migration of osteoblastic cells on various guided bone regeneration membranes. Clin. Oral Implants Res. 12 (4), 332–338. doi:10.1034/j.1600-0501.2001.012004332.x
Tang, J., Xiong, J., Wu, T., Tang, Z., Ding, G., Zhang, C., et al. (2014). Aspirin treatment improved mesenchymal stem cell immunomodulatory properties via the 15d-PGJ2/PPARγ/TGF-β1 pathway. Stem Cells Dev. 23 (17), 2093–2103. doi:10.1089/scd.2014.0081
Tasiopoulos, C. P., Petronis, S., Sahlin, H., and Hedhammar, M. (2020). Surface functionalization of PTFE membranes intended for guided bone regeneration using recombinant spider silk. ACS Appl. Bio Mater 3 (1), 577–583. doi:10.1021/acsabm.9b00972
Tejeda-Montes, E., Klymov, A., Nejadnik, M. R., Alonso, M., Rodriguez-Cabello, J. C., Walboomers, X. F., et al. (2014). Mineralization and bone regeneration using a bioactive elastin-like recombinamer membrane. Biomaterials 35 (29), 8339–8347. doi:10.1016/j.biomaterials.2014.05.095
Thieu, M. K. L., Haugen, H. J., Sanz-Esporrin, J., Sanz, M., Lyngstadaas, S. P., and Verket, A. (2021). Guided bone regeneration of chronic non-contained bone defects using a volume stable porous block TiO2 scaffold: an experimental in vivo study. Clin. Oral Implants Res. 32 (3), 369–381. doi:10.1111/clr.13708
Triplett, R. G., and Schow, S. R. (1996). Autologous bone grafts and endosseous implants: complementary techniques. J. Oral Maxillofac. Surg. 54 (4), 486–494. doi:10.1016/s0278-2391(96)90126-3
Tryba, A. M., Krok-Borkowicz, M., Kula, M., Piergies, N., Marzec, M., Wegener, E., et al. (2022). Surface functionalization of poly(l-lactide-co-glycolide) membranes with RGD-grafted poly(2-oxazoline) for periodontal tissue engineering. J. Funct. Biomater. 13 (1), 4. doi:10.3390/jfb13010004
Tsai, S. W., Yu, W. X., Hwang, P. A., Hsu, Y. W., and Hsu, F. Y. (2019). Fabrication and characteristics of PCL membranes containing strontium-substituted hydroxyapatite nanofibers for guided bone regeneration. Polym. (Basel) 11 (11), 1761. doi:10.3390/polym11111761
Tsai, S. W., Yu, W. X., Hwang, P. A., Huang, S. S., Lin, H. M., Hsu, Y. W., et al. (2018). Fabrication and characterization of strontium-substituted hydroxyapatite-CaO-CaCO3 nanofibers with a mesoporous structure as drug delivery carriers. Pharmaceutics 10 (4), 179. doi:10.3390/pharmaceutics10040179
Turri, A., Elgali, I., Vazirisani, F., Johansson, A., Emanuelsson, L., Dahlin, C., et al. (2016). Guided bone regeneration is promoted by the molecular events in the membrane compartment. Biomaterials 84, 167–183. doi:10.1016/j.biomaterials.2016.01.034
Ul Hassan, S., Bilal, B., Nazir, M. S., Naqvi, S. A. R., Ali, Z., Nadeem, S., et al. (2021). Recent progress in materials development and biological properties of GTR membranes for periodontal regeneration. Chem. Biol. Drug Des. 98 (6), 1007–1024. doi:10.1111/cbdd.13959
Van, T. T. T., Makkar, P., Farwa, U., and Lee, B. T. (2022). Development of a novel polycaprolactone based composite membrane for periodontal regeneration using spin coating technique. J. Biomater. Sci. Polym. Ed. 33 (6), 783–800. doi:10.1080/09205063.2021.2020414
Vernino, A. R., Jones, F. L., Holt, R. A., Nordquist, R. E., and Brand, J. W. (1995). Evaluation of the potential of a polylactic acid barrier for correction of periodontal defects in baboons: a clinical and histologic study. Int. J. Periodontics Restor. Dent. 15 (1), 84–101.
