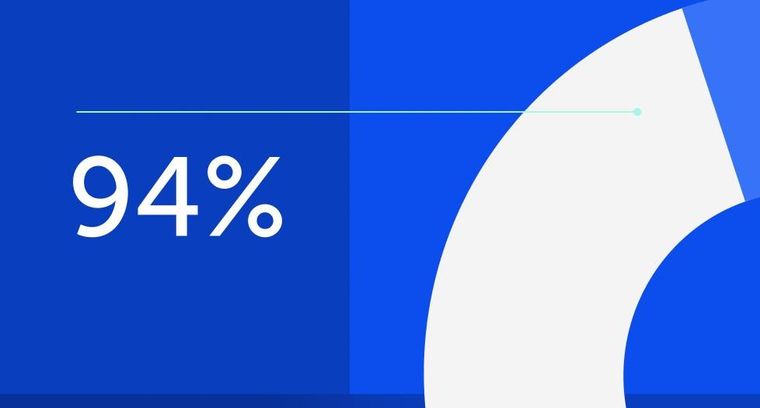
94% of researchers rate our articles as excellent or good
Learn more about the work of our research integrity team to safeguard the quality of each article we publish.
Find out more
REVIEW article
Front. Mater., 06 October 2022
Sec. Biomaterials and Bio-Inspired Materials
Volume 9 - 2022 | https://doi.org/10.3389/fmats.2022.999794
This article is part of the Research TopicAdvanced Biomaterials and 3D Printing Technologies in Bone RepairView all 23 articles
The use of titanium dental implants to replace missing teeth represents an important field of daily dental practice worldwide, which is highly reliable for long-term survival and success rates. However, titanium dental implants still have intrinsic problems that cannot meet the clinical requirements. Improving the performance of implants is an increasingly important area of dental research to reduce infection rates. Improved properties can be achieved by two main methods: 1) the overall change in the materials by changing the elemental composition and 2) surface modifications. This review provides an overview of various titanium-based alloys that have been employed to achieve a higher survival rate of implantation by adding elements or modifying the surface, with a special focus on their antibacterial applications. Recent developments in titanium-based alloys containing various antibacterial agents have been described in detail, including Cu-bearing, Ag-bearing, and Zr-bearing Ti alloys. Moreover, the applications of bioactive coatings and 3D printing materials with antibacterial properties are reviewed. This review aims to highlight the antibacterial challenges associated with titanium-based alloys to promote the further development and clinical application of antibacterial alloys.
Population ageing is a global phenomenon, and the number of people requiring fixation or total replacement of hard tissue is increasing (Lu et al., 2021b). Over the past few decades, the use of dental implants has become an indispensable part of clinical dentistry and a standard treatment procedure for dental reconstructive therapy (Alghamdi and Jansen, 2020). It is a highly successful treatment option for replacing missing teeth, with a documented long-term (>10 years) survival rate of over 90% for dental implants (Howe et al., 2019; Frisch et al., 2020).
In recent years, titanium-based alloys have been widely applied as dental implants because of their competitive properties, including excellent corrosion resistance, biocompatibility, non-magnetic properties, and non-toxicity (Liu et al., 2018; El-Bagoury et al., 2019). As a desirable material for implants, titanium-based alloys should have certain properties, such as a proper elastic modulus, antibacterial properties, and a higher osseointegration rate. A thin passive oxide layer is formed on the titanium-based implant, which makes it stable in bodily fluids and resistant to corrosion. It also facilitates osseointegration into the surrounding bone tissue (Prestat and Thierry, 2021).
However, pure titanium has no antibacterial property; therefore, bacteria tend to adhere to the neck of implants, resulting in peri-implant disease (Buser et al., 2002; Zadpoor, 2019). Infections, especially during or after implantation surgery, are serious complications for patients and cause huge pain and economic burden on them (Shimabukuro, 2020). Generally, patients need systemic antibiotic treatment to prevent infections after surgery, but the rising bacterial resistance can make existing antibiotics ineffective (Park et al., 2019). Invasive therapies used to fight infections, such as implantoplasty, might lead to other adverse outcomes (Toledano-Serrabona et al., 2021). In general, interactions between bacteria and materials can be divided into four stages. The primary step in this process is bacterial adhesion to the metal surface. The secondary step is the colonisation of bacteria on the metal surface. The third step involves biofilm formation and maturation. The final step is the proliferation of the bacteria (Zhang et al., 2021a). Many studies have reported that peri-implant infections are usually related to the formation of biofilms, which can protect bacteria from fluid shear stress and pharmacological therapies to a great extent (Norowski and Bumgardner, 2009; Quinn et al., 2020). The concentration of antibiotics at the focal site is insufficient, leading to rapid proliferation and secretion of extracellular polymers to form a biofilm after some pathogens assemble and adhere to the surface of the implant (Gristina and Costerton, 1985). Moreover, once a biofilm has formed, it will be extremely difficult to remove completely. Bacterial adhesion is also the critical stage of biofilm formation on teeth and subsequent tooth demineralization, especially the tissues around the oral treatment instruments are attached to more easily by bacteria (Fan et al., 2021). Worse still, it is difficult to clean by mechanical methods or mouthwashes. Taken together, it is of great significance to enable titanium-based implants, restorations or orthodontic archwires to be resistant to biofilm formation and to possess long-term antibacterial properties.
The agar diffusion plate test, plate-count method, live/dead stain, and dilution method can be used to assess antibacterial properties in the laboratory (Zhang et al., 2021a). According to SN/T 2399-2010, a material is considered antibacterial if the antibacterial rate is > 90%. When the antibacterial rate is less than 90%, the material has certain antibacterial properties; however, it is not an antibacterial material. When the antibacterial rate is > 99%, the material exhibits strong antibacterial properties (Lei et al., 2020). In addition, the methods commonly used to quantify the microbial biofilm or to observe the morphological changes include colony forming unit (CFU), scanning electron microscopy (SEM), confocal laser scanning microscopy (CLSM), real-time quantitative polymerase chain reaction, fluorescence in situ hybridization (FISH), etc. (Scheeren Brum et al., 2021). The methods like crystal violet assay, percentage transmission (%T) or percent transmittance (%T) and XTT reduction assay are characterized by high reliability, low-cost effectiveness and high sensitivity (Dhale et al., 2014). More recently developed methods of biofilm detection are ultrasonic Coda Wave Interferometry (Chen et al., 2021a), MgZnO dual-gate TFT biosensor (Li et al., 2020a), real-time quantitative polymerase chain reaction (Ríos-Castillo et al., 2020), Electrochemical detection (Abdelraheem et al., 2020), ect. These detection methods are more sensitive, lower consumption, more suitable for rapid detection, and the test results are more reliable and accurate.
This review focuses on various promising methods to improve the antibacterial properties of titanium dental implants as well as their relative mechanisms.
Currently, inorganic elements added to alloys as antibacterial agents mainly include antibacterial metallic elements, such as silver (Ag) and copper (Cu). Copper is an essential trace element in organisms and plays important role in human health, as well as in the maturation of the nervous, haematopoietic, bone, and other systems (Wang et al., 2021). Owing to its excellent properties in protecting the cardiovascular system, exerting antibacterial effects, and promoting bone fracture healing (Ren et al., 2015), Cu has become an important alloying element in many alloys such as Ti-Cu alloys (Borkow, 2012; Zhuang et al., 2021). Ag, due to its well-performing antibacterial and anti-biofilm formation capability, has been used as an antibacterial agent for thousands of years. Various Ag-related techniques have been introduced to modify biomedical devices for improved anti-infective properties, including doped solid coatings, nanoparticle-loaded thin films, hydrogel materials, and alloy applications (Zhang et al., 2021a). The titanium alloys containing antibacterial elements are listed in Table 1.
The antibacterial and antifungal mechanisms of Cu have been studied for a long time, involving the release of ionic copper from the surface and bacteria-metal contact. A large number of bacterial species, fungi, and viruses are vulnerable to copper, although some bacteria may be tolerant rather than resistant to copper (Grass et al., 2011; Borkow, 2012).
According to the classic view, the antimicrobial activity of Cu is based on its ability to form deleterious hydroxyl radicals via a Fenton-like mechanism. The direct production of reactive oxygen species (ROS) not only causes damages to lipids, proteins, membrane and DNA but also depletes bacterial antioxidants (Fu et al., 2014). The binding of the Cu2+ to the copper-binding site of the DNA will leads to disorders in helical structures and the denaturation of deoxyribonucleic acid (DNA) (Borkow and Gabbay, 2005). Cu impairs the function of cell membranes by peroxidizing lipids in the bilayer (Hong et al., 2012). It has also been suggested that the membrane damage caused by Cu is associated with the fact that Cu ions can interact with sh-groups and lead to their inactivation (Fu et al., 2014). The influence of Cu on proteins can come from two aspects. First, Cu is capable of inhibiting the expression of related genes of bacteria (Li et al., 2016c). And second, Cu ions may change the structure of proteins, or disrupt the enzyme structure and function by binding to the sulfur-containing or carboxylate - containing group (Sterritt and Lester, 1980). Specifically, the bacterial killing process runs as follows. First, Cu dissolves from the Cu-containing surface or material and causes cell damage. The cell membrane ruptures leading to a loss of membrane potential and cytoplasmic content. Next, the generation of ROS causes further cell damage by acting on proteins and lipids and finally genomic and plasmid DNA is degraded, leading to cell death (Grass et al., 2011). More explicit information regarding the mechanism is presented in Figure 1. Additionally, membrane depolarization is also considered to be one of the main mechanisms of Cu ions’ antimicrobial activity (Santo and Lam, 2011; Warnes et al., 2012; Fan et al., 2021). Copper ions can bind to negatively charged regions of the bacterial cell membrane (both outer and inner), reducing the potential difference and causing membrane depolarization. Membrane leakiness or even rupture can occur when the potential difference decreases to zero.
FIGURE 1. Schematic representation of the hypothetical scenario for the antibacterial mechanism of Cu2+.
Ag is also regarded as one of the most promising antibacterial agents (Ferraris and Spriano, 2016). Despite extensive research on Ag, the precise mechanism of antimicrobial effect remains to be defined. Similar to Cu, the most probable antimicrobial mechanism of silver compounds may include extensive disruption of cellular functions due to the interference with 1) cell membrane or 2) intracellular biomolecules including enzyme, protein and DNA (Yan et al., 2018), 3) the induction of oxidative stress by metal-mediated reactive oxygen species generation, culminating in associated oxidative damages (Morones-Ramirez et al., 2013). Sukumaran and Eldho described the mechanism by which silver ions prevent cell function and induce cell death by interacting with the bacterial membranes (Sukumaran and Eldho, 2012). Similarly, (Iqbal et al., 2015) proposed that silver ions interact with the proteins and enzymes of the germ membrane to cause cell damage. Upon contacting Ag ions, the zeta potential of the cell surface changes, causing cell membrane hyperpermeability, membrane depolarization and a decreased respiratory potential. At last, the disturbance of membrane integrity leads to an irreversible cell damage and consequently to cell death (Gomaa, 2017). Mocanu et al. (2014) thought that silver ions penetrate the cell membrane, bind to bacterial DNA, and inhibit the bacterial replication. Although Ag ions cannot donate or receive electrons, the electron transfer between Ag nanoparticles and the titanium substrate may cause a reactive oxidation stress (Wang et al., 2017). However, there have been some reports of bacterial resistance to the ionic silver (Losasso et al., 2014). Such resistance may involve the reduction of Ag ions to the less toxic neutral oxidation state or the active efflux of Ag ions from the cell by P-type adenosine triphosphatases or chemiosmotic Ag+/H+ antiporters (Silver et al., 2006). However, there is an inevitable problem. Ag, which is different from Cu, tends to accumulate in the human body and increase the level of serum Ag, which is harmful to human health (Masse` et al., 2000).
One of the basic requirements of novel materials used in biomedical sciences is their cytocompatibility. Cu is a highly bioactive element that exhibits strong bactericidal and cytotoxic effects (Park et al., 2013). The World Health Organization recommends that an adult should take copper 0.03 mg/kg per day and the tolerated amount is 10 mg (Filippini et al., 2018). Liu et al. (2014a) found that the antibacterial rate can be greater than 99% when the Cu immersion concentration is greater than 0.036 mg/L which is far less than the recommended daily intake. To a certain extent, Cu-bearing Ti alloys are safe.
