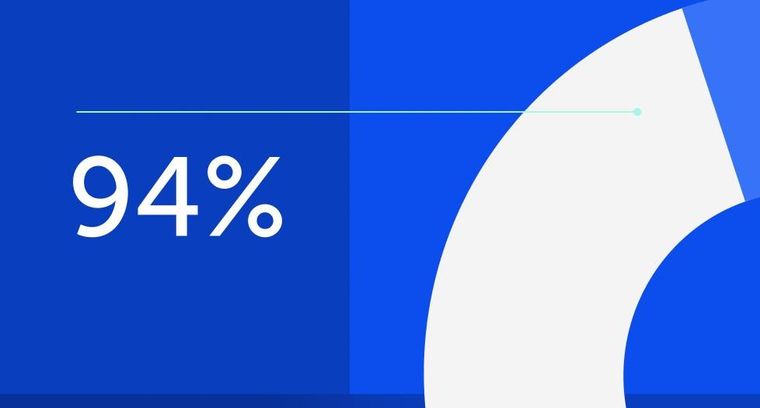
94% of researchers rate our articles as excellent or good
Learn more about the work of our research integrity team to safeguard the quality of each article we publish.
Find out more
ORIGINAL RESEARCH article
Front. Mater., 11 May 2023
Sec. Structural Materials
Volume 10 - 2023 | https://doi.org/10.3389/fmats.2023.1204083
Compared with polyvinyl alcohol-engineered cementitious composites (PVA-ECC), engineered cementitious composites containing polyethylene fibers (PE-ECC) has captured the attention of researchers because of lower cost. Nevertheless, there is a limited amount of research that focuses on their dynamic behavior. Thus, specimens with different water binder ratios were tested by split Hopkinson pressure bar (SHPB) to unveil the dynamic compressive behavior of PE-ECC. The dynamic behaviors were analyzed based on the tests, including dynamic failure modes, dynamic stress-strain curves, dynamic compressive strength, dynamic peak strain, and dynamic toughness. The result shows the PE-ECC has excellent compressive ductility, and a long post-peak descending part in the stress-strain relations. Furthermore, the sensitivity analysis reveals that the strain rate is the most crucial factor that directly affects the dynamic compressive strength, dynamic peak strain, and dynamic toughness in a monotonic fashion.
ECC is a random short fiber reinforced high-performance cement-based composite (Li et al., 1992). Different from brittle concrete, it has a tensile strain hardening effect and good tensile and compressive ductility because of the addition of fibers (Yu et al., 2020). Because of the excellent mechanical properties, with the continuous development of research and engineering practice, ECC has been applied to structural earthquake resistance (columns, coupling beams, joints, piers, etc.), dam repair, and composite bridge decks, etc. (Kunieda et al., 2006; Park et al., 2011; Hou et al., 2018; Li, 2019).
Previous studies have indicated that PVA-ECC has excellent mechanical properties under dynamic load (Yu et al., 2018; Cai et al., 2023). Kai et al. (2017) conducted SHPB tests on three kinds of PVA-ECC with different water binder ratios and superplasticizer dosage but almost the same static compressive strength. It shows that PVA-ECC is a strain-rate-sensitive material. Dynamic peak stress and dynamic peak strain of PVA-ECC increase, with the increase of strain rate. There is a linear relationship between the dynamic increase factor (DIF, ratio of the dynamic compressive strength to the static compressive strength) and the common logarithm of the strain rate. Li et al. (2020) studied the dynamic properties of PVA-ECC with different PVA fiber volume content and matrix strength under impact load. The results show that the dynamic mechanical properties of PVA-ECC with different PVA fiber volume content are sensitive to the strain rate, and the dynamic compressive strength, dynamic peak strain, and dynamic toughness increase, with the increase of the strain rate. The PVA fiber volume content has a great influence on the dynamic mechanical properties of PVA-ECC under impact load, especially on the post-peak toughness. The DIF is less affected by the PVA fiber volume content. The dynamic compressive strength increases with an increase in the static compressive strength. However, the dynamic peak strain is less affected by the static compressive strength.
In order to improve the utilization rate of solid waste and increase matrix strength, a variety of solid waste was added into ECC, such as ceramic waste and granular blast furnace slag (GGBS) (Chen et al., 2013; Yu et al., 2017; Xiong et al., 2021; Yu et al., 2023). Chen et al. (2013) used GGBS to replace part of the fly ash of PVA-ECC and conducted SHPB tests. The results show that the dynamic compressive strength of GGBS-ECC increases, with the increase of the strain rate, but the dynamic peak strain decreases, with the increase of the strain rate. The strain rate has little effect on the dynamic toughness.
