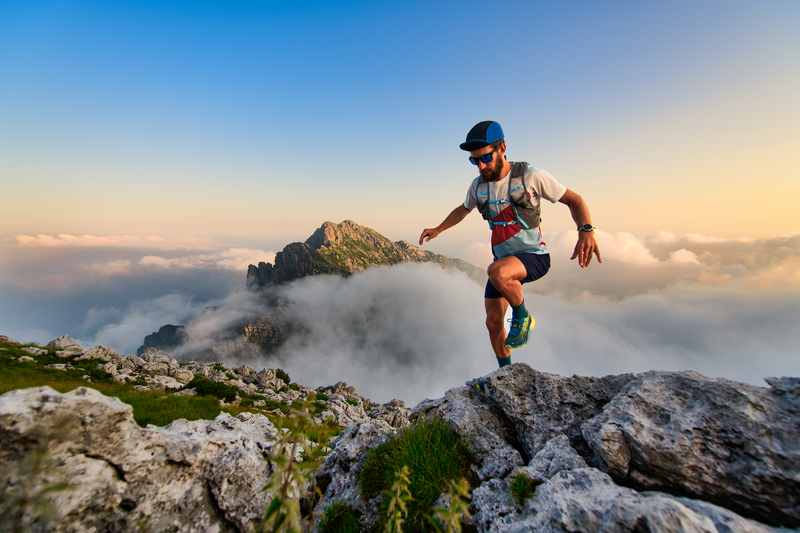
95% of researchers rate our articles as excellent or good
Learn more about the work of our research integrity team to safeguard the quality of each article we publish.
Find out more
MINI REVIEW article
Front. Mater. , 06 April 2023
Sec. Biomaterials and Bio-Inspired Materials
Volume 10 - 2023 | https://doi.org/10.3389/fmats.2023.1155643
This article is part of the Research Topic Advanced in situ Characterization of Biological Interfaces and Materials View all 5 articles
Microbially induced carbonate precipitation (MICP) mimics the natural cementation process that occurs in various geological settings by using the bicarbonate minerals resulting from various bacterial metabolic pathways as cementing agents. This bio-technique can be used to manufacture so-called “bio-bricks,” which rival regular bricks in strength and durability. In the last two decades, MICP has been increasingly utilized for the maintenance and repair of infrastructure. More recently, this process has also been shown to have great potential as an energy-saving and cost-effective means of in situ resource utilization (ISUR) to produce construction materials; these can be utilized for extraterrestrial human settlements for space programs such as lunar exploration. We thus review the description of natural cementation, the anaerobic and aerobic bacterial metabolic activities leading to calcium carbonate precipitation, the properties of the lunar regolith, the production of bio-bricks, and potential research needs.
Space exploration has been making great efforts to develop extraterrestrial human settlements. However, as estimated by the US National Aeronautics and Space Administration (NASA), it costs about $10,000 USD to put only one pound of material in Earth orbit. Thus, the in situ utilization of raw materials, also known as in situ resource utilization (ISRU), is an economic imperative. ISRU covers a wide range of production techniques, including the production of propellant, gases, and water for sustainable life support, and the production of construction materials for shelter (Pickett et al., 2020; Schlüter and Cowley, 2020). Scientists have considered and studied various techniques to transform ingredients (i.e., regolith/mined ores) to a construction phase on our Moon (Anand et al., 2012; Wilhelm and Curbach, 2014). Naser (2019) gave a detailed review of the main extraterrestrial processing methods, including melting/sintering, combustion, dry-mix/steam injection, and cold pressing.
The energy consumption of the aforementioned processing methods is daunting (Kara and Li, 2011; Qureshi et al., 2012). However, bio-cementation is being progressively recognized as a green solution for the application of bonding in construction materials. Bio-cementation uses microorganisms to induce the precipitation of carbonate minerals for construction. In the last two decades, microbially induced carbonate precipitation (MICP) has been increasingly utilized to maintain and repair infrastructures and has particularly been used to produce biological cement for sandstone bricks. More recently, numerous research articles have been published on the application of MICP to produce “bio-bricks” (Bernardi et al., 2014; Li et al., 2020b; Cheng et al., 2020; Nething et al., 2020; Zhao et al., 2020; Arab et al., 2021; Liu et al., 2021). This review focuses specifically on the metabolism of the MICP process, the properties of lunar regolith particles, and the characteristics of artificial bio-bricks.
Anaerobic microbes should surpass aerobic ones in respiration, given the fact that the moon lacks oxygen. In this review, the metabolic activities that induce carbonate precipitation are classified into aerobic and anaerobic groups. Microorganisms induce carbonate precipitation either by increasing pH, increasing dissolved inorganic carbon (DIC), or both. The process can be classified into several metabolic pathways—photosynthesis, ureolysis, ammonification, denitrification, sulfate reduction, denitrification, and methane oxidation (Jain, 2021). The major metabolism involved in microbial carbonate precipitation can be either aerobic (photosynthesis, ureolysis, and ammonification) or anerobic (sulfate reduction and denitrification). For anaerobic processes, the inorganic compounds sulfate (SO42-) and nitrate (NO3−) serve as electron acceptors for bacteria (Ersan, 2019). Methane oxidation is a metabolic process that can occur aerobically or anaerobically. Among these microbial metabolisms, ureolysis is the most studied MICP process due to its less complex and higher calcite precipitation (Wang et al., 2014; Castro-Alonso et al., 2019).