Recently, due to the antibacterial activity, Ti-Cu alloys have attracted more attention, followed by meaningful advances. A case in point is that the hydrogen fluoride (HF) etching + anodised Ti-Cu alloy possessed strong antibacterial properties, good biological compatibility, and osteogenic ability. Among these, increasing the Cu content contributed significantly to antibacterial and osteogenic properties, and a higher and more stable antibacterial rate was obtained when the Cu content was ≥ 5% (Zhang et al., 2021b). Liu et al. (2014a) also agreed that at least 5 wt% Cu is necessary for stable antibacterial properties. However, the antibacterial properties of Ti-Cu alloys are not always positively correlated with copper content. Zhang et al. (2016a) reported that after different treatment methods, Ti-5Cu alloy exhibited a much higher antibacterial rate than Ti-10Cu alloy, which may be due to the different existing forms of Cu in the alloy. They also noted that the contact sterilisation caused by the Ti2Cu phase was the main controlling mechanism for the antibacterial capabilities of Ti-Cu alloys, and fine Ti2Cu phases with a higher surface area could result in better antibacterial ability. Many studies have obtained similar results, showing that a high-volume fraction of the Ti2Cu phase (or Cu-rich phase) improves the antibacterial ability of the Ti-xCu alloys (Fowler et al., 2019b; Lu et al., 2021a). By incrementally adding the Ti2Cu phase to alloys, some treatments may improve their antibacterial ability. The heat treatment process can change the existing form of Cu, which in turn affects the final antibacterial activity of the titanium alloy (Zhang et al., 2016a). Likewise, the ageing treatment improved the antibacterial property owing to the large amount of nano-scale Ti2Cu precipitated from the matrix. Lu et al. (2021a) prepared special Ti-Cu samples by selective acid (SAE) etching to further improve their antibacterial properties. The results showed that more Ti2Cu particles were exposed on the surface of the samples after SAE, and the antibacterial activity against Staphylococcus aureus (S. aureus) was significantly enhanced. Moreover, the morphology of the Ti2Cu phase also affected the antibacterial properties of the Ti-Cu alloy. In research conducted by Xin et al. (2022), it was found that the lamellar Ti2Cu phase (L-Ti2Cu) has a higher Cu ions release rate than the granular Ti2Cu phase (G-Ti2Cu), which is due to the formation of elongated ‘‘micro-galvanic cell” between L-Ti2Cu phase and α-Ti matrix. An increased Cu ion release rate can improve the antibacterial activity of Ti-Cu. Such a galvanic cell, in addition, will deplete the electrons in the system and even inhibit the power of protons, which is not conducive to the formation of ATP (adenosine triphosphate) in bacterial cells (Li et al., 2016a; Wang et al., 2019).
Ti-6Al-4V, which has excellent biocompatibility coupled with superior mechanical properties, accounts for over 50% of all commercially used titanium alloys (Quinn et al., 2020). Adding Cu element to Ti-6Al-4V offers a means not only to improve the mechanical properties, but also, crucially, to improve the antibacterial activity. Some research indicated that Ti-6Al-4V-5Cu behaved better wear resistance and higher hardness than Ti-6Al-4V (Wang et al., 2015). Xu et al. suggested that cell viability and corrosion resistance of Ti-6Al-4V-5Cu was better than Ti-6Al-4V. Meanwhile, this alloy showed prominent antibacterial ability. They speculated that the improvement of antibacterial property of Ti-6Al-4V-5Cu might be related to the contact sterilization via the extensive precipitation of Ti2Cu (Xu et al., 2021). In the study of Ren et al. (2014), they examined the antibacterial ability of Ti-6Al-4V- xCu (x = 1, 3, 5 wt%) and found that these alloys showed strong abilities to kill the sessile bacteria. Furthermore, with the increase of the Cu content, the antibacterial activity of the Ti-6Al-4V- xCu alloys was advanced. By using co-culture, they found Ti-6Al-4V-5Cu is capable of killing nearly all bacterial colonies (Ren et al., 2014). In addition, extra treatment and heat treatment, for example, can improve the Ti-6Al-4V-Cu antibacterial property (Macpherson et al., 2017; Peng et al., 2018). This may occur because the content, amount and size of Ti2Cu in Ti-6Al-4V- xCu increased after the heat treatment, and the greater effective contact surface area exposure brings better antibacterial ability (Xu et al., 2021).
Recently, Ti-Zr alloys have been developed for dental applications which are comparable to those of the commercially pure titanium (cp-Ti) (Niinomi, 2003). Zirconium (Zr), which is a neutral element, is a transition metal with an atomic number of 40 and an atomic weight of 91.22 amu. Zirconium belongs to Group 4 (according to the new IUPAC name) in the periodic table and is identical to titanium and hafnium. Thus, no wonder it has a similar chemical structure and properties to titanium. Additionally, Zr, similar to Ti, is biologically inert and is well accepted by the human tissue (Steinemann, 1998). Ti-Zr alloys are non-toxic and exhibit superior biomechanics compared to cp-Ti. Moreover, the use of Ti-Zr alloys can further improve the mechanical properties of implants. The hardness of the Ti-Zr alloys increased with increasing Zr content and ranged from 266 HV (Ti-10Zr) to 350 HV (Ti-40Zr) (Ho et al., 2008). Ti-45Zr has the optimum strength/elastic modulus ratio and osteogenic activity, which would be advantageous for reducing the fracture risk of implant materials (Ou et al., 2021). Due to these properties, Ti-Zr alloys are suitable for making narrow dental implants (NDIs), which are an alternative treatment when there is insufficient bone for regular implants (Gonzalez-Valls et al., 2021).
The corrosion behaviour of implant materials strongly influences foreign body reactions in the vicinity of the implantation site and thus plays a significant role in the biocompatibility of implants. Ti is well known for its high corrosion resistance (Medvedev et al., 2016). When exposed to oxygen, Ti-Zr alloy forms a stable oxide layer on its surface within nanoseconds, like titanium, which makes it a highly biocompatible and corrosion-resistant metal (Fujita, 1993). Akimoto et al. (2018) found that 90% fewer Ti ions were released from Ti-30Zr than from pure Ti after 1 week of immersion in a physiological fluid. This indicates that the ZrO2 film is more stable and resilient to dissolution as compared to the Ti oxide, and the addition of Zr can significantly reduce Ti ion dissolution, further reducing the implant-induced foreign body reaction.
Despite the excellent biocompatibility of Ti-Zr alloys, the deficiency of antibacterial activity is a disadvantage associated with Ti-Zr implants. To prevent infection and ensure high success rates in clinical applications of medical devices and implants made from Ti-Zr alloys, antimicrobial performance should be improved. Chen et al. (2020) confirmed that Ti-Zr has a lower occurrence of Candida Albicans (C. Albicans) which might be clinically advantageous for medical devices, but the antibacterial mechanism still needs to be explored. To further improve the antibacterial properties of Ti-Zr alloys, adding antibacterial elements like Cu to improve their antibacterial properties is a good choice. Kolawole et al. (2020) confirmed that adding Cu to Ti-Zr alloys did not result in a decrease in the mechanical properties. They investigated the bactericidal effect of Ti-15Zr-xCu (3 ≤ x ≤ 7, wt%) on the survival of Escherichia coli (E. coli) and S. aureus. The results show that the antibacterial ability of such alloys is as high as 98.2%; thus, their antibacterial performance has been confirmed. Lee et al. claimed that the Ti-Nb-Ta-Zr (TNTZ) system showed promising properties for biocompatibility and strength comparable to those of Ti-6Al-4V. The alloys in the range of 3–10 wt% Cu all had Ti2Cu, which may cause the potential antibacterial ability of the material (Fowler et al., 2019a). Shi et al. developed a new titanium alloy, Ti-13Nb-13Zr-5Cu (TNZ-5Cu), with a low elastic modulus and good antibacterial properties. It exhibited strong antibacterial ability (>90%) against S. aureus, which increased with the extension of the ageing duration owing to the precipitation of the Ti2Cu phase (Shi et al., 2021). It has been reported that Ti-13Nb-13Zr-10Cu has antibacterial activity against S. aureus and a low elastic modulus of 66 GPa, which might reduce bacterial infection and “stress shielding” in bone implants (Ke et al., 2019).
In the past few years, Ti-Ag alloys have become promising antibacterial candidates. Many studies have shown that Ag content is related to antibacterial properties, and a high Ag content usually results in a high antibacterial activity (Chen et al., 2017; Lei et al., 2020; Shi et al., 2020). However, too much Ag works contrary to expectations. In a study by Nakajo et al. (2014), with up to 30 wt% Ag, Ti-Ag alloys had no effect on bacteria. Ti2Ag, like Ti2Cu, plays an important role in the antibacterial ability of the Ti-Ag alloys (Chen et al., 2017). Ag ion release contributed to the antibacterial effect, while Ti2Ag particles were the key factors in contact with the germs. Surface treatments such as acid etching can further enhance the antibacterial properties of Ti-Ag alloys by exposing more Ti2Ag particles (Lei et al., 2020; Shi et al., 2020).
According to Macpherson et al., selective laser melting Ti-6Al-4V containing 0.5 wt% Ag appeared to possess little antibacterial effect, possibly due to the low alloying content (Macpherson et al., 2017). In order to achieve effective antibacterial rates (>99%), the addition of Ag should be at least 3 wt% (Chen et al., 2016).
Young’s modulus mismatch between the metallic prosthesis and human bone results in a stress shielding effect, which has been identified as a major reason for loosening or failure of the implants (Alqattan et al., 2021). Ti-Ni alloys are commonly used in orthodontics for shape memory and other applications. They display a lower Young’s modulus than human bone. In addition, the results obtained by Zheng et al. (2011) showed that the number of bacteria on Ti-Ni-Ag alloy samples was less than that in other groups. Ti-Ni-Ag alloys may provide a new solution to this modulus problem. However, some reports claim nickel is harmful (Zhao et al., 2018; Pan et al., 2020).
Ag can also be added to the Ti-Nb alloy to obtain an alloy with low modulus and better antibacterial activity. Karbowniczek et al. (2017) confirmed that Ti-Nb-1Ag showed antibacterial activity of 71.4% against E. coli. Ou et al. obtained similar results. Ti-27.5Nb alloy with 0.2–1.2 wt% Ag showed an extremely strong antibacterial effect (>90% against both S. aureus and E. coli) (Ou et al., 2017). Cai et al. (2021) designed a novel Ti-13Nb-13Zr-12.5Ag alloy in an ageing state with a low elastic modulus of 79 GPa and strong antibacterial activity. They suggested that the precipitation of Ag-rich phase is a leading cause of antibacterial properties, and high amount of the Ag-rich particle resulted in a high antibacterial ability. However, the presence of Ti2Ag phase will increases the Young’s modulus. Therefore, how to balance them is still a challenge for the develop of low modulus antibacterial titanium alloy.
Surface modification is a promising approach for improving the antibacterial properties of Ti-based alloys. This section summarises representative strategies for surface modification for antibacterial purposes (Table 2).
For dental implants, the overwhelming majority of commercial surface modifications focus on changes in the roughness of the titanium surface, primarily on improving the bone-healing process and osseointegration capability. Bacterial-surface interactions, especially the process of bacterial adhesion, may be affected by several surface properties of materials, such as roughness, surface energy, surface charge, surface wettability, and topography. In addition, other factors such as the peri-implant environment, site-specific microbiota, and host response can also be affected by bacteria-surface interactions, as illustrated in Figure 2 (Belibasakis and Manoil, 2021). Therefore, various strategies have been developed to prevent biofilm formation at an early stage by engineering the anti-adhesive surface properties.
FIGURE 2. Schematic illustration of various factors that influence bacterial adhesion. Bacterial adhesion is governed by diverse surface properties including surface roughness, charge, wettability, and topography. Other factors, such as the peri-implant environment, site-specific microbiota, and host response, can also affect bacteria-surface interactions.
The desired as-modified surface should promote osteoblast adhesion and improve osseointegration but reduce the colonisation of bacteria, which is, in part, a conflicting demand. It is generally assumed that rougher surfaces promote bacterial biofilm formation because the surface area for adhesion is larger than that of flat surfaces, thereby protecting bacteria from shear forces. However, Lorenzetti et al. (2015) have shown in their study how the microscopic roughness dramatically affects bacterial adhesion, i.e., peak-to-peak distances equal to or larger than the bacterial size that could facilitate bacterial attachment (Figure 3). This is because bacteria can adhere to the grooves and are protected from direct exposure to external forces. As a result, structures with gaps smaller than the bacterial size provided only contact points for bacterial adhesion on the surface, reducing the contact area and the number of bacteria that successfully attached to the surface.
FIGURE 3. Average fraction of adhered bacteria on Ti NT, TiA, TiA-UV, TiB, and TiB-UV samples versus. (A) Peak-to-valley height (filled symbols) and Ra roughness (open symbols) within the machined grooves in comparison with glass and polypropylene (PP) surfaces and (B) peak-to-peak distance. (C) Height versus distance profiles for Ti NT, TiA, and TiB samples. (D) Gfp-E. coli stained with Live/Dead kit on Ti NT (Lorenzetti et al., 2015).
In addition, surface energy is believed to play a key role in the adhesion process. According to general results, the surface energy increases with increasing roughness of the Ti or TiO2 panel (Rupp et al., 2006). The surface energy of approximately 42.5 mJ/m2 (1 mJ/m2 = 1 mN/m) was most effective at restricting the proliferation of bacteria. The study by Bassouset al. (2019) also supported that the optimal surface energy (42.5 mJ/m2) could reduce fibrosis, improve osteogenesis, and restrict the adhesion of bacteria in a drug-free manner to prevent infection in Ti-6Al-4V implants.