To reduce the cost of ECC, cheaper fibers such as PP fibers and steel fibers were used to partially replace the expensive PVA fibers to form hybrid fiber ECC (Wang et al., 2015; Yang et al., 2015; Lin et al., 2019). Yang et al. (2015) used SHPB to study the dynamic mechanical properties of steel-PVA hybrid fibers ECC with different mix proportions. The result shows that the hybrid fibers ECC are also strain rate sensitive material. Steel fibers can improve the dynamic compressive strength and dynamic toughness at a high strain rate. The PVA fibers can improve deformation capacity but have little effect on the dynamic compressive strength. Li et al. (2016) studied the effect of steel fibers (0.0%–1.5%) on the dynamic mechanical properties of hybrid fibers ECC (steel fibers +2.0% PVA fibers) under impact load, including specimen dynamic failure modes, dynamic compressive strength, and dynamic toughness. The results show that the addition of steel fiber can significantly improve the dynamic mechanical properties of ECC. The strain rate sensitivity of the hybrid fiber ECC decreased, with the increase of steel fiber content.
At present, most of the fibers added to ECC are PVA fibers produced by the Kuraray Group. ECC using Kuraray PVA fibers has good mechanical properties, but the high price of the PVA fibers has hindered the large-scale application of ECC. For this reason, many researchers have explored the use of low-cost fiber to prepare ECC. ECC with low-cost PE fiber made in China showed good static properties (Yang, 2022). In this study, the dynamic mechanical properties of PE-ECC specimens with different water binder ratios under impact load were studied by using SHPB with a diameter of 80 mm. The dynamic failure modes, dynamic stress-strain curves, dynamic compressive strength, dynamic peak strain, and dynamic toughness of the PE-ECC specimens with different water binder ratios and strain rates were analyzed. The results of this study could provide a reference for the application of PE-ECC in impact-resistant structures.
The SHPB device with a diameter of 80 mm was used in this study (as shown in Figures 1, 2). The SHPB device is an important piece of equipment for studying the dynamic mechanical properties of materials, including loading system, pressure bar system, data acquisition system, and data processing system. The strain of the incident bar and transmission bar could be obtained by strain gauges on the surfaces of the incident bar and transmission bar. Applying one-dimensional elastic wave theory, the velocity difference between the front and rear surfaces of specimens could be calculated. Dividing the velocity difference by specimen thickness, the strain rates of the specimens could be obtained. Then the strain rates could be integrated to obtain strains of the specimens. According to the one-dimensional elastic wave theory, the loadings acting on the specimens by the incident bar and the transmission bar could also be calculated to obtain the stresses of the specimens. Finally, the SHPB device obtains the dynamic stress-strain relationship of the specimens in the whole process of the tests through indirect measurement, which avoids the difficulty of direct measurement.
Materials used to prepare PE-ECC in this study include P.O 42.5R ordinary Portland cement, the PE fibers (detailed parameters are presented in Table 1), polycarboxylic superplasticizer, 100 mesh quartz sand, Grade I fly ash, and thickener. Three mixing proportions with different water binder ratios were adopted as presented in Table 2.
A 15 L mortar mixer (maximum rotational speed 140 r/min) was used for mixing. Mixing procedures were as follows: 1) mixing cement, quartz sand, fly ash, and thickener for 2 min; 2) adding water, superplasticizer, and thickener mixing for 3 min; 3) adding the PE fibers. Continue mixing was applied for at least 5 min until the PE fibers were evenly distributed without agglomeration. Poured the mixture into molds, and vibrated for 2 min. Cubic specimens with a side length of 100 mm were fabricated in this study for testing static compressive strength, which was made according to Chinese Standard JC/T 2461-2018 (Standard Test Method for the Mechanical Properties of Ductile Fiber Reinforced Cementitious Composites) (MIIT, 2018). Cylinders with a diameter of 70 mm and height of 300 mm were fabricated. Before the tests, the cylinders were cut into cylinder specimens with a diameter of 70 mm and height of 35 mm for the SHPB tests and cylinder specimens with a diameter of 70 mm and height of 140 mm for the static compressive strength tests.
The static compressive strength test results of the cubic specimens and the cylindrical specimens are shown in Table 3. Three specimens were prepared for each mix proportion, and the results are mean values. With the increase of the water binder ratio, the static compressive strength decreases gradually. The typical failure mode of the specimens for the static compressive strength test is shown in Figure 3. The specimens showed strong integrity after the tests since the fragments were connected by the PE fibers.
FIGURE 3. Typical failure mode for the static compressive strength tests. (A) Cubic specimen. (B) Cylindrical specimen.
