- 1Department of Trauma and Joint Surgery, Shunde Hospital, Southern Medical University, Foshan, Guangdong, China
- 2Department of Advanced Oral Sciences and Therapeutics, University of Maryland Dental School, Baltimore, MD, United States
- 3Department of Orthopaedic Surgery, Nanfang Hospital, Southern Medical University, Guangzhou, Guangdong, China
- 4Key Laboratory of Shannxi Province for Craniofacial Precision Medicine Research, College of Stomatology, Xi’an Jiaotong University, Xi’an, Shannxi, China
- 5Department of Oncology and Diagnostic Sciences, University of Maryland School of Dentistry, Baltimore, United States
- 6Maurice H. Kornberg School of Dentistry, Temple University, Philadelphia, PA, United States
- 7Member, Marlene and Stewart Greenebaum Cancer Center, University of Maryland School of Medicine, Baltimore, MD, United States
- 8Jiangsu Key Laboratory of Oral Diseases, Nanjing Medical University, Nanjing, China
- 9Jiangsu Key Laboratory for Biomaterials and Devices, School of Biological Science and Medical Engineering, Southeast University, Nanjing, China
- 10Center for Stem Cell Biology and Regenerative Medicine, University of Maryland School of Medicine, Baltimore, MD, United States
Objectives: 1) Develop a novel construct of human periodontal ligament stem cells (hPDLSCs) encapsulated in degradable alginate microbeads (DAMB) with human platelet lysate (hPL) and injectable calcium phosphate cement (ICPC); 2) Investigate the proliferation and osteogenic differentiation of hPDLSCs in ICPC with hPL as a xeno-free supplement and animal serum replacement for bone tissue engineering applications.
Methods: hPDLSCs were encapsulated in alginate-fibrin microbeads (DAMB + fibrin), alginate-hPL degradable microbeads (DAMB + hPL), or alginate-fibrin-hPL microbeads (DAMB + fibrin + hPL). The proliferation and osteogenic differentiation of hPDLSCs were investigated in culturing with the ICPC scaffold.
Results: Flexural strength of ICPC was 8.4 ± 0.91 MPa, and elastic modulus was 1.56 ± 0.1 GPa, exceeding those of cancellous bone. hPDLSCs had higher viability in DAMB + fibrin + hPL group than in DAMB + fibrin. ALP was 69.97 ± 16.96 mU/mg for ICPC + DAMB + fibrin + hPL group, higher than 30.68 ± 2.86 mU/mg of ICPC + DAMB + fibrin (p < 0.05) and 4.12 ± 1.65 mU/mg of control (p < 0.01). At 7 days, osteogenic gene expressions (ALP, RUNX2, COL1, and OPN) in ICPC + DAMB + fibrin + hPL and ICPC + DAMB + fibrin were 4–11 folds that of control. At 21 days, the hPDLSC-synthesized bone mineral amounts in ICPC + DAMB + fibrin + hPL and ICPC + DAMB + fibrin were 13.2 folds and 11.1 folds that of control group, respectively.
Conclusion: The novel injectable CPC scaffold encapsulating hPDLSCs and hPL is promising to protect and deliver hPDLSCs. The hPL-based medium significantly enhanced the osteogenic differentiation of hPDLSCs in ICPC + DAMB + fibrin + hPL construct, suggesting a promising xeno-free approach for bone tissue regeneration applications.
Introduction
Bone tissue engineering involves the use of biomaterials, cells and bioactive factors and represents a promising approach for bone regeneration. Injectable calcium phosphate cement (ICPC) is used for bone repair due to their similarity to bone minerals (Szpalski et al., 2012a; Szpalski et al., 2012b; Wang P. et al., 2014). The first ICPC was developed in 1986 and consisted of a mixture of tetracalcium phosphate [TTCP, Ca4 (PO4)2O] and dicalcium phosphate anhydrous (DCPA, CaHPO4). ICPC was approved by the Food and Drug Administration (FDA) for the repair of craniofacial defects due to its excellent biocompatibility, osteoconductivity, resorbability, and injectability (Friedman et al., 1998).