The wettability of a surface depends primarily on its surface characteristics such as surface roughness and surface energy (Song et al., 2019). Superhydrophobic or superhydrophilic surfaces has been confirmed to possess antibacterial effects by reducing protein adsorption and bacterial adhesion (Montgomerie and Popat, 2021; Tian et al., 2022). The superhydrophobic surface is defined as a surface exhibiting a static water contact angle (WCA) greater than 150°, contact angle hysteresis (CAH) below 10°, and sliding angle less than 10°(Hoshian et al., 2017). As its resistance to the adhesion of organic substances, such as blood cells and microorganisms, superhydrophobic surface has been investigated for their use in blood-contacting medical devices (Wu et al., 2021). In contrast, superhydrophilic surface is generally defined by very low WCA (less than 10°) (Si et al., 2018). Artificial superhydrophobic or superhydrophilic surfaces can be formed via different routes and techniques, such as surface treatment, changing the surface composition, or altering the surface texture. Many surface modification methods are available for the changing of surface wettability, such as MAO, ion irradiation (Karthikeyan et al., 2020; Kolanthai et al., 2022), UV light irradiation, thermal annealing or chemical treatment (Liu et al., 2014b).
Surface charge is also an important surface property that can affect bacterial adhesion and biofilm formation on surfaces. More bacterial adhesion was observed on positively charged surfaces, probably because bacteria generally possess a net negative charge owing to amino, carboxyl, and phosphate groups on their cell wall surface (Rijnaarts et al., 1999). In addition, some studies have shown that the surface charge also affects the subsequent accumulation of biofilms on the material surface (Kao et al., 2017; Shen et al., 2020).
Surface topography can significantly affect bacterial adhesion and subsequent biofilm formation. It was found that specific ranges of dimensions (i.e., height, diameter, and interspacing) of nanopillars lead to a strong bactericidal effect (He et al., 2021), and the mechanism was suggested to occur by mechanical rupture of the cell. However, Jenkins et al. (2020) reported that nanopillars did not result in mechanical rupture or cell lysis. The deformation and subsequent penetration of bacteria may be caused by the induction of oxidative stress within bacterial cells upon contact with nanopillars.
As the antibiotic resistance of microbes to drugs increases, nanotechnology provides an opportunity to resolve this problem (Li et al., 2020c). Metal nanoparticles (NPs), which are referred to as nanobiotics, have been proposed as novel antimicrobial agents. They can potentially reduce or eliminate the continuous emergence of bacterial resistance (Lee et al., 2019). Implant surfaces functionalized by coating them with NPs were found to have antibacterial properties as well as resistance to bacterial adhesion (Li et al., 2020b).
Although the exact antibacterial mechanisms of NPs remain unknown, several hypotheses have been proposed. It is generally believed that the main antibacterial mechanism is the interaction between bacteria and ions released from the NPs (Panácek et al., 2006; Mcquillan et al., 2012; Dorobantu et al., 2015). Under humid conditions, the ions released from NPs can induce excess ROS generation (Applerot et al., 2009; Wang et al., 2017; Korzekwa et al., 2021), which can kill bacteria by destroying intracellular biomolecules (Choi and Zhiqiang, 2008). The possible antibacterial mechanisms of NPs are shown in Figure 4. Although NPs do not release ions and electrons under dry conditions, some studies have demonstrated that they still possess antimicrobial properties under such conditions. This indicates that direct contact is a potential antimicrobial mechanism (Sami et al., 2018; Loran et al., 2019). This hypothesis was confirmed by Loran et al. (2019)’s experiment which showed that ion production does not increase the antibacterial effect of NPs. Moreover, the sharp edges of nanostructures can destroy microbial membranes, thus preventing biofilm formation, but are toxic to human cells as well (Pashkuleva et al., 2010).
FIGURE 4. (A) Nanoparticles internalization into the cell wall and translocation. (B) Disruption of cell wall and release of intracellular materials. (C) Variations in envelope composition of cell wall and extrusion of cytoplasm. (D) Probable mechanisms, include the following: metal ions uptake into cells, intracellular depletion, and disruption of DNA replication, release of metal ions and ROS generation, accumulation and dissolution of NPs in the bacterial membrane (Sirelkhatim et al., 2015).
The nano-scale size can be similar to the dimensions of water-filled channels in biofilm structures and possesses good affinity with a negatively charged extracellular matrix. Furthermore, their high surface-to-volume ratio also has excellent advantages (Ding et al., 2021). The metals used for these nanoparticles are almost exclusively heavy metals, such as Ag, Cu, zinc (Zn), and ZnO nanoparticles, with Ag particles being more common (Sanchez-Lopez et al., 2020). Rodríguez-Contreras et al. (2021) fabricated a coating incorporating Ag NPs and Ca on the surface of Ti using a new thermochemical treatment method. This coating not only generated non-cytotoxic surfaces and maintained the bioactivity provided by Ca, but also endowed the surfaces of Ti with antimicrobial activity against both gram-positive and gram-negative bacterial strains. Similarly, Surmeneva et al. (2019) confirmed that the calcium phosphate (CaP) layers formed on the Ti surface by plasma electrolytic oxidation (PEO) treatment comprised of approximate 0.02 mg/cm2 Ag nanoparticles, hindered E. coli and S. aureus growth. Li et al. (2016b) also proved that Ag nanoparticles with gelatin microspheres incorporated into porous titanium showed a high antibacterial effect on both E. coli and S. aureus. Usually, Ag nanoparticles are reported to kill bacteria without resistance (Sobolev et al., 2019). Unfortunately, a study (Gunawan et al., 2013) revealed resistance, resulting from the adaptation of nanosilver. In a study by Panáček et al. (2018), some gram-negative bacteria were also reported to be resistant to AgNPs under repeated contact. Panáček et al. (2015) suggested that this may be related to flagellin production, causing nanoparticle aggregation. Despite resistance without genetic changes, it cannot be handled easily. However, the pomegranate rind extract did. There is a strategy that combines NPs and antibiotics to overcome microbial resistance. The combination of Ag NPs and conventional antibiotics has been found to provide a much better antibacterial effect than Ag NPs or antibiotics alone (Naqvi et al., 2013; Hemeg, 2017). The enhanced microbial susceptibility to this combination which can facilitate the entry of antibiotics into cells may be due to the increased permeability of the microbial walls modulated by NPs. Another reason may be that the enzymes that play key roles in antibiotic resistance are inactivated by NPs (Panáček et al., 2015). Another study (Sobolev et al., 2019) found that combining up to 75% Ag NPs and 25% Zn NPs on the implant surface had antibacterial effects on both adherent and planktonic bacteria in vitro and in vivo. The addition of Zn nanoparticles can reduce Ag release and enhance the mechanical properties of the alloy.
Similar to Cu and Ag, zinc (Zn) possesses antibacterial properties. It is widely used as an antibacterial agent in dental products. The antibacterial activity of Zn was relatively weak compared to those of Ag and Cu. However, Zn ions show non-cytotoxicity in the range from 10−6 to 10−5 M. What’s more, Zn is a significant element in metabolism activity, which can promote osteogenic responses and bone formation by upregulating the pre-expression of ossification-related genes and the synthesis of extracellular matrix (Zhang et al., 2016b; Almoudi et al., 2018; Shimabukuro et al., 2019; Shimabukuro et al., 2020).
Zn ions have multiple antibacterial effects on bacteria by interacting with intact cell membranes and inhibiting relative enzyme activities (Almoudi et al., 2018). It plays a crucial role in preventing the initial adhesion of bacteria (Singh et al., 2021). ZnO is the chemical state of Zn, which functions by means of ROS generation and Zn ion release. Some researchers have suggested that ROS generation plays a leading role in the antibacterial properties of Zn (Dutta et al., 2012; Prasanna and Vijayaraghavan, 2015; Almoudi et al., 2018; Shimabukuro et al., 2019).
To prevent implant-related infections, various techniques have been introduced to confer bacteriostasis to biomaterials, such as Zn incorporation into the implant surface. The results obtained by Petrini et al. (2006) showed that Ti oxide surfaces modified with Zn could significantly reduce the viability of five streptococcal oral strains. With increasing Zn content, the bacteriostasis of the coating was enhanced, while the cytoactivity of osteoblast cells was inhibited. Therefore, Zhao et al. (2013) proposed a range of Zn contents in the micro-arc oxidation (MAO) coating (from 0.199 at % to 0.574 at%) which may be beneficial to balance cytotoxicity and antibacterial capacity. Bi et al. (2020) fabricated biphasic Zn-HA/Bi-HA coatings which incorporated Zn and bismuth (Bi) ions onto titanium plates. They found that such coatings exhibited improved antimicrobial activity against E. coli and S. aureus and that Zn2+ ions may play an important role in this antimicrobial action owing to the lower release rate of Bi3+ ions from the coatings. For the purpose of improving biological properties, several other methods exist for adding elements to the surface of implants, like plasma-based low-energy ion implanted, ion beam or coatings prepared by wet chemical precipitation method followed by microwave irradiation (Karthikeyan et al., 2019; Arul et al., 2021).
Fluorine is a necessary trace element in the human body, 93%–97% is stored in the skeletal system (Ciosek et al., 2021). Fluoride is essential for the proper development of bones and teeth. It increases the proliferation of osteoblastic cells and promotes bone formation, at the same time, inhibiting the activity of osteoclasts; thus, leading to an increase in bone mass (Liu et al., 2019). Therefore, fluorine compounds have been used in the treatment of osteoporosis. In addition, fluoride has been used in preventive dentistry for decades. They are added to mouthwash, toothpaste, and topical gels to reduce dental caries by inhibiting the demineralisation of the natural tooth tissue (Goudouri et al., 2014). Fluorine also appears in surface modifications as an antimicrobial agent (Chen et al., 2021b). Up to now, two mechanisms of have been identified for the antibacterial effect of fluorine-treated materials. So far, two mechanisms have been suggested to explain the antibacterial action of Fluorine. One such mechanism is ascribed to the fluoride ions which can act directly as an enzyme inhibitor to the glycolytic enzyme and enolase. And the formation of metal-fluoride compounds is another potential mechanism. Metal-fluoride complexes is known to inhibit proton translocating F-ATPases and disrupt the bacterial metabolism and aciduric capability (Zhou et al., 2018). Prepared by the MAO method or plasma-based deposition methods, fluorine-containing coatings have shown antibacterial abilities in many studies (Perez-Jorge et al., 2017; Zhou et al., 2018; Zhao et al., 2019). However, some studies have confirmed that titanium dioxide/calcium phosphide coatings with a high fluorine content have obvious antibacterial effects, whereas coatings with a low fluorine content lack antibacterial activity (Zhou et al., 2018).
Gallium (Ga) is a semi-metallic element in Group 13 of the periodic table. Over the past decades, Ga has shown a potential therapeutic effect on numerous disorders, such as bone loss, haemostasis, hypercalcaemia, autoimmune diseases, allograft rejection, and certain types of cancers (Kurtuldu et al., 2022). In 2003, the citrate-buffered nitrate form was approved by the United States Food and Drug Administration (FDA) for the treatment of malignant tumour-related hypercalcaemia and autoimmune diseases (Leyland-Jones, 2003). Notably, its antibacterial effects have attracted considerable attention in recent years. Ga ions (Ga3+) can be mistakenly absorbed as Fe3+ owing to their similarities, which is known as “the Trojan horse strategy” (Li et al., 2022). Iron (Fe) is an essential element in the growth process of almost all bacteria, especially those that are predisposed to infections in vivo. When Ga3+ is actively transported into bacteria, it cannot be used in any metabolic pathways and iron metabolism is disturbed, ultimately resulting in the death of the bacteria (Minandri et al., 2014). Such an antimicrobial mechanism makes it difficult for bacteria to evolve resistance by reducing the uptake of Ga, as it would reduce iron uptake as well. Furthermore, Li et al. (2022) revealed that the alkaline microenvironment and ROS produced by the samples could contribute to the antibacterial effect. Recent studies have reported that Ga exerts significant inhibitory activity against numerous bacteria, including S. aureus, E. coli, Pseudomonas aeruginosa, and Acinetobacter baumannii (Xu et al., 2017; Cochis et al., 2019). Owing to Ga3+ and Sr2+ ions, the implant with a gallium-loaded SrTiO3 nanotube coating exhibited better osteoinductivity and antibacterial properties (Qiao et al., 2019). However, a high Ga content may decrease the cytocompatibility of bone-related materials. Co-doping with cerium (Ce), which exhibits good cytocompatibility and antibacterial properties, can mitigate the cytotoxicity of Ga (Łapa et al., 2020).