In the SHPB tests, at a relatively low strain rate, the broken specimens showed certain integrity (most fragments were connected through PE fibers), which was similar to the results of the static compressive strength tests. At a relatively high strain rate, the specimens were damaged seriously with small fragments. There were few PE fibers connecting the fragments. The failure modes of the specimens of mix M1 under different loading pressures are shown in Figure 4.
In the SHPB tests, three loading pressures (0.65, 0.85, and 1.05 MPa) were used for each mix proportion, and the corresponding incident bar speeds were 8.22, 9.90, and 11.56 m/s respectively. The dynamic stress-strain curves obtained in this study is shown in Figure 5. Each dynamic stress-strain curve is the average of the test results of three specimens. (Because of equipment failure, only one specimen in the 1–0.65 group had collected valid data.) The strain rate, dynamic compressive strength, dynamic peak strain, and dynamic increase factor (DIF, ratio of the dynamic compressive strength to the cylindrical compressive strength) are shown in Table 4.
FIGURE 5. Dynamic stress-strain curves. (A) Mix proportion M1. (B) Mix proportion M2. (C) Mix proportion M3.
Because of the existence of the PE fibers, the stress-strain curves have a long post-peak descending section. The mechanical properties of the specimens with different mix proportions exhibit strain rate sensitivity. The dynamic compressive strength and dynamic peak strain increase, with the increase of strain rate. For the specimens with the same mix proportion, the curves decrease gently with a relatively low strain rate, and the curves decrease steeply with a relatively high strain rate. This corresponds to the dynamic failure modes of the specimens: the specimens with a relatively low-strain rate still have certain integrity after failure because of the bridging effect of the PE fibers, which leads to a gentle decrease in bearing capacity. On the contrary, the PE fibers cannot maintain the integrity of the specimens with a relatively high strain rate, and the specimens were damaged seriously, which leads to a steep decrease in bearing capacity. Under the same loading pressure, the strain rates of the specimens with different mix proportions are similar, and the comparison of stress-strain curves is shown in Figure 6. Under the same loading pressure, the higher the static compressive strength is, the steeper the falling section of the stress-strain curves is, and that means, the worse the ductility is.
FIGURE 6. Comparison of dynamic stress-strain curves of specimens with different mix proportions. (A) Loading pressure 0.65 MPa. (B) Loading pressure 0.85 MPa. (C) Loading pressure 1.05 MPa.
It can be seen from Figure 5 and Table 4 that the dynamic compressive strength of the specimens increases, with the increase of strain rate. This obvious strain rate hardening phenomenon could be explained by the theory of energy. The failure of the specimens was due to the generation and development of cracks. The higher strain rate led to more cracks, more serious damage, and more energy to reach failure. However, the higher strain rate would lead to a shorter collision time, which resulted in a shorter time for the material to accumulate energy and a shorter buffer time. Enough energy could be accumulated for the generation and development of cracks only by increasing its stress (Li et al., 2008). Under the same loading pressure, the higher the static compressive strength was, the higher the corresponding dynamic compressive strength was.
In the static compressive strength tests, the maximum peak compressive strain is 0.44% (M1). In this study, the minimum dynamic peak strain of the specimen was 0.673% (1–0.65), and the maximum was 0.986% (2–1.05). The dynamic peak strain is sensitive to strain rate. The relationship between the common logarithm of strain rate and dynamic peak strain is obtained by linear fitting:
where
Toughness refers to the energy dissipated by the material during deformation. It is a comprehensive index of material strength and deformation capacity. It can be calculated by the area between the stress-strain curves and abscissa axes. In this study, taking the peak strain as a boundary, areas between the abscissa axes and rising section (Sup), falling section (Sdown) of the stress-strain curves, and the complete curves were calculated respectively to evaluate the dynamic toughness. The dynamic toughness indexes of PE-ECC with three mix proportions under different loading pressures are shown in Table 5. The dynamic toughness is sensitive to strain rate: the higher the strain rate is, the better the dynamic toughness is. As shown in Figure 8, with the same loading pressure, the higher the water binder ratio is, the worse the dynamic toughness becomes. Although the higher water binder ratio makes PE-ECC have better deformation ability, it also reduces its compressive strength, and finally reduces the dynamic toughness. The energy dissipated in the rising section of the stress-strain curves was little (The maximum is only 16.5%). Most of the energy was dissipated through the descending section, which means that the addition of PE fibers greatly improves the dynamic toughness of the material.