Various ICPC formulations were reported with optimizing the porosity, surface architectures, resorption rates, and mechanical strength (Barralet et al., 2002; Bohner et al., 2005; Bodde et al., 2009; Ginebra et al., 2010). Furthermore, cell therapy was employed for bone defect treatment with ICPC to increase the bone regeneration efficiency (Humbert et al., 2019). Due to its self-renewal and multiple differentiation potential, mesenchymal stem cells (MSCs) have been used for cell-based therapy. The seeding of ICPC with stem cells included human bone marrow MSCs (hBMSCs) (He et al., 2013), human umbilical cord MSCs (hUCMSCs) (Zhao et al., 2010), human embryonic stem cells (hESCs) (Liu et al., 2014), and human-induced pluripotent stem cells (hiPSCs) (Liu et al., 2013a).
In stem cell therapy, MSCs need to proliferate for an optimal therapeutic dose and differentiate into multiple cell lineages for effective tissue repair and clinical treatment. Therefore, the culture medium is essential to supply nutrients for cell metabolism, proliferation, and differentiation in vitro. Currently, cell culture medium supplements are based on animal serum, mostly fetal bovine serum (FBS), which consists of a large number of low and high molecular weight biomolecules with different physiological activities (Gstraunthaler, 2003; van der Valk et al., 2018). However, it has reported the FBS could evoke the xenogeneic immunological reactions and raise the risk of transmitting bovine infections (Brunner et al., 2010). As ICPC have been widely investigated in a number of clinical trials for bone regeneration, ICPC scaffolds seeding with MSCs represent a new strategy to promote pre-vascularization and enhance bone tissue engineering efficacy (Lin et al., 2019). Therefore, it is urgently needed to switch the culture medium to a FBS-free, xeno-free alternative, thus enable the MSCs seeding ICPC product not cause any potential infections, allergies or malignancies and ultimately facilitate the clinical application.
Human platelet lysate (hPL) is a valuable, non-xenogenic alternative to FBS in cell culture (Bernardo et al., 2007; Rauch et al., 2011). The advantages of hPL includes that it is human-derived, serum-free, and cost-effective. An increasing number of studies demonstrated that hPL showed a growth-promoting effect on a multitude of cell lines (Fernandez-Rebollo et al., 2017). Among the various types of MSCs, periodontal ligament stem cells (hPDLSCs) play a key role in dental and periodontal tissue regeneration. Seo et al. (2004) identified hPDLSCs in the periodontal ligament (PDL) and demonstrated that hPDLSCs implanted into nude mice generated cementum/PDL-like structures that resembled the native PDL. Compared with other MSCs, hPDLSCs have a marked ability to generate multiple tissue types, including alveolar bone, cementum, and Sharpey’s fibers, making them an ideal source for cell-based dental therapy. However, there has no report that investigated the effect of hPL as a cell growth supplement on the proliferation and osteogenic differentiation of hPDLSCs in culture with ICPC.
In stem cell-based bone tissue engineering, the number of functioning cells in the bone injury site appears to be of critical importance. However, it was reported that few transplanted cells survived via local administration of cell suspensions. One study demonstrated that only 50% of initial donor BMSCs remained alive 48 h after implantation, and only 5% survived after 8 weeks (Giannoni et al., 2010). Another study showed that less than 1% of human multipotent stromal cells were detectable after 30 days post-implantation (Becquart et al., 2012). Thus the preferred approach was delivering a large number of cells to the target site via a carrier scaffold. Alginate hydrogel is highly hydrated with excellent biocompatibility and has shown great potential as a carrier for cell delivery (Izeia et al., 2020; Xu et al., 2021). Previous studies have reported that alginate microbeads are an ideal vehicle to localize the cells at the target defect site for cell-based therapy (Li et al., 2012; Pal et al., 2014). In addition, it has been reported that the incorporation of hPL could improve the viability, adhesion, and proliferation of a various types of cell in the alginate hydrogel (Sandri et al., 2015; Saporito et al., 2019). However, there has been no report that investigated the alginate hydrogel incorporation of hPL as a cell delivery system with ICPC for bone tissue regeneration.