Although several disadvantages have been demonstrated, antibiotics still play a crucial role in the treatment of infections. The application of antibiotics to implant surfaces is another strategy to improve their antibacterial performance. Gentamycin is commonly used for infection therapy in the orthopaedics (Sarin et al., 2019). Using the direct oxidation method in Khodaei et al. (2017)’s study, gentamicin combined with strontium-containing gelatin microspheres was synthesized and deposited on the surface of a porous titanium scaffold, which was found to postpone strontium release and enhance antibacterial ability. Zhang et al. (2020) found that 3D printed porous Ti-6Al-4V implants with vancomycin displayed a remarkable inhibition of bone infection. Furthermore, the pH-responsive release of vancomycin was proposed. However, from my perspective, in vivo, the pH did not change significantly for a long time because of homeostasis. Chlorhexidine, an antiseptic, is a common antibacterial drug that is usually used as a mouthwash. By interacting with the negatively charged bacterial membrane, chlorhexidine can become bacteriostatic or bactericidal as its concentration increases. Vidal et al. (2021) reported that samples coated with CaP and chlorhexidine which were processed by direct ink writing (DIW), showed antibacterial ability.
The emergence of Antibacterial peptides (AMPs) offers new approaches to overcoming antimicrobial resistance which is a significant challenge in the current healthcare industry (Zhang et al., 2021a). Lozeau et al. (2018) concluded from a series of studies that AMPs are short (10–50), cationic (+2 to +9), amphiphilic, and broad-spectrum antimicrobial proteins that are part of the innate immune systems of many species, including humans. It is difficult for bacteria to develop resistance owing to their antimicrobial mechanisms. Ahmed and Hammami (2019) provided a clear picture to elucidate this, as shown in Figure 5, in which the barrel-stave model, toroidal-pore model, carpet-like mode and non-membrane binding activity, receptors, and internal activity are illustrated in detail.
FIGURE 5. Overview of mechanism of action of AMPs from Ref (Ahmed and Hammami, 2019).
Photosensitizers triggered by light can act as antibacterial agents owing to their photodynamic and photothermal properties. Under light irradiation, photosensitizers such as MoSe2, MnO2, TiO2, ZnO, IR780 phosphorus, and graphene oxide can generate reactive oxygen species and/or increase local temperature, thereby killing the bacteria (Kubiak et al., 2021). For light, visible (>410 nm or 660 nm), infrared (808 nm) simulated sunlight (SSL), and LED have been found to be useful (Dutta et al., 2012; Jing et al., 2020; Teng et al., 2020; Zhu et al., 2020; Chai et al., 2021). Combined with photosensitizers, chitosan and polydopamine (PDA) are components of the coat that enhance biocompatibility and help absorb light (Teng et al., 2020; Zhu et al., 2020). Zhu et al. (2020) designed a chitosan-modified MoS2 coating with antibacterial Ag nanoparticles was incorporated. According to their results, the antibacterial rate of the coating on S. aureus and E. coli was 98.66% and 99.77%, respectively, while chitosan within the system could reduce the cytotoxicity of Ag NPs. The advantage of photodynamic antibacterial and photothermal antibacterial strategies is that they can eliminate bacterial infection on implants safely and effectively within a short time and do not cause antibiotic resistance in bacteria. However, whether they can be applied on the surface of implants requires further research because light cannot pass through hard or soft tissue, and long-time infrared irradiation is harmful.
Selective laser melting (SLM) is an additive manufacturing AM technology that can be used to manufacture metal components. So far, bone prostheses manufactured via these technologies have primarily been used clinically in relatively low load-bearing areas. The currently available 3D printing technology and new research in dentistry involve different specialities, such as oral surgery, prosthodontics, orthodontics, endodontics, periodontal disease, and temporomandibular joint rehabilitation (Christina et al., 2022). In terms of prosthodontics, 3D printing technology can be applied to interim prostheses, fixed and removable prosthodontics, etc., with the main purpose of improving accuracy and saving time and costs (Jin et al., 2018; Tahayeri et al., 2018). Surgical implant guides, dental implants, and regenerative periodontology are the primary applications of 3D printing in the periodontics (Hämmerle et al., 2009; Park et al., 2012). In addition, 3D printing has also been used to treat TMJ diseases, including making occlusal stabilisation splints (SS) for patients with temporomandibular disorders (TMDs) (Salmi et al., 2013), TMJ replacement (Zheng et al., 2019), and TMJ tissue engineering (Legemate et al., 2016). Whether used for restorations, prostheses, or implants, the material needs to have an antibacterial activity to reduce the occurrence of caries and infections of soft or hard tissues (Fan et al., 2021).
Implants made by 3D printing technology possess a larger surface area than an equally sized solid material, which increases the difficulty of sterilising the implant because of the difficult-to-reach internal surface (Zadpoor, 2019). However, to date, no clear evidence has shown an increased risk of infection in 3D printed implants (Zadpoor, 2019). Moreover, research completed by Bassous et al. (2019) corroborated that 3D printed Ti-6Al-4V scaffolds can exhibit antibacterial activity while improving osseointegration. They suggested that the probable antibacterial nature of the scaffolds might be because the concentration of adsorbed hydrophilic proteins increased with an increasing polar component of the surface energy. Some researchers have improved the antibacterial effects of 3D printed materials by adding coatings to their surfaces. Sedelnikova et al. (2022) created Zn- and Ag-containing coatings on the surface of 3D printing materials using the MAO method. It confirmed that the material has good biocompatibility and high antibacterial activity against MRSA and E. coli. In comparison to conventional methods, this method has greater design flexibility, therefore it enables the development of novel implants. Cox et al. (2016) incorporated the antibiotic (gentamicin sulphate) cement within the 3d-printed titanium-based (Ti-6Al-4V) implants containing a reservoir. After immersing implant models containing injected cement in phosphate buffered saline (PBS), they observed that gentamicin sulphate achieved a controlled release and exceed the minimum inhibitory concentrations of S. aureus (16 μg/ml) and S. epidermidis after 6 h (1 μg/ml).
Ti-based orthopaedic and dental implants have been widely used and have been successful in the past few decades. However, the major limitations of their success in clinical applications are poor osseointegration and bacterial infection, which are the two main reasons for implant failure and revision surgery. Recently, novel alloys or surface modifications designed to fight microbial infections and enhance implant survival rates have been the focus of intense biomedical research. In this review, we summarise recent progress in techniques that have been explored to modify materials with the aim of addressing issues of conventional Ti implants, especially antibacterial applications, including the addition of antibacterial elements in materials, NPs, Antibacterial peptide photosensitive coatings, and some other surface modifications. Although these techniques have proven to be effective in improving the antibacterial properties of implants, they have drawbacks. For example, for novel alloys, the entire alteration of the materials is expensive and time-consuming, which may cause changes in the mechanical properties. Numerous surface modification techniques have been investigated to optimise the interfacial properties of implants without disrupting the bulk properties of Ti biomaterials. However, in terms of coatings of Ti biomaterial, there are three main barriers to the design of these surfaces: 1) thin coatings may not adhere to the substrate in some cases, 2) long-term and stable antibacterial effects, and 3) maintenance of an effective local antibacterial effect at the implant surface without influencing the host response, and not causing cytotoxicity to the tissue surrounding the implant or allowing increased drug-resistant strains at the site. Another major setback to implant longevity is that the implant processing and surface modification methods could result in cracking and deteriorated ageing resistance of the implant surface. These drawbacks have motivated us to explore other approaches. Therefore, more suitable methods are needed to improve implant performance.
Recently, more scholars have explored solutions from a novel perspective. The future development direction of antibacterial materials should be “smart” and “synergistic”. An ideal antibacterial material should remain “bio-inert” in the absence of bacteria, activate when bacteria are present, and kill bacteria when they adhere to the surface. They should combine multiple bactericidal mechanisms to synergistically improve antibacterial efficacy and avoid resistance and should also be able to remove dead bacteria that may cause the development of a multi-structured biofilm and restore clean surfaces, thus guaranteeing long-term antibacterial properties. It is common sense that the microenvironment of biofilms is different from that of normal tissue around infection sites. Therefore, some substances can act as triggers for the response of antibacterial materials. As for stimulations, in the study of Ding et al. (2021) many products of bacteria such as enzymes, lipases, and some external stimuli such as pH, light, and microwaves have been reported. However, most of the process of experiments based on that were conducted in vitro which does not represent a real infection environment. However, the future of stimuli-responsive antibacterial materials remains bright because of their outstanding properties, functions, and irreplaceable advantages over conventional therapies.
Undoubtedly, the performance of titanium-based alloys must be further improved to adapt to inter-patient and inter-disease variations. They will be designed and tested to fight against more types of bacteria, especially gram-negative bacteria. The elastic modulus of titanium-based alloys can be reduced to resemble natural bone without damaging their properties. New-generation titanium-based alloys should be able to fight against a wide range of pathogens, have a long-lasting antibacterial effect, possess high biocompatibility and stability, and shorten the treatment time. With the advancement of technology, the cost, time, and risk will reduce dramatically. Recent developments in materials engineering, nanotechnology, and additive manufacturing approaches are expected to have a considerable impact on the medical implants industry and drive further development for the design of a new generation of intelligent and multifunctional orthopedic implants. The highly reproducibility, cost-effectiveness, stability, and durability of these novel implants will be critical factors to consider for their widespread commercialisation. Upon fabrication of these novel implants, the next critical step would be their implementation in clinical practice which requires extensive in vivo experiments and long-term performances.
ZB and ZY contributed to the study’s conception and design. YM and JY wrote the manuscript and TY and QW polished the language of the manuscript. All authors contributed to manuscript revision and read and approved the submitted version.
This work was supported by the Science and Technology Project of Liaoning Province (2018020205-101), Key Research and Development Projects of Liaoning Province (2020020184-JH2/103), and Yanglei Academician Expert Workstation of Yunnan Province (202205 AF150025).
The authors declare that the research was conducted in the absence of any commercial or financial relationships that could be construed as a potential conflict of interest.
All claims expressed in this article are solely those of the authors and do not necessarily represent those of their affiliated organizations, or those of the publisher, the editors and the reviewers. Any product that may be evaluated in this article, or claim that may be made by its manufacturer, is not guaranteed or endorsed by the publisher.
Abdelraheem, W. M., Abdelkader, A. E., Mohamed, E. S., and Mohammed, M. S. (2020). Detection of biofilm formation and assessment of biofilm genes expression in different Pseudomonas aeruginosa clinical isolates. Meta Gene 23, 100646. doi:10.1016/j.mgene.2020.100646
Aguilera-Correa, J. J., Mediero, A., Conesa-Buendia, F. M., Conde, A., Arenas, M. A., de-Damborenea, J. J., et al. (2019). Microbiological and cellular evaluation of a fluorine-phosphorus-doped titanium alloy, a novel antibacterial and osteostimulatory biomaterial with potential applications in orthopedic surgery. Appl. Environ. Microbiol. 85 (2), e02271-18. doi:10.1128/AEM.02271-18
Ahmed, T. A. E., and Hammami, R. (2019). Recent insights into structure-function relationships of antimicrobial peptides. J. Food Biochem. 43 (1), e12546. doi:10.1111/jfbc.12546
Akimoto, T., Ueno, T., Tsutsumi, Y., Doi, H., Hanawa, T., and Wakabayashi, N. (2018). Evaluation of corrosion resistance of implant-use Ti-Zr binary alloys with a range of compositions. J. Biomed. Mat. Res. 106 (1), 73–79. doi:10.1002/jbm.b.33811
Alghamdi, H. S., and Jansen, J. A. (2020). The development and future of dental implants. Dent. Mat. J. 39 (2), 167–172. doi:10.4012/dmj.2019-140
Almoudi, M. M., Hussein, A. S., Abu Hassan, M. I., and Mohamad Zain, N. (2018). A systematic review on antibacterial activity of zinc against Streptococcus mutans. Saudi Dent. J. 30 (4), 283–291. doi:10.1016/j.sdentj.2018.06.003
Alqattan, M., Peters, L., Alshammari, Y., Yang, F., and Bolzoni, L. (2021). Antibacterial Ti-Mn-Cu alloys for biomedical applications. Regen. Biomater. 8 (1), rbaa050. rbaa050. doi:10.1093/rb/rbaa050
Applerot, G., Lipovsky, A., Dror, R., Perkas, N., Nitzan, Y., Lubart, R., et al. (2009). Enhanced antibacterial activity of nanocrystalline ZnO due to increased ROS-mediated cell injury. Adv. Funct. Mat. 19 (6), 842–852. doi:10.1002/adfm.200801081
Arul, K. T., Manikandan, E., Ramya, J. R., Indira, K., Mudali, U. K., Henini, M., et al. (2021). Enhanced anticorrosion properties of nitrogen ions modified polyvinyl alcohol/Mg-Ag ions co-incorporated calcium phosphate coatings. Mater. Chem. Phys. 261, 124182. doi:10.1016/j.matchemphys.2020.124182
Bassous, N. J., Jones, C. L., and Webster, T. J. (2019). 3-D printed Ti-6Al-4V scaffolds for supporting osteoblast and restricting bacterial functions without using drugs: Predictive equations and experiments. Acta Biomater. 96, 662–673. doi:10.1016/j.actbio.2019.06.055
Belibasakis, G. N., and Manoil, D. (2021). Microbial community-driven etiopathogenesis of peri-implantitis. J. Dent. Res. 100 (1), 21–28. doi:10.1177/0022034520949851
Bi, Q., Song, X., Chen, Y., Zheng, Y., Yin, P., and Lei, T. (2020). Zn-HA/Bi-HA biphasic coatings on Titanium: Fabrication, characterization, antibacterial and biological activity. Colloids Surfaces B Biointerfaces 189, 110813. doi:10.1016/j.colsurfb.2020.110813
Borkow, G., and Gabbay, J. (2005). Copper as a biocidal tool. Curr. Med. Chem. 12 (18), 2163–2175. doi:10.2174/0929867054637617
Borkow, G. (2012). Using copper to fight microorganisms. Curr. Chem. Biol. 6 (2), 93–103. doi:10.2174/187231312801254723
Buser, D., Ingimarsson, S., Dula, K., Lussi, A., Hirt, H. P., and Belser, U. C. (2002). Long-term stability of osseointegrated implants in augmented bone: A 5-year prospective study in partially edentulous patients. Int. J. Periodontics Restor. Dent. 22 (2), 109–117.