In this study, we present the effects of water binder ratio and strain rate on the mechanical properties of the PE-ECC specimens under impact load. Based on the static compressive strength tests and the SHPB tests of PE-ECC specimens with three mix proportions, the following conclusions could be obtained:
1. The PE fibers in PE-ECC could change the failure mode of the specimens. In the static compressive strength tests and the SHPB tests with relatively low strain rates, the broken specimens had certain integrity. (Most fragments were connected through PE fibers.) In the SHPB tests with a high strain rate, the specimens were damaged seriously, and the size of the fragments was small. In addition, there was no phenomenon that the fragments were connected through PE fibers.
2. The PE-ECC specimens have excellent compressive ductility, and the stress-strain curves have a long post-peak descending section. The mechanical property is sensitive to strain rates. The dynamic compressive strength increases, with the increase of strain rate. For the specimens with the same mix proportion, the curves decrease gently with a relatively low strain rate, and the curves decrease steeply with a relatively high strain rate.
3. The dynamic peak strain and the dynamic toughness are sensitive to the strain rate. The dynamic peak strain increases linearly with the common logarithm of strain rate. The higher the strain rate is, the better the dynamic toughness is. For specimens with the same loading pressure, the higher the water binder ratio is, the worse the dynamic toughness becomes.
The raw data supporting the conclusion of this article will be made available by the authors, without undue reservation.
LL and JX conceived and designed the study. LL and ZW performed the experiments. LL, JX, and ZW wrote the paper. LL and JX reviewed and edited the manuscript. All authors contributed to the article and approved the submitted version. All authors listed have made a substantial, direct, and intellectual contribution to the work and approved it for publication.
The study was funded by the National Natural Science Foundation of China (Grant No. 52278160), the Innovation Project of Guangdong Graduate Education (Grant No. 2019JGXM101), Young innovative talents project of regular universities in Guangdong Province (Grant No. 2018KQNCX278), and Guangdong Natural Science Foundation (Grant Nos 2020A1515110814 and 2023A1515012081).
The authors gratefully acknowledge the laboratory of School of Transportation, Civil Engineering and Architecture, Foshan University for providing the resources required for this study.
The authors declare that the research was conducted in the absence of any commercial or financial relationships that could be construed as a potential conflict of interest.
All claims expressed in this article are solely those of the authors and do not necessarily represent those of their affiliated organizations, or those of the publisher, the editors and the reviewers. Any product that may be evaluated in this article, or claim that may be made by its manufacturer, is not guaranteed or endorsed by the publisher.
Cai, J. M., Pan, J. L., Lin, Y. Z., Han, J. S., Ding, B., and Ukrainczyk, N. (2023). Effect of temperature on the low-velocity impact behaviors of engineered cementitious composite. J. Mater. Civ. Eng. 35 (7), 04023167. doi:10.1061/JMCEE7.MTENG-15023
Chen, Z. T., Yang, Y. Z., and Yao, Y. (2013). Quasi-static and dynamic compressive mechanical properties of engineered cementitious composite incorporating ground granulated blast furnace slag. Mater. Des. 44 (FEB), 500–508. doi:10.1016/j.matdes.2012.08.037
Hou, W., Xu, S. L., Ji, D. S., Li, Q. H., and Lin, G. (2018). Cyclic performance of steel plate-reinforced high toughness-concrete coupling beams with different span-to-depth ratios. J. Struct. Eng. 144 (10), 04018170. doi:10.1061/(ASCE)ST.1943-541X.0002175
Kai, M. F., Xiao, Y., Shuai, X. L., and Ye, G. (2017). Compressive behavior of engineered cementitious composites under high strain-rate loading. J. Mater. Civ. Eng. 29 (4), 04016254. doi:10.1061/(ASCE)MT.1943-5533.0001781
Kunieda, M., and Rokugo, K. (2006). Recent progress on HPFRCC in Japan required performance and applications. J. Adv. Concr. Technol. 4 (1), 19–33. doi:10.3151/jact.4.19
Li, Q., Zhao, H. X., Xu, S. L., and Gao, X. (2016). Influence of steel fiber on dynamic compressive behavior of hybrid fiber ultra high toughness cementitious composites at different strain rates. Constr. Build. Mater 125, 490–500. OCT.30. doi:10.1016/j.conbuildmat.2016.08.066
Li, V. C. (2019). Engineered cementitious composites (ECC): Bendable concrete for sustainable and resilient infrastructure. Berlin, Germany: Springer-Verlag Berlin Heidelberg.