Photosynthesis is an autotrophic aerobic metabolic process that is reported to be feasible for MICP. Altermann et al. (2006) proposed that cyanobacteria can store Ca2+ and Mg2+ ions in organic envelopes and precipitate carbonate minerals within their sheaths, as well as extracellular polymeric substances. However, to satisfy the oversaturation status of Ca2+ and CO32- in aquatic environments, heterotrophic bacteria such as sulfate reducers must help remove sulfate and raise carbonate alkalinity. The interplay of cyanobacteria and heterotrophic bacteria are believed to have contributed to the Earth’s carbonate factory for the last 3 billion years. This mechanism has contributed to increased pH through photosynthesis by microalgae, resulting in the exchange of HCO3− and OH− across the membrane (Figure 1A). With pH of up to 10.329 (Pedersen et al., 2013), CO32- dominates the solution to reach supersaturation in the microenvironment around the cells. With calcium ions present in the microenvironment, carbonate minerals precipitate out of solution, inducing MICP. Photosynthesis utilizes HCO3− and decreases DIC but increases pH and CO32- concentration to induce MICP.
FIGURE 1. Factors that induce carbonate precipitation by different metabolisms. BP, byproduct; DIC, dissolved inorganic carbon. (A) Photosynthesis: (B) Ureolysis; (C) Ammonification; (D) Sulfate reduction; (E) Denitrification; (F) Aerobic methane oxidation; (G) Anaerobic methane oxidation.
Ureolysis is the enzymatic degradation of urea by ureases, producing carbonic acids and ammonia. The carbonic acid produced increases the concentration of DIC. Ammonia increases the pH of the environment by ammonia hydrolysis. In this case, ureolysis simultaneously influences the concentration of DIC and the pH of the environment (Figure 1B), leading to the precipitation of carbonate minerals along with the presence of calcium ions in the environment. The genus Bacillus harbors the most species currently used in MICP biotechnology. A prime example of this is Sporosarcina pasteurii, formerly Bacillus pasteurii, which is one of many species highly efficient in ureolysis due to its high levels of secreted urease; it is used repeatedly as a study model for MICP. Bacillus is known to harbor many urea hydrolyzing species, so this factor is often exploited and manipulated for biotechnological application (Table 1). B. sphaericus appears in numerous papers in use as another model organism for MICP via urea hydrolysis (Table 1) (Hammes et al., 2003; Van Tittelboom et al., 2010; Achal et al., 2011; De Muynck et al., 2011; Cheng et al., 2013; Wang et al., 2014; Erşan et al., 2016; Kim et al., 2017; Sharma et al., 2021). Other species of this genus are applied in a variety of biotechnological applications, including B. cereus in soil stability (Oualha et al., 2020), B. licheniformis in the cementation of sand (Saricicek et al., 2019; Šovljanski et al., 2021) and B. pseudofirmus in self-healing concrete (Jonkers et al., 2010). The availability of whole-genome sequence data for several of the many species within this genus makes Bacillus ideal for future MICP genetic bioengineering.
TABLE 1. Mechanism of precipitation induced by Bacillus species recorded in literature and capable of MICP.
The ammonification of amino acids is a metabolic process similar to ureolysis, in which microbes increase the DIC concentration as well as the pH of an environment. Amino acids contain both nitrogen and carbon, which are oxidized by heterogenic microbes under aerobic conditions to form NH3 and CO32- (Figure 1C). The hydrolysis process of ammonia product generates OH− around cells and creates a high local supersaturated environment of calcium carbonate. Rodriguez-Navarro et al. (2003) reported that the metabolic activity of Myxococcus xanthus involves the production of NH3 and CO2 by means of the oxidative deamination of amino acids. Apart from the modification of physical chemistry, both live and dead M. xanthus play a passive role in calcium carbonate precipitation by acting as a template for heterogeneous nucleation (Chekroun et al., 2004).
Sulfate reduction refers to reducing sulfate to sulfide coupled with anaerobic oxidizing organic carbon to bicarbonate, during which DIC and calcium concentrations increase Figure 1D). Specifically, the oxidation of organic acids increases the DIC concentration. More importantly, the release of calcium ions from gypsum (CaSO4) increases local Ca2+ concentration (Figure 2). Sulfate-reducing bacteria (SRB) have been reported to produce a copious amount of exopolymeric substances (EPSs) with buffering and Mg–Ca binding capacity, which controls the precipitation of the carbonate minerals by acting as templates for different carbonate minerals (Braissant et al., 2007; Bontognali et al., 2014; Qian et al., 2019). In addition, the nucleation of carbonate minerals occurs on the cell surface, as well as bacterial nanoglobules (Van Lith et al., 2003; Aloisi et al., 2006). Both their cell surface and EPS carry a net negative electric charge and have the capacity to bind Mg2+ and Ca2+ ions. Thus, SRB have been recognized as key to the precipitation of calcium carbonate, resulting in lithifying microbial communities (Baumgartner et al., 2006; Braissant et al., 2007). However, the application of sulfate reduction by SRB in concrete restoration has the problem of side-product H2S, which could cause corrosion of the concrete structure by its reaction with oxygen (Mori et al., 1991; Li et al., 2019). Many efforts have been made to control H2S production in anaerobic environments, such as using iron salts (Fe2+ + S2− → FeS) (Muñoz et al., 2015). More economically, the magnetite-assisted in situ microbial oxidation of H2S to S0 has been recently reported (Jung et al., 2020). Furthermore, elemental sulfur S0 can reduce CO2 into formic acid under ultraviolet light below 280 nm (Li et al., 2020a). Thus, state-of-the-art of physical/chemical and biological technology requires more research on the application of sulfate reduction in bioconcrete.
FIGURE 2. Schematic presentation of bacterial calcium metabolism and subsequent CaCO3 precipitation under high-Ca2+ extracellular conditions. Revised from Hammes et al. (2002). (A) Localized acidification; (B) ATP-dependent calcium pumps; (C) Localized alkalisation:(D) Carbonate precipitation.