Cai, D., Zhao, X., Yang, L., Wa Ng, R., Zhang, E., Chen, D. f., et al. (2021). A novel biomedical titanium alloy with high antibacterial property and low elastic modulus. J. Mat. Sci. Technol. 81, 13–25. doi:10.1016/j.jmst.2021.01.015
Chai, M., An, M., and Zhang, X. (2021). Construction of a TiO2/MoSe2/CHI coating on dental implants for combating Streptococcus mutans infection. Mater. Sci. Eng. C 129, 112416. doi:10.1016/j.msec.2021.112416
Chen, B., Abdallah, M., Campistron, P., Moulin, E., Callens, D., Khelissa, S. O., et al. (2021a). Detection of biofilm formation by ultrasonic Coda Wave Interferometry. J. Food Eng. 290, 110219. doi:10.1016/j.jfoodeng.2020.110219
Chen, M., Wang, X.-Q., Zhang, E.-L., Wan, Y.-Z., and Hu, J. (2021b). Antibacterial ability and biocompatibility of fluorinated titanium by plasma-based surface modification. Rare Met. 41, 689–699. doi:10.1007/s12598-021-01808-y
Chen, M., Yang, L., Zhang, L., Han, Y., Lu, Z., Qin, G., et al. (2017). Effect of nano/micro-Ag compound particles on the bio-corrosion, antibacterial properties and cell biocompatibility of Ti-Ag alloys. Mater. Sci. Eng. C 75, 906–917. doi:10.1016/j.msec.2017.02.142
Chen, M., Zhang, E., and Zhang, L. (2016). Microstructure, mechanical properties, bio-corrosion properties and antibacterial properties of Ti-Ag sintered alloys. Mater. Sci. Eng. C 62, 350–360. doi:10.1016/j.msec.2016.01.081
Chen, S., Tsoi, J. K. H., Tsang, P. C. S., Park, Y. J., Song, H. J., and Matinlinna, J. P. (2020). Candida albicans aspects of binary titanium alloys for biomedical applications. Regen. Biomater. 7 (2), 213–220. doi:10.1093/rb/rbz052
Choi, O., and Zhiqiang, H. U. (2008). Size dependent and reactive oxygen species related nanosilver toxicity to nitrifying bacteria. Environ. Sci. Technol. 42 (12), 4583–4588. doi:10.1021/es703238h
Christina, H., Maria, B., Athina, B., and Petros, K. (2022). “Chapter 9 - 3D printing in dentistry with emphasis on prosthetic rehabilitation and regenerative approaches,” in 3D printing: Applications in medicine and surgery volume 2. Editors V. N. Papadopoulos, V. Tsioukas, and J. S. Suri (Elsevier), 195–219.
Ciosek, Z., Kot, K., Kosik-Bogacka, D., Lanocha-Arendarczyk, N., and Rotter, I. (2021). The effects of calcium, magnesium, phosphorus, fluoride, and lead on bone tissue. Biomolecules 11 (4), 506. doi:10.3390/biom11040506
Cochis, A., Azzimonti, B., Chiesa, R., Rimondini, L., and Gasik, M. (2019). Metallurgical gallium additions to titanium alloys demonstrate a strong time-increasing antibacterial activity without any cellular toxicity. ACS Biomater. Sci. Eng. 5 (6), 2815–2820. doi:10.1021/acsbiomaterials.9b00147
Cox, S. C., Jamshidi, P., Eisenstein, N. M., Webber, M. A., Hassanin, H., Attallah, M. M., et al. (2016). Adding functionality with additive manufacturing: Fabrication of titanium-based antibiotic eluting implants. Mater. Sci. Eng. C 64, 407–415. doi:10.1016/j.msec.2016.04.006
Dhale, R. P., Ghorpade, M. V., and Dharmadhikari, C. A. (2014). Comparison of various methods used to detect biofilm production of Candida species. J. Clin. Diagn. Res. 8 (11), DC18–c20. doi:10.7860/jcdr/2014/10445.5147
Ding, M., Zhao, W., Song, L.-J., and Luan, S.-F. (2021). Stimuli-responsive nanocarriers for bacterial biofilm treatment. Rare Met. 4, 482–498. doi:10.1007/s12598-021-01802-4
Dorobantu, L. S., Fallone, C., Noble, A. J., Veinot, J., Burrell, R. E., Goss, G. G., et al. (2015). Toxicity of silver nanoparticles against bacteria, yeast, and algae. J. Nanopart. Res. 17 (4), 172. doi:10.1007/s11051-015-2984-7
Dutta, R. K., Nenavathu, B. P., Gangishetty, M. K., and Reddy, A. V. (2012). Studies on antibacterial activity of ZnO nanoparticles by ROS induced lipid peroxidation. Colloids Surfaces B Biointerfaces 94, 143–150. doi:10.1016/j.colsurfb.2012.01.046
El-Bagoury, N., Ahmed, S. I., Ahmed Abu Ali, O., El-Hadad, S., Fallatah, A. M., Mersal, G. A. M., et al. (2019). The influence of microstructure on the passive layer chemistry and corrosion resistance for some titanium-based alloys. Mater. (Basel) 12 (8), 1233. doi:10.3390/ma12081233
Fan, D.-Y., Yi, Z., Feng, X., Tian, W.-Z., Xu, D.-K., Cristino Valentino, A. M., et al. (2021). Antibacterial property of a gradient Cu-bearing titanium alloy by laser additive manufacturing. Rare Met. 41, 580–593. doi:10.1007/s12598-021-01826-w
Ferraris, S., and Spriano, S. (2016). Antibacterial titanium surfaces for medical implants. Mater. Sci. Eng. C 61, 965–978. doi:10.1016/j.msec.2015.12.062
Filippini, T., Cilloni, S., Malavolti, M., Violi, F., Malagoli, C., Tesauro, M., et al. (2018). Dietary intake of cadmium, chromium, copper, manganese, selenium and zinc in a Northern Italy community. J. Trace Elem. Med. Biol. 50, 508–517. doi:10.1016/j.jtemb.2018.03.001
Fowler, L., Janse Van Vuuren, A., Goosen, W., Engqvist, H., Ohman-Magi, C., and Norgren, S. (2019a). Investigation of copper alloying in a TNTZ-cux alloy. Mater. (Basel) 12 (22), 3691. doi:10.3390/ma12223691
Fowler, L., Janson, O., Engqvist, H., Norgren, S., and Ohman-Magi, C. (2019b). Antibacterial investigation of titanium-copper alloys using luminescent Staphylococcus epidermidis in a direct contact test. Mater. Sci. Eng. C 97, 707–714. doi:10.1016/j.msec.2018.12.050
Frisch, E., Wild, V., Ratka-Krüger, P., Vach, K., and Sennhenn-Kirchner, S. (2020). Long-term results of implants and implant-supported prostheses under systematic supportive implant therapy: A retrospective 25-year study. Clin. Implant Dent. Relat. Res. 22 (6), 689–696. doi:10.1111/cid.12944
Fu, Y., Chang, F. M., and Giedroc, D. P. (2014). Copper transport and trafficking at the host-bacterial pathogen interface. Acc. Chem. Res. 47 (12), 3605–3613. doi:10.1021/ar500300n
Fujita, M. (1993). In vitro study on biocompatibility of zirconium and titanium. Kokubyo Gakkai Zasshi. 60 (1), 54–65. doi:10.5357/koubyou.60.54
Gomaa, E. Z. (2017). Silver nanoparticles as an antimicrobial agent: A case study on Staphylococcus aureus and Escherichia coli as models for gram-positive and gram-negative bacteria. J. Gen. Appl. Microbiol. 63 (1), 36–43. doi:10.2323/jgam.2016.07.004
Gonzalez-Valls, G., Roca-Millan, E., Cespedes-Sanchez, J. M., Gonzalez-Navarro, B., Torrejon-Moya, A., and Lopez-Lopez, J. (2021). Narrow diameter dental implants as an alternative treatment for atrophic alveolar ridges. Systematic review and meta-analysis. Mater. (Basel) 14 (12), 3234. doi:10.3390/ma14123234
Goudouri, O. M., Kontonasaki, E., Lohbauer, U., and Boccaccini, A. R. (2014). Antibacterial properties of metal and metalloid ions in chronic periodontitis and peri-implantitis therapy. Acta Biomater. 10 (8), 3795–3810. doi:10.1016/j.actbio.2014.03.028
Grass, G., Rensing, C., and Solioz, M. (2011). Metallic copper as an antimicrobial surface. Appl. Environ. Microbiol. 77 (5), 1541–1547. doi:10.1128/AEM.02766-10
Gristina, A. G., and Costerton, J. W. (1985). Bacterial adherence to biomaterials and tissue. The significance of its role in clinical sepsis. J. Bone Jt. Surg. 67 (2), 264–273. doi:10.2106/00004623-198567020-00014
Gunawan, C., Teoh, W. Y., Marquis, C. P., and Amal, R. (2013). Induced adaptation of Bacillus sp. to antimicrobial nanosilver. Small 9 (21), 3554–3560. doi:10.1002/smll.201300761
Guo, S., Lu, Y., Wu, S., Liu, L., He, M., Zhao, C., et al. (2017). Preliminary study on the corrosion resistance, antibacterial activity and cytotoxicity of selective-laser-melted Ti6Al4V-xCu alloys. Mater. Sci. Eng. C 72, 631–640. doi:10.1016/j.msec.2016.11.126
Hämmerle, C. H., Stone, P., Jung, R. E., Kapos, T., and Brodala, N. (2009). Consensus statements and recommended clinical procedures regarding computer-assisted implant dentistry. Int. J. Oral Maxillofac. Implants 24, 126–131.
He, Z., Yang, X., Wang, N., Mu, L., Pan, J., Lan, X., et al. (2021). Anti-biofouling polymers with special surface wettability for biomedical applications. Front. Bioeng. Biotechnol. 9, 807357. doi:10.3389/fbioe.2021.807357
Hemeg, H. A. (2017). Nanomaterials for alternative antibacterial therapy. Int. J. Nanomedicine 12, 8211–8225. doi:10.2147/ijn.s132163
Ho, W. F., Chen, W. K., Wu, S. C., and Hsu, H. C. (2008). Structure, mechanical properties, and grindability of dental Ti-Zr alloys. J. Mat. Sci. Mat. Med. 19 (10), 3179–3186. doi:10.1007/s10856-008-3454-x
Hong, R., Kang, T. Y., Michels, C. A., and Gadura, N. (2012). Membrane lipid peroxidation in copper alloy-mediated contact killing of Escherichia coli. Appl. Environ. Microbiol. 78 (6), 1776–1784. doi:10.1128/aem.07068-11
Hoshian, S., Kankuri, E., Ras, R. H. A., Franssila, S., and Jokinen, V. (2017). Water and blood repellent flexible tubes. Sci. Rep. 7 (1), 16019. doi:10.1038/s41598-017-16369-3
Howe, M.-S., Keys, W., and Richards, D. (2019). Long-term (10-year) dental implant survival: A systematic review and sensitivity meta-analysis. J. Dent. 84, 9–21. doi:10.1016/j.jdent.2019.03.008
Iqbal, N., Abdul Kadir, M. R., Bin Mahmood, N. H., Iqbal, S., Almasi, D., Naghizadeh, F., et al. (2015). Characterization and biological evaluation of silver containing fluoroapatite nanoparticles prepared through microwave synthesis. Ceram. Int. 41 (5), 6470–6477. doi:10.1016/j.ceramint.2015.01.086
Jenkins, J., Mantell, J., Neal, C., Gholinia, A., Verkade, P., Nobbs, A. H., et al. (2020). Antibacterial effects of nanopillar surfaces are mediated by cell impedance, penetration and induction of oxidative stress. Nat. Commun. 11 (1), 1626. doi:10.1038/s41467-020-15471-x
Jin, S. J., Jeong, I. D., Kim, J. H., and Kim, W. C. (2018). Accuracy (trueness and precision) of dental models fabricated using additive manufacturing methods. Int. J. Comput. Dent. 21 (2), 107–113.