Verné, E., Bretcanu, O., Balagna, C., Bianchi, C. L., Cannas, M., Gatti, S., et al. (2009). Early stage reactivity and in vitro behavior of silica-based bioactive glasses and glass-ceramics. J. Mater Sci. Mater Med. 20 (1), 75–87. doi:10.1007/s10856-008-3537-8
Villar, C. C., and Cochran, D. L. (2010). Regeneration of periodontal tissues: guided tissue regeneration. Dent. Clin. North Am. 54 (1), 73–92. doi:10.1016/j.cden.2009.08.011
Wang, J., Wang, L., Zhou, Z., Lai, H., Xu, P., Liao, L., et al. (2016). Biodegradable polymer membranes applied in guided bone/tissue regeneration: a review. Polym. (Basel) 8 (4), 115. doi:10.3390/polym8040115
Wang, W., and Yeung, K. W. K. (2017). Bone grafts and biomaterials substitutes for bone defect repair: a review. Bioact. Mater 2 (4), 224–247. doi:10.1016/j.bioactmat.2017.05.007
Wang, Y. F., Liu, L., Xue, X., and Liang, X. J. (2017). Nanoparticle-based drug delivery systems: what can they really do in vivo? F1000Res 6, 681. doi:10.12688/f1000research.9690.1
Wang, Z., Ma, K., Jiang, X., Xie, J., Cai, P., Li, F., et al. (2020). Electrospun poly(3-hydroxybutyrate-co-4-hydroxybutyrate)/Octacalcium phosphate Nanofibrous membranes for effective guided bone regeneration. Mater Sci. Eng. C Mater Biol. Appl. 112, 110763. doi:10.1016/j.msec.2020.110763
Ward, E. (2022). A review of tissue engineering for periodontal tissue regeneration. J. Vet. Dent. 39 (1), 49–62. doi:10.1177/08987564211065137
Weadock, K. S., Miller, E. J., Bellincampi, L. D., Zawadsky, J. P., and Dunn, M. G. (1995). Physical crosslinking of collagen fibers: comparison of ultraviolet irradiation and dehydrothermal treatment. J. Biomed. Mater Res. 29 (11), 1373–1379. doi:10.1002/jbm.820291108
Wessing, B., Lettner, S., and Zechner, W. (2018). Guided bone regeneration with collagen membranes and particulate graft materials: a systematic review and meta-analysis. Int. J. Oral Maxillofac. Implants 33 (1), 87–100. doi:10.11607/jomi.5461
Wissing, T. B., Bonito, V., Bouten, C. V. C., and Smits, A. (2017). Biomaterial-driven in situ cardiovascular tissue engineering-a multi-disciplinary perspective. NPJ Regen. Med. 2, 18. doi:10.1038/s41536-017-0023-2
Won, J. Y., Park, C. Y., Bae, J. H., Ahn, G., Kim, C., Lim, D. H., et al. (2016). Evaluation of 3D printed PCL/PLGA/β-TCP versus collagen membranes for guided bone regeneration in a beagle implant model. Biomed. Mater 11 (5), 055013. doi:10.1088/1748-6041/11/5/055013
Wu, C., Miron, R., Sculean, A., Kaskel, S., Doert, T., Schulze, R., et al. (2011). Proliferation, differentiation and gene expression of osteoblasts in boron-containing associated with dexamethasone deliver from mesoporous bioactive glass scaffolds. Biomaterials 32 (29), 7068–7078. doi:10.1016/j.biomaterials.2011.06.009
Wu, G., Deng, H., Jiang, T., Tu, H., Chen, J., Zhan, Y., et al. (2017). Regulating the gaps between folds on the surface of silk fibroin membranes via LBL deposition for improving their biomedical properties. Colloids Surf. B Biointerfaces 154, 228–238. doi:10.1016/j.colsurfb.2017.02.038
Xia, Q., Zhang, Y., Li, Z., Hou, X., and Feng, N. (2019). Red blood cell membrane-camouflaged nanoparticles: a novel drug delivery system for antitumor application. Acta Pharm. Sin. B 9 (4), 675–689. doi:10.1016/j.apsb.2019.01.011
Xie, Y., Li, S., Zhang, T., Wang, C., and Cai, X. (2020). Titanium mesh for bone augmentation in oral implantology: current application and progress. Int. J. Oral Sci. 12 (1), 37. doi:10.1038/s41368-020-00107-z
Xue, J., Shi, R., Niu, Y., Gong, M., Coates, P., Crawford, A., et al. (2015). Fabrication of drug-loaded anti-infective guided tissue regeneration membrane with adjustable biodegradation property. Colloids Surf. B Biointerfaces 135, 846–854. doi:10.1016/j.colsurfb.2015.03.031
Xue, Y., Zhu, Z., Zhang, X., Chen, J., Yang, X., Gao, X., et al. (2021). Accelerated bone regeneration by MOF modified multifunctional membranes through enhancement of osteogenic and angiogenic performance. Adv. Healthc. Mater 10 (6), e2001369. doi:10.1002/adhm.202001369
Yamano, S., Haku, K., Yamanaka, T., Dai, J., Takayama, T., Shohara, R., et al. (2014). The effect of a bioactive collagen membrane releasing PDGF or GDF-5 on bone regeneration. Biomaterials 35 (8), 2446–2453. doi:10.1016/j.biomaterials.2013.12.006