Denitrification, like sulfate reduction, utilizes a highly oxidized-phase nitrate as the electron acceptor under anaerobic conditions, coupled with the oxidization of organic compounds. The denitrification process increases the pH in the microenvironment by consuming H+ and producing CO2 by oxidizing organic compounds, favoring carbonate precipitation (Figure 1E) (Lin et al., 2021). Recent research has demonstrated the potential of denitrification for improving the mechanical and hydraulic properties of soils (Pham et al., 2016; Hamdan et al., 2017; Keykha et al., 2018; Cheng and Shahin, 2019; Lin et al., 2021). The reduction of nitrate should produce the harmless terminal side-product N2. However, producing N2 requires four different enzymes involved in the denitrification process, or toxic nitrite and nitrous oxide might accumulate (Van Paassen et al., 2010). Denitrification has been evaluated as the most suitable MICP method for soil reinforcement by defeating ureolysis and sulfate reduction, based on four factors: substrate solubility, precipitation rate, carbonate yield, and the amount and type of by-product (Van Paassen et al., 2010).
Given the lack of oxygen on the moon or in space, anaerobic metabolic microbes should surpass aerobic ones when applied to MICP in space. Microbes that can use in situ minerals directly as the energy source to grow should generate considerable savings.
Methane oxidation drives the concentration of carbon dioxide in marine and freshwater sediments due to its aerobic and anaerobic oxidation. Methane serves as the electron donor; for methane oxidation, the type of electron acceptor determines its aerobic or anaerobic process. In aerobic conditions, methane mono-oxygenase converts methane to methanol, which is further converted into formate by another cell enzymatic activity (Jain, 2021). Oxygen seems to be the electron acceptor in this process. Formate must then be further oxidized to CO2. The increase of DIC concentration favors the precipitation of carbonate mineral around the microbial cell in the alkaline environment (Figure 1F). However, the aerobic oxidation of methane may lead to the dissolution of carbonates if the environment is not alkaline due to consequent increasing acidity from this process (Reeburgh, 2007). The anerobic oxidation of methane can use sulfate as the final electron acceptor. This process increases DIC concentration and the byproduct HS− (Figure 1G). Caesar et al. (2019) proposed that the MICP has potential application in the mitigation of methane release into the atmosphere due to anaerobic methane oxidation.
Microbes may form minerals authigenically or diagenetically. Microbial-induced carbonate mineral formation is passive in the environment to “cement” sand-sized material. Geologically, the distribution of cement varies with the degree of water filled in the pore spaces between the material particles (Figure 3). When completely filled with water, cements present as homogeneous fringes around material particles. The properties of sand-sized material on the moon should be considered because it determines the characteristic of pore spaces.
FIGURE 3. Distribution of cement under various environments. Revised from Scholle and Ulmer-Scholle, 1978.
On the lunar surface, regolith refers to fragmental and unconsolidated rock material—also known as lunar soil. The thickness of this layered regolith is estimated at about 2–15 m thick with a specific gravity ranging 2.9–3.5 g/cm3 (Shkuratov and Bondarenko, 2001; Taylor et al., 2003; Lin et al., 2020). Contributing to the formation of the lunar regolith are meteoroid impacts on the moon for over 4 billion years; furthermore, the lunar regolith may be recycled by unceasing impacts of large and small meteoroids (Lucey et al., 2006; Lin et al., 2020).
The chemistry of the lunar soil shows Si, Al, Fe, Mg, Ca, Ti, Na, K, Mn, and Cr as the major and minor abundant elements in bulk and size fractions (Papike et al., 1982). The chemical composition varies with grain size. In most cases, the smallest fraction (<10 um) is enriched in Al, Ca, Na, K, light rare earth elements, and Th and is depleted in Mg, Fe, Mn, and Sc. Moreover, the soils of the lunar maria are chemically distinct from highland soils.
The particle size of the lunar soil is consistently and broadly distributed (Morrison et al., 1970; Mitchell et al., 1972; Graf, 1993; Jolliff et al., 2000; Carrier, 2003; McCubbin et al., 2010). The size of 143 different lunar samples collected during the Apollo and Luna missions are cataloged by Graf (1993) and vary from 0.002 to 4 mm. Carrier (2003), the director of the Lunar Geotechnical Institute, describes the particle size of lunar soil to be sandy silt/silty sand, well-graded in geotechnical terms. The geotechnical particle size parameters are published with average mean particle size D50 = 72 μm, average coefficient of uniformity Cu = 16, and average coefficient of curvature Cc = 1.2 (Carrier, 2003). These parameters indicate that the Moon’s soil may be internally erodible and susceptible to “segregation piping” (Carrier, 2003). On the other hand, the lunar soil is characterized as very fine sand, very poorly sorted, nearly symmetrical, and geologically mesokurtic. These geological terms describe not merely particle size but also reflect the depositional environment. Obviously, meteorite impact is a very dynamic process, producing poorly sorted particles. Duke et al. (1970) proposed that it is caused by melting and consequent consolidation into clumps of finer particles on the Moon so that material finer than 15 um is less abundant. In this review, we tend to include both terms since the cementation process relates to geotechnical application and geological properties.
The mineralogy of the lunar soil is not very complicated and includes plagioclase (typical formula Ca2Al2Si2O8), pyroxene ((Ca,Mg,Fe)2Si2O6), ilmenite (FeTiO3), olivine ((Mg,Fe)2SiO4), and impact glass as major phases (Smith and Steele, 1976; Taylor et al., 2003). The simple mineralogy is produced in a very reducing environment. The remarkably distinct grain boundaries indicate no real alteration. Impact glass is apparently a product of frequent meteorite impacts on the lunar surface and can fuse minerals and rock fragments together (Taylor and Liu, 2010). Notably, all agglutinitic glasses contain myriads of nanophase Fe particles distributed in glass without touching each other, resulting in a relatively high magnetic susceptibility to agglutinitic glass (Taylor and Liu, 2010). Troilite is the only sulfide in the lunar regolith.