Jing, Z., Zhang, T., Xiu, P., Cai, H., Wei, Q., Fan, D., et al. (2020). Functionalization of 3D-printed titanium alloy orthopedic implants: A literature review. Biomed. Mat. 15 (5), 052003. doi:10.1088/1748-605X/ab9078
Kao, W. K., Gagnon, P. M., Vogel, J. P., and Chole, R. A. (2017). Surface charge modification decreases Pseudomonas aeruginosa adherence in vitro and bacterial persistence in an in vivo implant model. Laryngoscope 127 (7), 1655–1661. doi:10.1002/lary.26499
Karbowniczek, J., Cordero-Arias, L., Virtanen, S., Misra, S. K., Valsami-Jones, E., Tuchscherr, L., et al. (2017). Electrophoretic deposition of organic/inorganic composite coatings containing ZnO nanoparticles exhibiting antibacterial properties. Mater. Sci. Eng. C 77, 780–789. doi:10.1016/j.msec.2017.03.180
Karthikeyan, K. R., Arul, K. T., Ramya, J. R., Nabhiraj, P. Y., Menon, R., Krishna, J. B. M., et al. (2019). Core/shell structures on argon ions implanted polymer based zinc ions incorporated HAp nanocomposite coatings. Mater. Sci. Semicond. Process. 104, 104687. doi:10.1016/j.mssp.2019.104687
Karthikeyan, K. R., Arul, K. T., Ramya, J. R., Nabhiraj, P. Y., Menon, R., Krishna, J. B. M., et al. (2020). Novel microporous surface and blue emission of argon ion implanted polyvinylacohol/bionanohydroxyapatite coatings. Radiat. Phys. Chem. 171, 108678. doi:10.1016/j.radphyschem.2020.108678
Ke, Z., Yi, C., Zhang, L., He, Z. Y., Tan, J., and Jiang, Y. H. (2019). Characterization of a new Ti-13Nb-13Zr-10Cu alloy with enhanced antibacterial activity for biomedical applications. Mat. Lett. 253, 335–338. doi:10.1016/j.matlet.2019.07.008
Khodaei, M., Valanezhad, A., and Watanabe, I. (2017). Controlled gentamicin- strontium release as a dual action bone agent: Combination of the porous titanium scaffold and biodegradable polymers. J. Alloys Compd. 720, 22–28. doi:10.1016/j.jallcom.2017.05.236
Kolanthai, E., Ahymah Joshy, M. I., Thanigai Arul, K., Manojkumar, P., Rameshbabu, N., Ashok, M., et al. (2022). Effect of swift heavy silicon ion irradiation on TiO2 thin film prepared by micro arc oxidized technique. Mater. Today Proc. 58, 932–941. doi:10.1016/j.matpr.2021.12.312
Kolawole, S. K., Hai, W., Zhang, S., Sun, Z., Siddiqui, M. A., Ullah, I., et al. (2020). Preliminary study of microstructure, mechanical properties and corrosion resistance of antibacterial Ti-15Zr-xCu alloy for dental application. J. Mater. Sci. Technol. 50, 31–43. doi:10.1016/j.jmst.2020.03.003
Korzekwa, K., Kedziora, A., Stanczykiewicz, B., Bugla-Ploskonska, G., and Wojnicz, D. (2021). Benefits of usage of immobilized silver nanoparticles as Pseudomonas aeruginosa antibiofilm factors. Int. J. Mol. Sci. 23 (1), 284. doi:10.3390/ijms23010284
Kubiak, B., Radtke, A., Topolski, A., Wrzeszcz, G., Golinska, P., Kaszkowiak, E., et al. (2021). The composites of PCL and tetranuclear titanium(IV)-oxo complexes as materials exhibiting the photocatalytic and the antimicrobial activity. Int. J. Mol. Sci. 22 (13), 7021. doi:10.3390/ijms22137021
Kurtuldu, F., Mutlu, N., Boccaccini, A. R., and Galusek, D. (2022). Gallium containing bioactive materials: A review of anticancer, antibacterial, and osteogenic properties. Bioact. Mat. 17, 125–146. doi:10.1016/j.bioactmat.2021.12.034
Łapa, A., Cresswell, M., Campbell, I., Jackson, P., Goldmann, W. H., Detsch, R., et al. (2020). Gallium‐ and cerium‐doped phosphate glasses with antibacterial properties for medical applications. Adv. Eng. Mat. 22 (9), 1901577. doi:10.1002/adem.201901577
Lee, N. Y., Ko, W. C., and Hsueh, P. R. (2019). Nanoparticles in the treatment of infections caused by multidrug-resistant organisms. Front. Pharmacol. 10, 1153. doi:10.3389/fphar.2019.01153
Legemate, K., Tarafder, S., Jun, Y., and Lee, C. H. (2016). Engineering human TMJ discs with protein-releasing 3D-printed scaffolds. J. Dent. Res. 95 (7), 800–807. doi:10.1177/0022034516642404
Lei, Z., Zhang, H., Zhang, E., You, J., Ma, X., and Bai, X. (2020). Antibacterial activities and cell responses of Ti-Ag alloys with a hybrid micro- to nanostructured surface. J. Biomater. Appl. 34 (10), 1368–1380. doi:10.1177/0885328220905103
Leyland-Jones, B. (2003). Treatment of cancer-related hypercalcemia: The role of gallium nitrate. Semin. Oncol. 30 (5), as0093775403001714–19. doi:10.1016/s0093-7754(03)00171-4
Li, G., Wu, Y., Li, Y., Hong, Y., Zhao, X., Reyes, P. I., et al. (2020a). Early stage detection of Staphylococcus epidermidis biofilm formation using MgZnO dual-gate TFT biosensor. Biosens. Bioelectron. 151, 111993. doi:10.1016/j.bios.2019.111993
Li, K., Tian, H., Guo, A., Jin, L., Chen, W., and Tao, B. (2022). Gallium (Ga)-strontium (Sr) layered double hydroxide composite coating on titanium substrates for enhanced osteogenic and antibacterial abilities. J. Biomed. Mat. Res. A 110 (2), 273–286. doi:10.1002/jbm.a.37284
Li, M., Ma, Z., Zhu, Y., Xia, H., Yao, M., Chu, X., et al. (2016a). Toward a molecular understanding of the antibacterial mechanism of copper-bearing titanium alloys against Staphylococcus aureus. Adv. Healthc. Mat. 5 (5), 557–566. doi:10.1002/adhm.201500712
Li, M., Wang, Y., Gao, L., Sun, Y., Wang, J., Qu, S., et al. (2016b). Porous titanium scaffold surfaces modified with silver loaded gelatin microspheres and their antibacterial behavior. Surf. Coat. Technol. 286, 140–147. doi:10.1016/j.surfcoat.2015.12.006
Li, S., Liu, Y., Tian, Z., Liu, X., Han, Z., and Ren, L. (2020b). Biomimetic superhydrophobic and antibacterial stainless-steel mesh via double-potentiostatic electrodeposition and modification. Surf. Coatings Technol. 403, 126355. doi:10.1016/j.surfcoat.2020.126355
Li, Y., Liu, L., Wan, P., Zhai, Z., Mao, Z., Ouyang, Z., et al. (2016c). Biodegradable Mg-Cu alloy implants with antibacterial activity for the treatment of osteomyelitis: In vitro and in vivo evaluations. Biomaterials 106, 250–263. doi:10.1016/j.biomaterials.2016.08.031
Li, Y., Xiao, P., Wang, Y., and Hao, Y. (2020c). Mechanisms and control measures of mature biofilm resistance to antimicrobial agents in the clinical context. ACS Omega 5 (36), 22684–22690. doi:10.1021/acsomega.0c02294
Liu, J., Li, F., Liu, C., Wang, H., Ren, B., Yang, K., et al. (2014a). Effect of Cu content on the antibacterial activity of titanium-copper sintered alloys. Mater. Sci. Eng. C 35, 392–400. doi:10.1016/j.msec.2013.11.028
Liu, K., Cao, M., Fujishima, A., and Jiang, L. (2014b). Bio-inspired titanium dioxide materials with special wettability and their applications. Chem. Rev. 114 (19), 10044–10094. doi:10.1021/cr4006796
Liu, R., Tang, Y., Zeng, L., Zhao, Y., Ma, Z., Sun, Z., et al. (2018). In vitro and in vivo studies of anti-bacterial copper-bearing titanium alloy for dental application. Dent. Mat. 34 (8), 1112–1126. doi:10.1016/j.dental.2018.04.007
Liu, S., Zhou, H., Liu, H., Ji, H., Fei, W., and Luo, E. (2019). Fluorine-contained hydroxyapatite suppresses bone resorption through inhibiting osteoclasts differentiation and function in vitro and in vivo. Cell Prolif. 52 (3), e12613. doi:10.1111/cpr.12613
Loran, S., Cheng, S., Botton, G. A., Yahia, L., Yelon, A., and Sacher, E. (2019). The physicochemical characterization of the Cu nanoparticle surface, and of its evolution on atmospheric exposure: Application to antimicrobial bandages for wound dressings. Appl. Surf. Sci. 473 (APR.15), 25–30. doi:10.1016/j.apsusc.2018.12.149
Lorenzetti, M., Dogsa, I., Stosicki, T., Stopar, D., Kalin, M., Kobe, S., et al. (2015). The influence of surface modification on bacterial adhesion to titanium-based substrates. ACS Appl. Mat. Interfaces 7 (3), 1644–1651. doi:10.1021/am507148n
Losasso, C., Belluco, S., Cibin, V., Zavagnin, P., Micetic, I., Gallocchio, F., et al. (2014). Antibacterial activity of silver nanoparticles: Sensitivity of different Salmonella serovars. Front. Microbiol. 5, 227. doi:10.3389/fmicb.2014.00227
Lozeau, L. D., Rolle, M. W., and Camesano, T. A. (2018). A QCM-D study of the concentration- and time-dependent interactions of human LL37 with model mammalian lipid bilayers. Colloids Surfaces B Biointerfaces 167, 229–238. doi:10.1016/j.colsurfb.2018.04.016
Lu, M., Zhang, Z., Zhang, J., Wang, X., Qin, G., and Zhang, E. (2021a). Enhanced antibacterial activity of Ti-Cu alloy by selective acid etching. Surf. Coatings Technol. 421, 127478. doi:10.1016/j.surfcoat.2021.127478
Lu, X., Wu, Z., Xu, K., Wang, X., Wang, S., Qiu, H., et al. (2021b). Multifunctional coatings of titanium implants toward promoting osseointegration and preventing infection: Recent developments. Front. Bioeng. Biotechnol. 9, 783816. doi:10.3389/fbioe.2021.783816
Macpherson, A., Li, X., McCormick, P., Ren, L., Yang, K., and Sercombe, T. B. (2017). Antibacterial titanium produced using selective laser melting. Jom 69 (12), 2719–2724. doi:10.1007/s11837-017-2589-y
Masse`, V., Bruno, A., Bosetti, M., Biasibetti, A., Cannas, M., and Gallinaro, P. (2000). Prevention of pin track infection in external fixation with silver coated pins: Clinical and microbiological results. J. Biomed. Mat. Res. 53 (5), 600–604. doi:10.1002/1097-4636(200009)53:5<600:aid-jbm21>3.0.co;2-d
Mcquillan, J. S., Infante, H. G., Stokes, E., and Shaw, A. M. (2012). Silver nanoparticle enhanced silver ion stress response in Escherichia coli K12. Nanotoxicology 6 (8), 857–866. doi:10.3109/17435390.2011.626532
Medvedev, A. E., Molotnikov, A., Lapovok, R., Zeller, R., Berner, S., Habersetzer, P., et al. (2016). Microstructure and mechanical properties of Ti-15Zr alloy used as dental implant material. J. Mech. Behav. Biomed. Mat. 62, 384–398. doi:10.1016/j.jmbbm.2016.05.008
Minandri, F., Bonchi, C., Frangipani, E., Imperi, F., and Visca, P. (2014). Promises and failures of gallium as an antibacterial agent. Future Microbiol. 9 (3), 379–397. doi:10.2217/fmb.14.3
Mocanu, A., Furtos, G., Rapuntean, S., Horovitz, O., Flore, C., Garbo, C., et al. (2014). Synthesis; characterization and antimicrobial effects of composites based on multi-substituted hydroxyapatite and silver nanoparticles. Appl. Surf. Sci. 298, 225–235. doi:10.1016/j.apsusc.2014.01.166
Montgomerie, Z., and Popat, K. C. (2021). Improved hemocompatibility and reduced bacterial adhesion on superhydrophobic titania nanoflower surfaces. Mater. Sci. Eng. C 119, 111503. doi:10.1016/j.msec.2020.111503
Morones-Ramirez, J. R., Winkler, J. A., Spina, C. S., and Collins, J. J. (2013). Silver enhances antibiotic activity against gram-negative bacteria. Sci. Transl. Med. 5 (190), 190ra81. doi:10.1126/scitranslmed.3006276