The excellent biocompatibility of ICPC renders it suitable for cell and growth factor delivery. hPL has been used as a cell nutrient supplement for cell expansion, as well as a source of growth factors for tissue engineering applications. To date, a literature search revealed no report on the development of injectable hPDLSC-hPL-CPC-microbead constructs for bone tissue engineering. There has been no report on hPL-containing degradable microbeads as a vehicle to deliver hPDLSCs. There has been no report of investigating hPL as a cell growth supplement for hPDLSCs in culture with CPC, using the FBS-free and xeno-free cell culture medium alternative to avoid the risk of transmitting bovine infections and the initiation of xenogeneic immunological reactions.
Therefore, the objectives of the present study were to: 1) Develop a novel construct of hPDLSCs encapsulated in hydrogel microbeads with hPL and ICPC; 2) Investigate the proliferation and osteogenic differentiation of hPDLSCs in microbeads in ICPC with hPL as the xeno-free supplement for bone regeneration. The following hypotheses were tested: 1) Encapsulating hPDLSCs in alginate microbeads would protect the hPDLSCs during the setting reaction of ICPC paste; 2) The incorporation of hPL could improve the viability of hPDLSCs in the alginate microbeads; 3) The hPDLSCs released from the degradable alginate microbeads in ICPC would be able to proliferate and undergo osteogenic differentiate when using a xeno-free growth medium with hPL.
Materials and methods
Fabrication of injectable calcium phosphate cement scaffold
The ICPC powder consisted of a mixture of TTCP and DCPA at a TTCP: DCPA molar ratio of 1:1 because this ratio was shown to produce apatite minerals (Xu and Simon, 2005). The TTCP powder was synthesized from a solid-state reaction between CaHPO4 and CaCO3 (Baker Chemical Company, NJ, United States) and then ground to obtain a median particle size of 17 μm. The DCPA powder (Baker Chemical) was ground to obtain a median particle size of 1 μm. Chitosan was used as cement liquid as previous studies have indicated that the addition of chitosan to CPC enhanced the strength and durability of ICPC (Weir and Xu, 2008). Two cement liquids at 0 and 7.5% chitosan were used. The ICPC liquid consisted of chitosan malate mixed with sterile distilled water at a chitosan/(chitosan + water) mass fraction of 7.5%. The ICPC powder was mixed with the chitosan liquid at a mass ratio of 2:1. Each paste was placed into 3 mm3 × 4 mm3 × 25 mm3 stainless steel molds to make flexural specimens. Each specimen was covered with a glass slide on each side, clamped, and incubated in a humidor with 100% relative humidity at 37°C for 24 h.
Load-bearing properties
The hardened specimens were demolded and immersed in distilled water for 24 h. A three-point flexural test was used to fracture the specimens in a universal Testing Machine (MTS, Eden Prairie, MN). Three-point bending strength S = 3FmaxL/(2 bh2), where Fmax is the maximum load on the load-displacement (F-d) curve, L is the span, b is the specimen width and h is the thickness. Elastic modulus E = (F/d) (L3/[4 bh3]), where load F divided by displacement d is the slope (Xu et al., 2002).