The grain-size property of the starting materials is the main factor to consider in laboratory experiments of artificially forming bio-bricks. Previous studies of the properties of artificial bio-bricks have tested their unconfined compressive strength to better establish the validity and feasibility of MICP biotechnology (Table 2). The grain sizes used to produce bio-bricks are normally with D50 in the range of 0.42–0.73 mm (Table 2). However, it is difficult to report a relationship between the grain size and the compressive strength of the correspondently produced bio-bricks since the same grain size might result in a different unconfined compressive strength under different saturation conditions (Bernardi et al., 2014; Cheng et al., 2020). Sporosarcina pasteurii—known as Bacillus pasteurii under older taxonomies (Yoon et al., 2001)—is a widely used model organism for making bio-bricks via MICP because it is highly productive in its secretion of extracellular urease. Arab et al. (2021) reported a hybrid technique to produce bio-bricks using enzyme-induced carbonate precipitation and sodium alginate biopolymer. This shows that carbonate precipitation without microorganisms can also produce bio-bricks.
The unconfined compressive strength of bio-bricks shows that they can have strengths of 1 MPa to 23 MPa (Table 2). The variation in unconfined compressive strength in different studies may be due to different reaction conditions. However, Nething et al. (2020) found a variation in strength between samples produced with the same parameters, which still requires further investigation of the cementation process. Regardless, bio-bricks have stress comparable to bricks prepared with more conventional cement and hydraulic lime additives (Bernardi et al., 2014). Previous studies have shown that the bio-bricks produced are comparable to cement-treated beams in terms of their mechanical properties and can also be considered an eco-friendly alternative to conventional bricks (Bernardi et al., 2014; Li et al., 2020b; Cheng et al., 2020; Nething et al., 2020; Zhao et al., 2020; Arab et al., 2021).
The recent surge in lunar exploration by several nations and continuing research on lunar samples have encouraged scientists to think more about ISRU. Regolith generated by meteoroid impacts is the most accessible resource on the Moon’s surface. By reviewing its properties, lunar regolith is the most suitable material with applicable grain size and mineralogy. Inspired by the natural geological cementation process by carbonate minerals, the artificial production of bricks on the Moon using in situ sand-sized regolith and mimicking MICP process shows promise. The mineralogy of material used in the artificial bio-brick experiments was mostly quartz or silica, and 0.4–1.0 M CaCl2 was added to serve as a Ca2+ source for the precipitation of carbonate minerals (Table 2). Minerals such as plagioclase and pyroxene in regolith on the Moon happen to contain Ca, which can be used as a Ca2+ source. Artificially cemented bio-bricks made out of sands closely resemble their natural counterparts with respect to the failure mechanism at micro-scale (Table 2). Few studies, in fact, have tested the idea of consolidating lunar simulant soil in the form of a brick with non-trivial strength properties (Dikshit et al., 2020; Kumar et al., 2020; Dikshit et al., 2022). However, the average mean particle size for regolith (D50 = 72 μm) is approximately one order smaller than those sands (0.36–0.73 mm) used to produce artificially cemented bio-bricks in Table 2. Sizing down the particles in laboratory conditions might help future field application.
Although MICP has been successfully demonstrated in the laboratory for many cases, its real application in the field of ISRU faces huge challenges. Since the conditions on the Moon cannot be controlled as in a laboratory, microorganisms are likely to suffer from harsh environments. Therefore, it is essential to establish a qualitative and quantitative simulation to manufacture bio-bricks by selecting a suitable and effective microbial species for the Moon’s extreme conditions. Microbes must overcome various stresses that suppress their ability to grow or their basic survival. Microbes have developed strategies such as the formation of cysts and spores, expression of repair enzymes for damage, changes in cellular membranes, and synthesis of molecules for relieving stresses for better survivability (Haruta and Kanno, 2015). The same species might present different microbial survivability under different conditions. Survival strategies can change from normal to micro-gravity, which may change microbial survivability. We must consider the fact that most microbial cells that provide nucleation sites for carbonate formation on Earth might change on the Moon, affecting dynamic cementation. In order to test the promise of MICP enabled bio-consolidation in extra-terrestrial conditions, it is essential to conduct low-gravity tests. Moreover, the generation of undesired by-products must be avoided. The upscaling of MICP from the laboratory to lunar application might present technical difficulties in obtaining homogeneous treatment. Finally, a substantial amount of water is needed for the bio-cementation process. Interestingly, water ice (H2O) and hydroxyl radicals (OH-) have been recently identified on the lunar surface (Li et al., 2018; Rubanenko et al., 2019). Although the source of water ice accumulating in permanently shaded regions on airless bodies in the inner Solar System still remains undetermined, the existence of water on the lunar surface would save in situ bio-cementation considerable costs. Urea is another indispensable component of ureolysis-based MICP, and is the primary choice of bio-brick production. Lambert and Randall (2019) have successfully used human urine as a sole source of urea for manufacturing bio-bricks. This could thus provide a potentially critical solution for obtaining urea in situ. Alternative cell-free systems should also be considered to completely avoid these technical challenges associated with culturing microbes for cell-based MICP. The cell-free urease system reported by Arab et al. (2021) serves as a good starting point in this direction. If successful, such catalysts with active enzymes could even be manufactured on Earth and cost-effectively shipped to the field of ISRU.