Nakajo, K., Takahashi, M., Kikuchi, M., Takada, Y., Okuno, O., Sasaki, K., et al. (2014). Inhibitory effect of Ti-Ag alloy on artificial biofilm formation. Dent. Mat. J. 33 (3), 389–393. doi:10.4012/dmj.2013-334
Naqvi, S. Z., Kiran, U., Ali, M. I., Jamal, A., Hameed, A., Ahmed, S., et al. (2013). Combined efficacy of biologically synthesized silver nanoparticles and different antibiotics against multidrug-resistant bacteria. Int. J. Nanomedicine 8, 3187–3195. doi:10.2147/ijn.s49284
Niinomi, M. (2003). Fatigue performance and cyto-toxicity of low rigidity titanium alloy, Ti-29Nb-13Ta-4.6Zr. Biomaterials 24 (16), 2673–2683. doi:10.1016/s0142-9612(03)00069-3
Norowski, P. A., and Bumgardner, J. D. (2009). Biomaterial and antibiotic strategies for peri-implantitis: A review. J. Biomed. Mat. Res. 88 (2), 530–543. doi:10.1002/jbm.b.31152
Ou, K.-L., Weng, C.-C., Lin, Y.-H., and Huang, M.-S. (2017). A promising of alloying modified beta-type Titanium-Niobium implant for biomedical applications: Microstructural characteristics, in vitro biocompatibility and antibacterial performance. J. Alloys Compd. 697, 231–238. doi:10.1016/j.jallcom.2016.12.120
Ou, P., Hao, C., Liu, J., He, R., Wang, B., and Ruan, J. (2021). Cytocompatibility of Ti-xZr alloys as dental implant materials. J. Mat. Sci. Mat. Med. 32 (5), 50. doi:10.1007/s10856-021-06522-w
Pan, Y., Lin, Y., Jiang, L., Lin, H., Xu, C., Lin, D., et al. (2020). Removal of dental alloys and titanium attenuates trace metals and biological effects on liver and kidney. Chemosphere 243, 125205. doi:10.1016/j.chemosphere.2019.125205
Panácek, A., Kvítek, L., Prucek, R., Pizurova, N., and Pizúrová, N. d. (2006). Kolá?, M., Vec?e?ováSilver colloid nanoparticles: Synthesis, characterization, and their antibacterial activity. J. Phys. Chem. B 110 (33), 16248–16253. doi:10.1021/jp063826h
Panáček, A., Kvitek, L., Smekalova, M., Vecerova, R., Kolar, M., Roderova, M., et al. (2018). Bacterial resistance to silver nanoparticles and how to overcome it. Nat. Nanotechnol. 13 (1), 65–71. doi:10.1038/s41565-017-0013-y
Panáček, A., Smékalová, M., Kilianová, M., Prucek, R., Bogdanová, K., Večeřová, R., et al. (2015). Strong and nonspecific synergistic antibacterial efficiency of antibiotics combined with silver nanoparticles at very low concentrations showing No cytotoxic effect. Molecules 21 (1), E26. doi:10.3390/molecules21010026
Park, C. H., Rios, H. F., Jin, Q., Sugai, J. V., Padial-Molina, M., Taut, A. D., et al. (2012). Tissue engineering bone-ligament complexes using fiber-guiding scaffolds. Biomaterials 33 (1), 137–145. doi:10.1016/j.biomaterials.2011.09.057
Park, C., Seong, Y. J., Kang, I. G., Song, E. H., Lee, H., Kim, J., et al. (2019). Enhanced osseointegration ability of poly(lactic acid) via tantalum sputtering-based plasma immersion ion implantation. ACS Appl. Mat. Interfaces 11 (11), 10492–10504. doi:10.1021/acsami.8b21363
Park, Y. J., Song, Y. H., An, J. H., Song, H. J., and Anusavice, K. J. (2013). Cytocompatibility of pure metals and experimental binary titanium alloys for implant materials. J. Dent. (Shiraz). 41 (12), 1251–1258. doi:10.1016/j.jdent.2013.09.003
Pashkuleva, I., Marques, A. P., Vaz, F., and Rui, L. R. (2010). Surface modification of starch based biomaterials by oxygen plasma or UV-irradiation. J. Mat. Sci. Mat. Med. 21 (1), 21–32. doi:10.1007/s10856-009-3831-0
Peng, C., Zhang, S., Sun, Z., Ren, L., and Yang, K. (2018). Effect of annealing temperature on mechanical and antibacterial properties of Cu-bearing titanium alloy and its preliminary study of antibacterial mechanism. Mater. Sci. Eng. C 93, 495–504. doi:10.1016/j.msec.2018.08.018
Perez-Jorge, C., Arenas, M. A., Conde, A., Hernandez-Lopez, J. M., de Damborenea, J. J., Fisher, S., et al. (2017). Bacterial and fungal biofilm formation on anodized titanium alloys with fluorine. J. Mat. Sci. Mat. Med. 28 (1), 8. doi:10.1007/s10856-016-5811-5
Petrini, P., Arciola, C. R., Pezzali, I., Bozzini, S., Montanaro, L., Tanzi, M. C., et al. (2006). Antibacterial activity of zinc modified titanium oxide surface. Int. J. Artif. Organs 29 (4), 434–442. doi:10.1177/039139880602900414
Prasanna, V. L., and Vijayaraghavan, R. (2015). Insight into the mechanism of antibacterial activity of ZnO – surface defects mediated reactive oxygen species even in Dark+. Langmuir 31 (33), 9155–9162.
Prestat, M., and Thierry, D. (2021). Corrosion of titanium under simulated inflammation conditions: Clinical context and in vitro investigations. Acta Biomater. 136, 72–87. doi:10.1016/j.actbio.2021.10.002
Qiao, H., Zhang, C., Dang, X., Yang, H., Huang, Y., Chen, Y., et al. (2019). Gallium loading into a polydopamine-functionalised SrTiO3 nanotube with combined osteoinductive and antimicrobial activities. Ceram. Int. 45 (17), 22183–22195. doi:10.1016/j.ceramint.2019.07.240
Quinn, J., McFadden, R., Chan, C. W., and Carson, L. (2020). Titanium for orthopedic applications: An overview of surface modification to improve biocompatibility and prevent bacterial biofilm formation. iScience 23 (11), 101745. doi:10.1016/j.isci.2020.101745
Ren, L., Ma, Z., Li, M., Zhang, Y., Liu, W., Liao, Z., et al. (2014). Antibacterial properties of Ti–6Al–4V–xCu alloys. J. Mat. Sci. Technol. 30 (7), 699–705. doi:10.1016/j.jmst.2013.12.014
Ren, L., Wong, H., Yan, C., Yeung, K., and Yang, K. (2015). Osteogenic ability of Cu-bearing stainless steel. J. Biomed. Mat. Res. 103 (7), 1433–1444. doi:10.1002/jbm.b.33318
Rijnaarts, H. H. M., Norde, W., Lyklema, J., and Zehnder, A. J. B. (1999). DLVO and steric contributions to bacterial deposition in media of different ionic strengths. Colloids Surfaces B Biointerfaces 14 (1), 179–195. doi:10.1016/S0927-7765(99)00035-1
Ríos-Castillo, A. G., Ripolles-Avila, C., and Rodríguez-Jerez, J. J. (2020). Detection of Salmonella Typhimurium and Listeria monocytogenes biofilm cells exposed to different drying and pre-enrichment times using conventional and rapid methods. Int. J. Food Microbiol. 324, 108611. doi:10.1016/j.ijfoodmicro.2020.108611
Rodríguez-Contreras, A., Torres, D., Rafik, B., Ortiz-Hernandez, M., Ginebra, M. P., Calero, J. A., et al. (2021). Bioactivity and antibacterial properties of calcium- and silver-doped coatings on 3D printed titanium scaffolds. Surf. Coatings Technol. 421, 127476. doi:10.1016/j.surfcoat.2021.127476
Rupp, F., Scheideler, L., Olshanska, N., de Wild, M., Wieland, M., and Geis-Gerstorfer, J. (2006). Enhancing surface free energy and hydrophilicity through chemical modification of microstructured titanium implant surfaces. J. Biomed. Mat. Res. A 76 (2), 323–334. doi:10.1002/jbm.a.30518
Salmi, M., Paloheimo, K. S., Tuomi, J., Ingman, T., and Mäkitie, A. (2013). A digital process for additive manufacturing of occlusal splints: A clinical pilot study. J. R. Soc. Interface 10 (84), 20130203. doi:10.1098/rsif.2013.0203
Sami, R., Dionysiou, D. D., Pillai, S. C., and John, K. (2018). Advances in catalytic/photocatalytic bacterial inactivation by nano Ag and Cu coated surfaces and medical devices. Appl. Catal. B Environ. 240, 291–318. doi:10.1016/j.apcatb.2018.07.025
Sanchez-Lopez, E., Gomes, D., Esteruelas, G., Bonilla, L., Lopez-Machado, A. L., Galindo, R., et al. (2020). Metal-based nanoparticles as antimicrobial agents: An overview. Nanomater. (Basel) 10 (2), 292. doi:10.3390/nano10020292
Santo, C., E., Lam, E., W., Elowsky, C. G., Quaranta, D., Domaille, D. W., Chang, C. J., et al. (2011). Bacterial killing by dry metallic copper surfaces. Appl. Environ. Microbiol. 77, 794–802. doi:10.1128/AEM.01599-10
Sarin, N., Singh, K. J., Kaur, H., Kaur, R., and Singh, J. (2019). Preliminary studies of the effect of doping of chromium oxide in SiO2-CaO-P2O5 bioceramics for bone regeneration applications. Spectrochimica Acta Part A Mol. Biomol. Spectrosc. 229, 118000. doi:10.1016/j.saa.2019.118000
Scheeren Brum, R., Apaza-Bedoya, K., Labes, L. G., Volpato, C. A. M., Pimenta, A. L., and Benfatti, C. A. M. (2021). Early biofilm formation on rough and smooth titanium specimens: A systematic review of clinical studies. J. Oral Maxillofac. Res. 12 (4), e1. doi:10.5037/jomr.2021.12401
Sedelnikova, M. B., Sharkeev, Y. P., Tolkacheva, T. V., Uvarkin, P. V., Chebodaeva, V. V., Prosolov, K. A., et al. (2022). Additively manufactured porous titanium 3D–scaffolds with antibacterial Zn-Ag- calcium phosphate biocoatings. Mater. Charact. 186, 111782. doi:10.1016/j.matchar.2022.111782
Shen, J., Gao, P., Han, S., Kao, R. Y. T., Wu, S., Liu, X., et al. (2020). A tailored positively-charged hydrophobic surface reduces the risk of implant associated infections. Acta Biomater. 114, 421–430. doi:10.1016/j.actbio.2020.07.040
Shen, X., Al-Baadani, M. A., He, H., Cai, L., Wu, Z., Yao, L., et al. (2019). Antibacterial and osteogenesis performances of LL37-loaded titania nanopores in vitro and in vivo. Int. J. Nanomedicine 14, 3043–3054. doi:10.2147/IJN.S198583
Shi, A., Cai, D., Hu, J., Zhao, X., Qin, G., Han, Y., et al. (2021). Development of a low elastic modulus and antibacterial Ti-13Nb-13Zr-5Cu titanium alloy by microstructure controlling. Mater. Sci. Eng. C 126, 112116. doi:10.1016/j.msec.2021.112116
Shi, A., Zhu, C., Fu, S., Wang, R., Qin, G., Chen, D., et al. (2020). What controls the antibacterial activity of Ti-Ag alloy, Ag ion or Ti2Ag particles? Mater. Sci. Eng. C 109, 110548. doi:10.1016/j.msec.2019.110548
Shimabukuro, M. (2020). Antibacterial property and biocompatibility of silver, copper, and zinc in titanium dioxide layers incorporated by one-step micro-arc oxidation: A review. Antibiot. (Basel) 9 (10), 716. doi:10.3390/antibiotics9100716
Shimabukuro, M., Hiji, A., Manaka, T., Nozaki, K., Chen, P., Ashida, M., et al. (2020). Time-transient effects of silver and copper in the porous titanium dioxide layer on antibacterial properties. J. Funct. Biomater. 11 (2), 44. doi:10.3390/jfb11020044
Shimabukuro, M., Tsutsumi, Y., Nozaki, K., Chen, P., Yamada, R., Ashida, M., et al. (2019). Chemical and biological roles of zinc in a porous titanium dioxide layer formed by micro-arc oxidation. Coatings 9 (11), 705. doi:10.3390/coatings9110705
Si, Y., Dong, Z., and Jiang, L. (2018). Bioinspired designs of superhydrophobic and superhydrophilic materials. ACS Cent. Sci. 4 (9), 1102–1112. doi:10.1021/acscentsci.8b00504
Silver, S., Phung le, T., and Silver, G. (2006). Silver as biocides in burn and wound dressings and bacterial resistance to silver compounds. J. Ind. Microbiol. Biotechnol. 33 (7), 627–634. doi:10.1007/s10295-006-0139-7