Yazdani, J., Ahmadian, E., Sharifi, S., Shahi, S., and Maleki Dizaj, S. (2018). A short view on nanohydroxyapatite as coating of dental implants. Biomed. Pharmacother. 105, 553–557. doi:10.1016/j.biopha.2018.06.013
Zahid, S., Khan, A. S., Chaudhry, A. A., Ghafoor, S., Ain, Q. U., Raza, A., et al. (2019). Fabrication, in vitro and in vivo studies of bilayer composite membrane for periodontal guided tissue regeneration. J. Biomater. Appl. 33 (7), 967–978. doi:10.1177/0885328218814986
Zelikman, H., Slutzkey, G., Rosner, O., Levartovsky, S., Matalon, S., and Beitlitum, I. (2022). Bacterial growth on three non-resorbable polytetrafluoroethylene (PTFE) membranes-an in vitro study. Mater. (Basel) 15 (16), 5705. doi:10.3390/ma15165705
Zhang, H. Y., Jiang, H. B., Kim, J. E., Zhang, S., Kim, K. M., and Kwon, J. S. (2020). Bioresorbable magnesium-reinforced PLA membrane for guided bone/tissue regeneration. J. Mech. Behav. Biomed. Mater 112, 104061. doi:10.1016/j.jmbbm.2020.104061
Zhang, J., Ma, S., Liu, Z., Geng, H., Lu, X., Zhang, X., et al. (2017). Guided bone regeneration with asymmetric collagen-chitosan membranes containing aspirin-loaded chitosan nanoparticles. Int. J. Nanomedicine 12, 8855–8866. doi:10.2147/ijn.S148179
Zhang, W., Li, P., Shen, G., Mo, X., Zhou, C., Alexander, D., et al. (2021). Appropriately adapted properties of hot-extruded Zn-0.5Cu-xFe alloys aimed for biodegradable guided bone regeneration membrane application. Bioact. Mater 6 (4), 975–989. doi:10.1016/j.bioactmat.2020.09.019
Zhang, Z. Y., Teoh, S. H., Chong, M. S., Schantz, J. T., Fisk, N. M., Choolani, M. A., et al. (2009). Superior osteogenic capacity for bone tissue engineering of fetal compared with perinatal and adult mesenchymal stem cells. Stem Cells 27 (1), 126–137. doi:10.1634/stemcells.2008-0456
Zheng, X., Ke, X., Yu, P., Wang, D., Pan, S., Yang, J., et al. (2020). A facile strategy to construct silk fibroin based GTR membranes with appropriate mechanical performance and enhanced osteogenic capacity. J. Mater Chem. B 8 (45), 10407–10415. doi:10.1039/d0tb01962c
Zhao, S., Pinholt, E. M., Madsen, J. E., and Donath, K. (2000). Histological evaluation of different biodegradable and non-biodegradable membranes implanted subcutaneously in rats. J. Craniomaxillofac Surg. 28 (2), 116–122. doi:10.1054/jcms.2000.0127
Zhou, T., Chen, S., Ding, X., Hu, Z., Cen, L., and Zhang, X. (2021). Fabrication and characterization of collagen/PVA dual-layer membranes for periodontal bone regeneration. Front. Bioeng. Biotechnol. 9, 630977. doi:10.3389/fbioe.2021.630977
Keywords: membranes, GBR, periodontal bone defects, bone regenaration, periodontitis
Citation: Wang D, Zhou X, Cao H, Zhang H, Wang D, Guo J and Wang J (2023) Barrier membranes for periodontal guided bone regeneration: a potential therapeutic strategy. Front. Mater. 10:1220420. doi: 10.3389/fmats.2023.1220420
Received: 10 May 2023; Accepted: 10 November 2023;
Published: 29 November 2023.
Edited by:
Arun Prabhu Rameshbabu, Harvard Medical School, United StatesReviewed by:
Patricia González-Alva, National Autonomous University of Mexico, MexicoCopyright © 2023 Wang, Zhou, Cao, Zhang, Wang, Guo and Wang. This is an open-access article distributed under the terms of the Creative Commons Attribution License (CC BY). The use, distribution or reproduction in other forums is permitted, provided the original author(s) and the copyright owner(s) are credited and that the original publication in this journal is cited, in accordance with accepted academic practice. No use, distribution or reproduction is permitted which does not comply with these terms.
*Correspondence: Jianming Guo, Z2ptOTExMjM5QDEyNi5jb20=; Jifeng Wang, d2FuZ2ppZmVuZ0BzdXN0ZWNoLWhvc3BpdGFsLmNvbQ==
†These authors have contributed equally to this work
Disclaimer: All claims expressed in this article are solely those of the authors and do not necessarily represent those of their affiliated organizations, or those of the publisher, the editors and the reviewers. Any product that may be evaluated in this article or claim that may be made by its manufacturer is not guaranteed or endorsed by the publisher.
Research integrity at Frontiers
Learn more about the work of our research integrity team to safeguard the quality of each article we publish.