HZ designed this review due to her geology and geomicrobiology background as well as other published works in this area. HZ reviewed the literature, organized the structure of the manuscript, and wrote the manuscript. SN wrote and revised the manuscript. MX revised the manuscript. All authors read and approved the final manuscript.
This work was funded by the Science and Technology Development Fund, Macau SAR (File No. SKL-LPS (MUST)-2021-2023), and State Key Laboratory of Applied Microbiology, Southern China (Grant No. SKLAM008-2021).
The authors also wish to thank Dr. André Antunes for reading an early version of the article.
The authors declare that the research was conducted in the absence of any commercial or financial relationships that could be construed as a potential conflict of interest.
All claims expressed in this article are solely those of the authors and do not necessarily represent those of their affiliated organizations, or those of the publisher, the editors, and the reviewers. Any product that may be evaluated in this article, or claim that may be made by its manufacturer, is not guaranteed or endorsed by the publisher.
Achal, V., Pan, X., and Özyurt, N. (2011). Improved strength and durability of fly ash-amended concrete by microbial calcite precipitation. Ecol. Eng. 37 (4), 554–559. doi:10.1016/j.ecoleng.2010.11.009
Aloisi, G., Gloter, A., Kruger, M., Wallmann, K., Guyot, F., and Zuddas, P. (2006). Nucleation of calcium carbonate on bacterial nanoglobules. Geology 34 (12), 1017–1020. doi:10.1130/g22986a.1
Altermann, W., Kazmierczak, J., Oren, A., and Wright, D. (2006). Cyanobacterial calcification and its rock-building potential during 3.5 billion years of Earth history. Geobiology 4 (3), 147–166. doi:10.1111/j.1472-4669.2006.00076.x
Anand, M., Crawford, I. A., Balat-Pichelin, M., Abanades, S., Van Westrenen, W., Péraudeau, G., et al. (2012). A brief review of chemical and mineralogical resources on the Moon and likely initial in situ resource utilization (ISRU) applications. Planet. Space Sci. 74 (1), 42–48. doi:10.1016/j.pss.2012.08.012
Arab, M. G., Omar, M., Almajed, A., Elbaz, Y., and Ahmed, A. H. (2021). Hybrid technique to produce bio-bricks using enzyme-induced carbonate precipitation (EICP) and sodium alginate biopolymer. Constr. Build. Mater. 284, 122846. doi:10.1016/j.conbuildmat.2021.122846
Baumgartner, L. K., Reid, R. P., Dupraz, C., Decho, A. W., Buckley, D., Spear, J., et al. (2006). Sulfate reducing bacteria in microbial mats: Changing paradigms, new discoveries. Sediment. Geol. 185 (3-4), 131–145. doi:10.1016/j.sedgeo.2005.12.008
Bernardi, D., DeJong, J., Montoya, B., and Martinez, B. (2014). Bio-bricks: Biologically cemented sandstone bricks. Constr. Build. Mater. 55, 462–469. doi:10.1016/j.conbuildmat.2014.01.019
Bontognali, T. R., McKenzie, J. A., Warthmann, R. J., and Vasconcelos, C. (2014). Microbially influenced formation of Mg-calcite and Ca-dolomite in the presence of exopolymeric substances produced by sulphate-reducing bacteria. Terra nova. 26 (1), 72–77. doi:10.1111/ter.12072
Braissant, O., Decho, A. W., Dupraz, C., Glunk, C., Przekop, K. M., and Visscher, P. T. (2007). Exopolymeric substances of sulfate-reducing bacteria: Interactions with calcium at alkaline pH and implication for formation of carbonate minerals. Geobiology 5 (4), 401–411. doi:10.1111/j.1472-4669.2007.00117.x
Caesar, K., Kyle, J., Lyons, T., Tripati, A., and Loyd, S. (2019). Carbonate formation in salt dome cap rocks by microbial anaerobic oxidation of methane. Nat. Commun. 10 (1), 808–809. doi:10.1038/s41467-019-08687-z
Carrier, W. D. (2003). Particle size distribution of lunar soil. J. Geotechnical Geoenvironmental Eng. 129 (10), 956–959. doi:10.1061/(asce)1090-0241(2003)129:10(956)
Castro-Alonso, M. J., Montañez-Hernandez, L. E., Sanchez-Muñoz, M. A., Macias Franco, M. R., Narayanasamy, R., and Balagurusamy, N. (2019). Microbially induced calcium carbonate precipitation (MICP) and its potential in bioconcrete: Microbiological and molecular concepts. Front. Mater. 6, 126. doi:10.3389/fmats.2019.00126
Chekroun, K. B., Rodríguez-Navarro, C., González-Munoz, M. T., Arias, J. M., Cultrone, G., and Rodríguez-Gallego, M. (2004). Precipitation and growth morphology of calcium carbonate induced by myxococcus xanthus: Implications for recognition of bacterial carbonates. J. Sediment. Res. 74 (6), 868–876. doi:10.1306/050504740868
Cheng, L., Cord-Ruwisch, R., and Shahin, M. A. (2013). Cementation of sand soil by microbially induced calcite precipitation at various degrees of saturation. Can. Geotechnical J. 50 (1), 81–90. doi:10.1139/cgj-2012-0023
Cheng, L., Kobayashi, T., and Shahin, M. A. (2020). Microbially induced calcite precipitation for production of “bio-bricks” treated at partial saturation condition. Constr. Build. Mater. 231, 117095. doi:10.1016/j.conbuildmat.2019.117095
Cheng, L., and Shahin, M. A. (2019). “Microbially induced calcite precipitation (MICP) for soil stabilization,” in Ecological wisdom inspired restoration engineering (Singapore: Springer), 47–68.