Singh, T. A., Sharma, A., Tejwan, N., Ghosh, N., Das, J., and Sil, P. C. (2021). A state of the art review on the synthesis, antibacterial, antioxidant, antidiabetic and tissue regeneration activities of zinc oxide nanoparticles. Adv. Colloid Interface Sci. 295, 102495. doi:10.1016/j.cis.2021.102495
Sirelkhatim, A., Mahmud, S., Seeni, A., Kaus, N. H. M., Ann, L. C., Bakhori, S. K. M., et al. (2015). Review on zinc oxide nanoparticles: Antibacterial activity and toxicity mechanism. Nanomicro. Lett. 7 (3), 219–242. doi:10.1007/s40820-015-0040-x
Sobolev, A., Valkov, A., Kossenko, A., Wolicki, I., Zinigrad, M., and Borodianskiy, K. (2019). Bioactive coating on Ti alloy with high osseointegration and antibacterial Ag nanoparticles. ACS Appl. Mat. Interfaces 11 (43), 39534–39544. doi:10.1021/acsami.9b13849
Song, K., Lee, J., Choi, S. O., and Kim, J. (2019). Interaction of surface energy components between solid and liquid on wettability, and its application to textile anti-wetting finish. Polym. (Basel) 11 (3), 498. doi:10.3390/polym11030498
Steinemann, S. G. (1998). Titanium-the material of choice? Periodontol. 2000 17, 7–21. doi:10.1111/j.1600-0757.1998.tb00119.x
Sterritt, R. M., and Lester, J. N. (1980). Interactions of heavy metals with bacteria. Sci. Total Environ. 14 (1), 5–17. doi:10.1016/0048-9697(80)90122-9
Sukumaran, P., and Eldho, K. P. (2012). Silver nanoparticles: Mechanism of antimicrobial action, synthesis, medical applications, and toxicity effects. Int. Nano Lett. 2 (1), 32. doi:10.1186/2228-5326-2-32
Surmeneva, M., Lapanje, A., Chudinova, E., Ivanova, A., Koptyug, A., Loza, K., et al. (2019). Decreased bacterial colonization of additively manufactured Ti6Al4V metallic scaffolds with immobilized silver and calcium phosphate nanoparticles. Appl. Surf. Sci. 480, 822–829. doi:10.1016/j.apsusc.2019.03.003
Tahayeri, A., Morgan, M., Fugolin, A. P., Bompolaki, D., Athirasala, A., Pfeifer, C. S., et al. (2018). 3D printed versus conventionally cured provisional crown and bridge dental materials. Dent. Mat. 34 (2), 192–200. doi:10.1016/j.dental.2017.10.003
Teng, X., Liu, X., Cui, Z., Zheng, Y., Chen, D.-f., Li, Z., et al. (2020). Rapid and highly effective bacteria-killing by polydopamine/IR780@MnO2–Ti using near-infrared light. Prog. Nat. Sci. Mater. Int. 30 (5), 677–685. doi:10.1016/j.pnsc.2020.06.003
Tian, M., Cai, S., Ling, L., Zuo, Y., Wang, Z., Liu, P., et al. (2022). Superhydrophilic hydroxyapatite/hydroxypropyltrimethyl ammonium chloride chitosan composite coating for enhancing the antibacterial and corrosion resistance of magnesium alloy. Prog. Org. Coatings 165, 106745. doi:10.1016/j.porgcoat.2022.106745
Toledano-Serrabona, J., Gil, F. J., Camps-Font, O., Valmaseda-Castellón, E., Gay-Escoda, C., and Sánchez-Garcés, M. Á. (2021). Physicochemical and biological characterization of Ti6Al4V particles obtained by implantoplasty: An in vitro study. Part I. Materials 14 (21), 6507. doi:10.3390/ma14216507
Vidal, E., Guillem-Marti, J., Ginebra, M.-P., Combes, C., Rupérez, E., and Rodriguez, D. (2021). Multifunctional homogeneous calcium phosphate coatings: Toward antibacterial and cell adhesive titanium scaffolds. Surf. Coatings Technol. 405, 126557. doi:10.1016/j.surfcoat.2020.126557
Wang, G., Jin, W., Qasim, A. M., Gao, A., Peng, X., Li, W., et al. (2017). Antibacterial effects of titanium embedded with silver nanoparticles based on electron-transfer-induced reactive oxygen species. Biomaterials 124, 25–34. doi:10.1016/j.biomaterials.2017.01.028
Wang, J., Zhang, S., Sun, Z., Wang, H., Ren, L., and Yang, K. (2019). Optimization of mechanical property, antibacterial property and corrosion resistance of Ti-Cu alloy for dental implant. J. Mater. Sci. Technol. 35 (10), 2336–2344. doi:10.1016/j.jmst.2019.03.044
Wang, P., Yuan, Y., Xu, K., Zhong, H., Yang, Y., Jin, S., et al. (2021). Biological applications of copper-containing materials. Bioact. Mat. 6 (4), 916–927. doi:10.1016/j.bioactmat.2020.09.017
Wang, S., Ma, Z., Liao, Z., Song, J., Yang, K., and Liu, W. (2015). Study on improved tribological properties by alloying copper to CP-Ti and Ti-6Al-4V alloy. Mater. Sci. Eng. C 57, 123–132. doi:10.1016/j.msec.2015.07.046
Warnes, S. L., Caves, V., and Keevil, C. W. (2012). Mechanism of copper surface toxicity in Escherichia coli O157:H7 and Salmonella involves immediate membrane depolarization followed by slower rate of DNA destruction which differs from that observed for Gram‐positive bacteria. Environ. Microbiol. 14 (7), 1730–1743. doi:10.1111/j.1462-2920.2011.02677.x
Wu, X. H., Liew, Y. K., Mai, C. W., and Then, Y. Y. (2021). Potential of superhydrophobic surface for blood-contacting medical devices. Int. J. Mol. Sci. 22 (7), 3341. doi:10.3390/ijms22073341
Xin, C., Wang, N., Chen, Y., He, B., Zhao, Q., Chen, L., et al. (2022). Biological corrosion behaviour and antibacterial properties of Ti-Cu alloy with different Ti2Cu morphologies for dental applications. Mater. Des. 215, 110540. doi:10.1016/j.matdes.2022.110540
Xu, D., Wang, T., Lu, Z., Wang, Y., Sun, B., Wang, S., et al. (2021). Ti-6Al-4V-5Cu synthesized for antibacterial effect in vitro and in vivo via contact sterilization. J. Mater. Sci. Technol. 90, 133–142. doi:10.1016/j.jmst.2021.03.007
Xu, Z., Zhao, X., Chen, X., Chen, Z., and Xia, Z. (2017). Antimicrobial effect of gallium nitrate against bacteria encountered in burn wound infections. RSC Adv. 7 (82), 52266–52273. doi:10.1039/c7ra10265h
Yan, X., He, B., Liu, L., Qu, G., Shi, J., Hu, L., et al. (2018). Antibacterial mechanism of silver nanoparticles in Pseudomonas aeruginosa: Proteomics approach. Metallomics 10 (4), 557–564. doi:10.1039/c7mt00328e
Zadpoor, A. A. (2019). Additively manufactured porous metallic biomaterials. J. Mat. Chem. B 7 (26), 4088–4117. doi:10.1039/c9tb00420c
Zhang, E., Wang, X., Chen, M., and Hou, B. (2016a). Effect of the existing form of Cu element on the mechanical properties, bio-corrosion and antibacterial properties of Ti-Cu alloys for biomedical application. Mater. Sci. Eng. C 69, 1210–1221. doi:10.1016/j.msec.2016.08.033
Zhang, E., Zhao, X., Hu, J., Wang, R., Fu, S., and Qin, G. (2021a). Antibacterial metals and alloys for potential biomedical implants. Bioact. Mat. 6 (8), 2569–2612. doi:10.1016/j.bioactmat.2021.01.030
Zhang, L., Gao, Q., and Han, Y. (2016b). Zn and Ag Co-doped anti-microbial TiO2 coatings on Ti by micro-arc oxidation. J. Mat. Sci. Technol. 32 (9), 919–924. doi:10.1016/j.jmst.2016.01.008
Zhang, T., Wei, Q., Zhou, H., Zhou, W., Fan, D., Lin, X., et al. (2020). Sustainable release of vancomycin from micro-arc oxidised 3D-printed porous Ti6Al4V for treating methicillin-resistant Staphylococcus aureus bone infection and enhancing osteogenesis in a rabbit tibia osteomyelitis model. Biomater. Sci. 8 (11), 3106–3115. doi:10.1039/c9bm01968e
Zhang, W., Zhang, S., Liu, H., Ren, L., Wang, Q., and Zhang, Y. (2021b). Effects of surface roughening on antibacterial and osteogenic properties of Ti-Cu alloys with different Cu contents. J. Mat. Sci. Technol. 88, 158–167. doi:10.1016/j.jmst.2021.01.067
Zhao, B. H., Zhang, W., Wang, D. N., Feng, W., Liu, Y., Lin, Z., et al. (2013). Effect of Zn content on cytoactivity and bacteriostasis of micro-arc oxidation coatings on pure titanium. Surf. Coatings Technol. 228, S428–S432. doi:10.1016/j.surfcoat.2012.05.037
Zhao, L., Si, J., Wei, Y., Li, S., Jiang, Y., Zhou, R., et al. (2018). Toxicity of porcelain-fused-to-metal substrate to zebrafish (Danio rerio) embryos and larvae. Life Sci. 203, 66–71. doi:10.1016/j.lfs.2018.04.019
Zhao, Q., Yi, L., Hu, A., Jiang, L., Hong, L., and Dong, J. (2019). Antibacterial and osteogenic activity of a multifunctional microporous coating codoped with Mg, Cu and F on titanium. J. Mat. Chem. B 7 (14), 2284–2299. doi:10.1039/c8tb03377c
Zheng, J., Chen, X., Jiang, W., Zhang, S., Chen, M., and Yang, C. (2019). An innovative total temporomandibular joint prosthesis with customized design and 3D printing additive fabrication: A prospective clinical study. J. Transl. Med. 17 (1), 4. doi:10.1186/s12967-018-1759-1
Zheng, Y. F., Zhang, B. B., Wang, B. L., Wang, Y. B., Li, L., Yang, Q. B., et al. (2011). Introduction of antibacterial function into biomedical TiNi shape memory alloy by the addition of element Ag. Acta Biomater. 7 (6), 2758–2767. doi:10.1016/j.actbio.2011.02.010
Zhou, J., Li, B., and Han, Y. (2018). F-doped TiO2 microporous coating on titanium with enhanced antibacterial and osteogenic activities. Sci. Rep. 8 (1), 17858. doi:10.1038/s41598-018-35875-6
Zhu, M., Liu, X., Tan, L., Cui, Z., Liang, Y., Li, Z., et al. (2020). Photo-responsive chitosan/Ag/MoS2 for rapid bacteria-killing. J. Hazard. Mat. 383, 121122. doi:10.1016/j.jhazmat.2019.121122
Keywords: titanium, implants, infection, antibacterial properties, surface treatments, 3D-printed
Citation: Ma Y, Yan J, Yan T, Wang Q, Bao Z and Yi Z (2022) Biological properties of Cu-bearing and Ag-bearing titanium-based alloys and their surface modifications: A review of antibacterial aspect. Front. Mater. 9:999794. doi: 10.3389/fmats.2022.999794
Received: 21 July 2022; Accepted: 20 September 2022;
Published: 06 October 2022.
Edited by:
Yanjin Lu, Fujian Institute of Research on the Structure of Matter (CAS), ChinaCopyright © 2022 Ma, Yan, Yan, Wang, Bao and Yi. This is an open-access article distributed under the terms of the Creative Commons Attribution License (CC BY). The use, distribution or reproduction in other forums is permitted, provided the original author(s) and the copyright owner(s) are credited and that the original publication in this journal is cited, in accordance with accepted academic practice. No use, distribution or reproduction is permitted which does not comply with these terms.
*Correspondence: Zhifan Bao, emZiYW9AY211LmVkdS5jbg==; Zhe Yi, emhleWlAY211LmVkdS5jbg==
Disclaimer: All claims expressed in this article are solely those of the authors and do not necessarily represent those of their affiliated organizations, or those of the publisher, the editors and the reviewers. Any product that may be evaluated in this article or claim that may be made by its manufacturer is not guaranteed or endorsed by the publisher.
Research integrity at Frontiers
Learn more about the work of our research integrity team to safeguard the quality of each article we publish.