Li, V. C., and Leung, C. K. Y. (1992). Steady-state and multiple cracking of short random fiber composites. J. Eng. Mech. 118 (11), 2246–2264. doi:10.1061/(asce)0733-9399(1992)118:11(2246)
Li, W. M., Xu, J. Y., Shen, L. J., and Li, Q. (2008). Dynamic mechanical properties of basalt fiber reinforced concrete using a split Hopkinson pressure bar. Acta Mater. Compos. Sin. 25 (2), 135–142. doi:10.13801/j.cnki.fhclxb.2008.02.029
Li, Y., Zhang, W. B., and Liu, Z. J. (2020). Study on dynamic compressive properties of PVA-ECC. J. Build. Mater. 23 (3), 513–520. doi:10.3969/j.issn.1007-9629.2020.03.005
Lin, J. X., Song, Y., Xie, Z. H., Guo, Y. C., Wei, X., Zeng, J. J., et al. (2019). Static and dynamic mechanical behavior of engineered cementitious composites with PP and PVA fibers. J. Build. Eng. 29, 101097. doi:10.1016/j.jobe.2019.101097
Miit, (2018). Standard test method for the mechanical properties of ductile fiber reinforced cementitious composites. Beijing, China: Ministry of Industry and Information Technology. PRC.
Park, W. S., and Yun, H. D. (2011). Seismic performance of pseudo strain-hardening cementitious composite coupling beams with different reinforcement details. Compos. B Eng. 42 (6), 1427–1445. doi:10.1016/j.compositesb.2011.04.049
Wang, Z. B., Zhang, J., Wang, J. H., and Shi, Z. J. (2015). Tensile performance of polyvinyl alcohol–steel hybrid fiber reinforced cementitious composite with impact of water to binder ratio. J. Compos. Mater. 49 (18), 1–19. doi:10.1177/0021998314542450
Xiong, Y., Xu, G. Z., Wu, D., Fang, S., and Tang, Y. F. (2021). Investigation of using the ceramic polishing brick powder in engineered cementitious composites. J. Build. Eng. 43, 102489. doi:10.1016/j.jobe.2021.102489
Yang, H. X., Huang, Y. S., and Li, J. (2015). Dynamic mechanical properties of hybrid fiber-reinforced cement-based composites. J. South China Univ. Technol. Nat. Sci. Ed. 43 (7), 50–56. doi:10.3969/j.issn.1000-565X.2015.07.008
Yang, Y. (2022). Experimental research on ECC mechanical properties of hybrid fibers containing ceramic waste at high temperature. Guangdong Province, China: South China University of Technology.
Yu, K. Q., Li, L. Z., Yu, J. T., Wang, Y. C., Yue, Y. H., and Xu, Q. F. (2018). Direct tensile properties of engineered cementitious composites: A review. Constr. Build. Mater 165, 346–362. doi:10.1016/j.conbuildmat.2017.12.124
Yu, K. Q., Lin, M. F., Tian, L. K., and Ding, Y. (2023). Long-term stable and sustainable high-strength engineered cementitious composite incorporating limestone powder. Structures 47, 530–543. JAN. doi:10.1016/j.istruc.2022.10.008
Yu, K. Q., Lu, Z. D., Dai, J. G., and Shah, S. P. (2020). Direct tensile properties and stress–strain model of UHP-ECC. J. Mater. Civ. Eng. 32 (1), 04019334. doi:10.1061/(ASCE)MT.1943-5533.0002975
Keywords: impact load, PE-ECC, SHPB, dynamic stress-strain curve, dynamic toughness
Citation: Liu L, Xiao J and Wu Z (2023) Experimental study on compressive behavior of PE-ECC under impact load. Front. Mater. 10:1204083. doi: 10.3389/fmats.2023.1204083
Received: 11 April 2023; Accepted: 03 May 2023;
Published: 11 May 2023.
Edited by:
Kequan Yu, Tongji University, ChinaReviewed by:
Xingyan Shang, Shandong Jianzhu University, ChinaCopyright © 2023 Liu, Xiao and Wu. This is an open-access article distributed under the terms of the Creative Commons Attribution License (CC BY). The use, distribution or reproduction in other forums is permitted, provided the original author(s) and the copyright owner(s) are credited and that the original publication in this journal is cited, in accordance with accepted academic practice. No use, distribution or reproduction is permitted which does not comply with these terms.
*Correspondence: Lingfei Liu, bGluZ2ZlaWxpdUBmb3N1LmVkdS5jbg==
Disclaimer: All claims expressed in this article are solely those of the authors and do not necessarily represent those of their affiliated organizations, or those of the publisher, the editors and the reviewers. Any product that may be evaluated in this article or claim that may be made by its manufacturer is not guaranteed or endorsed by the publisher.
Research integrity at Frontiers
Learn more about the work of our research integrity team to safeguard the quality of each article we publish.