De Muynck, W., Leuridan, S., Van Loo, D., Verbeken, K., Cnudde, V., De Belie, N., et al. (2011). Influence of pore structure on the effectiveness of a biogenic carbonate surface treatment for limestone conservation. Appl. Environ. Microbiol. 77 (19), 6808–6820. doi:10.1128/aem.00219-11
Dikshit, R., Dey, A., Gupta, N., Varma, S. C., Venugopal, I., Viswanathan, K., et al. (2020). Space bricks: From LSS to machinable structures via MICP. Ceram. Int. 47, 14892–14898. doi:10.1016/j.ceramint.2020.07.309
Dikshit, R., Gupta, N., Dey, A., Viswanathan, K., and Kumar, A. (2022). Microbial induced calcite precipitation can consolidate martian and lunar regolith simulants. Plos one 17 (4), e0266415. doi:10.1371/journal.pone.0266415
Duke, M. B., Woo, C. C., Bird, M. L., Sellers, G. A., and Finkelman, R. B. (1970). Lunar soil: Size distribution and mineralogical constituents. Science 167 (3918), 648–650. doi:10.1126/science.167.3918.648
Erşan, Y. Ç., Hernandez-Sanabria, E., Boon, N., and De Belie, N. (2016). Enhanced crack closure performance of microbial mortar through nitrate reduction. Cem. Concr. Compos. 70, 159–170. doi:10.1016/j.cemconcomp.2016.04.001
Ersan, Y. C. (2019). “Overlooked strategies in exploitation of microorganisms in the field of building materials,” in Ecological wisdom inspired restoration engineering (Berlin, Germany: Springer), 19–45.
Graf, J. C. (1993). Lunar soils grain size catalog. Washington, DC: National Aeronautics and Space Administration, Office of Management.
Hamdan, N., Kavazanjian, E., Rittmann, B. E., and Karatas, I. (2017). Carbonate mineral precipitation for soil improvement through microbial denitrification. Geomicrobiol. J. 34 (2), 139–146. doi:10.1080/01490451.2016.1154117
Hammes, F., and Verstraete, W. (2002). Key roles of pH and calcium metabolism in microbial carbonate precipitation. Reviews in environmental science and biotechnology. 1), 3–7. doi:10.1023/A:1015135629155
Haruta, S., and Kanno, N. (2015). Survivability of microbes in natural environments and their ecological impacts. Microbes Environ. 30 (2), 123–125. doi:10.1264/jsme2.me3002rh
Jain, S. (2021). “An overview of factors influencing microbially induced carbonate precipitation for its field implementation,” in Building materials for sustainable and ecological environment (Washington, United States: American Chemical Society), 73–99. doi:10.1007/978-981-16-1706-5_5
Jolliff, B. L., Gillis, J. J., Haskin, L. A., Korotev, R. L., and Wieczorek, M. A. (2000). Major lunar crustal terranes: Surface expressions and crust-mantle origins. J. Geophys. Res. Planets 105 (2), 4197–4216. doi:10.1029/1999je001103
Jonkers, H. M., Thijssen, A., Muyzer, G., Copuroglu, O., and Schlangen, E. (2010). Application of bacteria as self-healing agent for the development of sustainable concrete. Ecol. Eng. 36 (2), 230–235. doi:10.1016/j.ecoleng.2008.12.036
Jung, H., Baek, G., and Lee, C. (2020). Magnetite-assisted in situ microbial oxidation of H2S to S0 during anaerobic digestion: A new potential for sulfide control. Chem. Eng. J. 397, 124982. doi:10.1016/j.cej.2020.124982
Kara, S., and Li, W. (2011). Unit process energy consumption models for material removal processes. CIRP Ann. 60 (1), 37–40. doi:10.1016/j.cirp.2011.03.018
Keykha, H. A., Mohamadzadeh, H., Asadi, A., and Kawasaki, S. (2018). Ammonium-free carbonate-producing bacteria as an ecofriendly soil biostabilizer. Geotechnical Test. J. 42 (1), 20170353–20170429. doi:10.1520/gtj20170353
Kim, H. J., Shin, B., Lee, Y. S., and Park, W. (2017). Modulation of calcium carbonate precipitation by exopolysaccharide in Bacillus sp. JH7. Appl. Microbiol. Biotechnol. 101 (16), 6551–6561. doi:10.1007/s00253-017-8372-8
Kumar, A., Dikshit, R., Gupta, N., Jain, A., Dey, A., Nandi, A., et al. (2020). Bacterial growth induced biocementation Technology,'space brick'-A proposal for experiment at microgravity and planetary environments. BioRxiv 2020, 914853. doi:10.1101/2020.01.22.914853
Lambert, S. E., and Randall, D. G. (2019). Manufacturing bio-bricks using microbial induced calcium carbonate precipitation and human urine. Water Res. 160, 158–166. doi:10.1016/j.watres.2019.05.069
Li, S., Lucey, P. G., Milliken, R. E., Hayne, P. O., Fisher, E., Williams, J.-P., et al. (2018). Direct evidence of surface exposed water ice in the lunar polar regions. Proc. Natl. Acad. Sci. 115 (36), 8907–8912. doi:10.1073/pnas.1802345115
Li, X., O'Moore, L., Song, Y., Bond, P. L., Yuan, Z., Wilkie, S., et al. (2019). The rapid chemically induced corrosion of concrete sewers at high H2S concentration. Water Res. 162, 95–104. doi:10.1016/j.watres.2019.06.062
Li, Y., Li, Y., Liu, Y., Wu, Y., Wu, J., Wang, B., et al. (2020a). Photoreduction of inorganic carbon (+IV) by elemental sulfur: Implications for prebiotic synthesis in terrestrial hot springs. Sci. Adv. 6 (47), eabc3687. doi:10.1126/sciadv.abc3687
Li, Y., Wen, K., Li, L., Huang, W., Bu, C., and Amini, F. (2020b). Experimental investigation on compression resistance of bio-bricks. Constr. Build. Mater. 265, 120751. doi:10.1016/j.conbuildmat.2020.120751
Lin, H., Lin, Y., Yang, W., He, Z., Hu, S., Wei, Y., et al. (2020). New insight into lunar regolith-forming processes by the lunar rover Yutu-2. Geophys. Res. Lett. 47 (14), e2020GL087949. doi:10.1029/2020gl087949
Lin, W., Lin, W., Cheng, X., Chen, G., and Ersan, Y. C. (2021). Microbially induced desaturation and carbonate precipitation through denitrification: A review. Appl. Sci. 11 (17), 7842. doi:10.3390/app11177842
Liu, J., Li, G., and Li, X. a. (2021). Geotechnical engineering properties of soils solidified by microbially induced CaCO3 precipitation (MICP). Adv. Civ. Eng. 2021, 1–21. doi:10.1155/2021/6683930
Lucey, P., Korotev, R. L., Gillis, J. J., Taylor, L. A., Lawrence, D., Campbell, B. A., et al. (2006). Understanding the lunar surface and space-Moon interactions. Rev. mineralogy Geochem. 60 (1), 83–219. doi:10.2138/rmg.2006.60.2
McCubbin, F. M., Steele, A., Hauri, E. H., Nekvasil, H., Yamashita, S., and Hemley, R. J. (2010). Nominally hydrous magmatism on the Moon. Proc. Natl. Acad. Sci. 107 (25), 11223–11228. doi:10.1073/pnas.1006677107
Mitchell, J., Houston, W., Scott, R., Costes, N., Carrier, W., and Bromwell, L. (1972). Mechanical properties of lunar soil: Density, porosity, cohesion and angle of internal friction. Lunar Planet. Sci. Conf. Proc. 3, 3235.
Mori, T., Koga, M., Hikosaka, Y., Nonaka, T., Mishina, F., Sakai, Y., et al. (1991). Microbial corrosion of concrete sewer pipes, H2S production from sediments and determination of corrosion rate. Water Sci. Technol. 23 (7-9), 1275–1282. doi:10.2166/wst.1991.0579
Morrison, G. H., Gerard, J. T., Kashuba, A. T., Gangadharam, E. V., Rothenberg, A. M., Potter, N. M., et al. (1970). Multielement analysis of lunar soil and rocks. Science 167 (3918), 505–507. doi:10.1126/science.167.3918.505
Muñoz, R., Meier, L., Diaz, I., and Jeison, D. (2015). A review on the state-of-the-art of physical/chemical and biological technologies for biogas upgrading. Rev. Environ. Sci. Bio/Technology 14 (4), 727–759. doi:10.1007/s11157-015-9379-1
Naser, M. (2019). Extraterrestrial construction materials. Prog. Mater. Sci. 105, 100577. doi:10.1016/j.pmatsci.2019.100577
Nething, C., Smirnova, M., Gröning, J. A., Haase, W., Stolz, A., and Sobek, W. (2020). A method for 3D printing bio-cemented spatial structures using sand and urease active calcium carbonate powder. Mater. Des. 195, 109032. doi:10.1016/j.matdes.2020.109032
Oualha, M., Bibi, S., Sulaiman, M., and Zouari, N. (2020). Microbially induced calcite precipitation in calcareous soils by endogenous Bacillus cereus, at high pH and harsh weather. J. Environ. Manag. 257, 109965. doi:10.1016/j.jenvman.2019.109965
Papike, J., Simon, S., and Laul, J. (1982). The lunar regolith: Chemistry, mineralogy, and petrology. Rev. Geophys. 20 (4), 761–826. doi:10.1029/rg020i004p00761
Pedersen, O., Colmer, T. D., and Sand-Jensen, K. (2013). Underwater photosynthesis of submerged plants–recent advances and methods. Front. plant Sci. 4, 140. doi:10.3389/fpls.2013.00140
Pham, V. P., Nakano, A., Van Der Star, W. R., Heimovaara, T. J., and Van Paassen, L. A. (2016). Applying MICP by denitrification in soils: A process analysis. Environ. Geotech. 5 (2), 79–93. doi:10.1680/jenge.15.00078
Pickett, M. T., Roberson, L. B., Calabria, J. L., Bullard, T. J., Turner, G., and Yeh, D. H. (2020). Regenerative water purification for space applications: Needs, challenges, and technologies towards' closing the loop. Life Sci. space Res. 24, 64–82. doi:10.1016/j.lssr.2019.10.002
Qian, J., Wang, J., Yue, Z., and Wu, W. (2019). Surface crystallization behavior of calcium carbonate in the presence of SMPs secreted by SRB. J. Cryst. Growth 525, 125208. doi:10.1016/j.jcrysgro.2019.125208
Qureshi, F., Li, W., Kara, S., and Herrmann, C. (2012). “Unit process energy consumption models for material addition processes: A case of the injection molding process,” in Leveraging technology for a sustainable world (Berlin, Germany: Springer), 269–274.
Reeburgh, W. S. (2007). Oceanic methane biogeochemistry. Chem. Rev. 107 (2), 486–513. doi:10.1021/cr050362v
Rodriguez-Navarro, C., Rodriguez-Gallego, M., Ben Chekroun, K., and Gonzalez-Munoz, M. T. (2003). Conservation of ornamental stone by Myxococcus xanthus-induced carbonate biomineralization. Appl. Environ. Microbiol. 69 (4), 2182–2193. doi:10.1128/aem.69.4.2182-2193.2003
Rubanenko, L., Venkatraman, J., and Paige, D. A. (2019). Thick ice deposits in shallow simple craters on the Moon and Mercury. Nat. Geosci. 12 (8), 597–601. doi:10.1038/s41561-019-0405-8
Saricicek, Y. E., Gurbanov, R., Pekcan, O., and Gozen, A. G. (2019). Comparison of microbially induced calcium carbonate precipitation eligibility using sporosarcina pasteurii and bacillus licheniformis on two different sands. Geomicrobiol. J. 36 (1), 42–52. doi:10.1080/01490451.2018.1497732
Schlüter, L., and Cowley, A. (2020). Review of techniques for in-situ oxygen extraction on the moon. Planet. Space Sci. 181, 104753. doi:10.1016/j.pss.2019.104753
Scholle, P. A., and Ulmer-Scholle, D. (1978). “Cements and cementation,” in In Encyclopedia of sediments and sedimentary rocks (Dordrecht: Springer), 110–119.
Sharma, M., Satyam, N., and Reddy, K. R. (2021). Investigation of various gram-positive bacteria for MICP in Narmada Sand, India. Int. J. Geotechnical Eng. 15 (2), 220–234. doi:10.1080/19386362.2019.1691322
Shkuratov, Y. G., and Bondarenko, N. V. (2001). Regolith layer thickness mapping of the Moon by radar and optical data. Icarus 149 (2), 329–338. doi:10.1006/icar.2000.6545
Smith, J., and Steele, I. (1976). Lunar mineralogy; a heavenly detective story; Part II. Am. Mineralogist 61 (11-12), 1059–1116.
Šovljanski, O., Pezo, L., Stanojev, J., Bajac, B., Kovač, S., Tóth, E., et al. (2021). Comprehensive profiling of microbiologically induced CaCO3 precipitation by ureolytic Bacillus isolates from alkaline soils. Microorganisms 9 (8), 1691. doi:10.3390/microorganisms9081691
Taylor, L. A., and Liu, Y. (2010). “Important considerations for lunar soil simulants,” in Earth and space 2010: Engineering, science, construction, and operations in challenging environments (Reston, Virginia, United States: American Society of Civil Engineers), 106–118.
Taylor, L., Pieters, C., Patchen, A., Taylor, D., Morris, R., Keller, L., et al. (2003). Mineralogical characterization of lunar highland soils. Lunar Planet Sci. 34, 1774.
Van Lith, Y., Warthmann, R., Vasconcelos, C., and McKenzie, J. A. (2003). Microbial fossilization in carbonate sediments: A result of the bacterial surface involvement in dolomite precipitation. Sedimentology 50 (2), 237–245. doi:10.1046/j.1365-3091.2003.00550.x
Van Paassen, L. A., Daza, C. M., Staal, M., Sorokin, D. Y., van der Zon, W., and van Loosdrecht, M. C. (2010). Potential soil reinforcement by biological denitrification. Ecol. Eng. 36 (2), 168–175. doi:10.1016/j.ecoleng.2009.03.026
Van Tittelboom, K., De Belie, N., De Muynck, W., and Verstraete, W. (2010). Use of bacteria to repair cracks in concrete. Cem. Concr. Res. 40 (1), 157–166. doi:10.1016/j.cemconres.2009.08.025
Wang, J., Soens, H., Verstraete, W., and De Belie, N. (2014). Self-healing concrete by use of microencapsulated bacterial spores. Cem. Concr. Res. 56, 139–152. doi:10.1016/j.cemconres.2013.11.009
Wilhelm, S., and Curbach, M. (2014). Review of possible mineral materials and production techniques for a building material on the moon. Struct. Concr. 15 (3), 419–428. doi:10.1002/suco.201300088
Yoon, J.-H., Lee, K.-C., Weiss, N., Kho, Y. H., Kang, K. H., and Park, Y.-H. (2001). Sporosarcina aquimarina sp. nov., a bacterium isolated from seawater in Korea, and transfer of Bacillus globisporus (Larkin and Stokes 1967), Bacillus psychrophilus (Nakamura 1984) and Bacillus pasteurii (Chester 1898) to the genus Sporosarcina as Sporosarcina globispora comb. nov., Sporosarcina psychrophila comb. nov. and Sporosarcina pasteurii comb. nov., and emended description of th. Int. J. Syst. Evol. Microbiol. 51 (3), 1079–1086. doi:10.1099/00207713-51-3-1079
Keywords: natural geological cementation, MICP, ureolysis, metabolisms, biotechnology, moon exploration
Citation: Zuo H, Ni S and Xu M (2023) An assumption of in situ resource utilization for “bio-bricks” in space exploration. Front. Mater. 10:1155643. doi: 10.3389/fmats.2023.1155643
Received: 31 January 2023; Accepted: 20 March 2023;
Published: 06 April 2023.
Edited by:
Jinhui Tao, Pacific Northwest National Laboratory (DOE), United StatesReviewed by:
Wei Huang, Huazhong University of Science and Technology, ChinaCopyright © 2023 Zuo, Ni and Xu. This is an open-access article distributed under the terms of the Creative Commons Attribution License (CC BY). The use, distribution or reproduction in other forums is permitted, provided the original author(s) and the copyright owner(s) are credited and that the original publication in this journal is cited, in accordance with accepted academic practice. No use, distribution or reproduction is permitted which does not comply with these terms.
*Correspondence: Shuisong Ni, nis@miamioh.edu
Disclaimer: All claims expressed in this article are solely those of the authors and do not necessarily represent those of their affiliated organizations, or those of the publisher, the editors and the reviewers. Any product that may be evaluated in this article or claim that may be made by its manufacturer is not guaranteed or endorsed by the publisher.
Research integrity at Frontiers
Learn more about the work of our research integrity team to safeguard the quality of each article we publish.