hPDLSC isolation from extracted human teeth
hPDLSCs were isolated from the PDL tissues on extracted human premolars collected from healthy patients. The procedures were approved by the Institutional Review Board of the University of Maryland Baltimore (HP-00052180). All patients or their guardians were informed with written consent. The PDL tissue was scraped off the middle third of the root surfaces (Chen et al., 2020). The scraped tissues were digested in a solution of 3 mg/ml collagenase I (Worthington Biochem, Freehold, NJ, United States) and 4 mg/ml dispase (Roche, Mannheim, Germany) for 1 h at 37°C in a humidified atmosphere with 5% CO2. Then the PDL samples were placed into culture dishes with growth medium. The medium consisted of Dulbecco’s modified Eagle’s medium (DMEM, GIBCO BRL, Grand Island, NY, United States) supplemented with 20% fetal bovine serum (FBS, Invitrogen, Carlsbad, CA, United States) and 1% penicillin/streptomycin (P.S, GIBCO BRL). The samples were incubated at 37°C with 5% CO2. Single cells were observed 3 days later, and cell colonies were formed at 7–10 days. The individual cell colonies were digested to a single cell suspension using filter paper (Whatman, TISCH Scientific, North Bend, Ohio, United States) with 0.25% Trypsin-EDTA (GIBCO BRL), and transferred to 24-well culture plates, and the cells were passaged when they reach to 80% confluency. It was shown in our previous study that the hPDLSCs were positive for STRO-1, CD146+, and OCT4 and negative for CD34 and CD45 (Chen et al., 2020). Passage 3 cells were used in subsequent experiments.
hPDLSC encapsulation in alginate microbeads
Alginate was made degradable by oxidation at 7.5%, following a method described in a previous study (Bouhadir et al., 2001). Three types of alginate-based microbeads were fabricated to encapsulate the hPDLSCs: 1) Alginate-fibrin microbeads (DAMB + fibrin); 2) Alginate-hPL microbeads (DAMB + hPL); 3) Alginate-fibrin-hPL microbeads (DAMB + fibrin + hPL). A 1.2% (mass fraction) sodium alginate solution was prepared by dissolving oxidized alginate in saline (155 mmol/L NaCl) containing 0.1% fibrinogen for preparing type-1 microbeads. For type 2 microbeads, oxidized alginate was dissolved by 50% dilution of hPL with saline. For type 3 microbeads, fibrinogen was added at a concentration of 0.1% to the alginate-hPL solution. hPDLSCs were encapsulated at a density of 100,000 cells/mL of alginate solution. The alginate-cell solution was loaded into a syringe that was connected to a bead-generating device (Var J). Nitrogen gas was fed to the gas inlet and a pressure of 8 psi was established to form a coaxial airflow to break up the alginate droplets (Zhou and Xu, 2011). For type 1 and type 3 microbeads, the droplets fell into a well of 6-well plate containing 8 ml of 200 mmol/L calcium chloride solution plus 1 NIH units/mL of thrombin (Millipore Sigma) and cross-linked for 20 min to form DAMB + fibrin and DAMB + fibrin + hPL. For DAMB + hPL, the droplets fell into a well of 200 mmol/L calcium chloride solution and crosslinked for 20 min to form microbeads. A microscope (Eclipse TE-2000S, Nikon, Melville, NY) was used to measure the size of the microbeads.
Viability of hPDLSCs inside alginate microbeads
The ICPC paste was extruded through a sterile syringe into a 12 well-plate with 0.05 ml of paste per well. The cement was set in an incubator for 30 min. The hPDLSC-encapsulating microbeads were suspended in the culture medium and added into the 24 well-plate containing CPC for co-culture. The viability and proliferation of hPDLCSs were tested in four groups:
1. ICPC + DAMB + fibrin + DMEM group (Alginate-fibrin microbeads encapsulating 1×105 hPDLSCs with 0.05 ml ICPC scaffold in DMEM);
2. ICPC + DAMB + fibrin + FBS group (Alginate-fibrin microbeads encapsulating 1 × 105 hPDLSCs with 0.05 ml ICPC scaffold in growth medium containing 10% FBS);
3. ICPC + DAMB + hPL group (Alginate-hPL microbeads encapsulating with 1 × 105 hPDLSCs with 0.05 ml ICPC scaffold, cultured in DMEM for the first three days and then in growth medium containing 2.5% hPL);
4. ICPC + DAMB + fibrin + hPL group (Alginate-fibrin-hPL microbeads encapsulating with 1 × 105 hPDLSCs with 0.05 ml ICPC scaffold, cultured in DMEM for the first three days and then in growth medium containing 2.5% hPL).
After culturing for 1, 7, and 14 days, cells were stained with a live/dead kit (Invitrogen) and observed via epifluorescence microscopy (Eclipse TE-2000S, Nikon). Two images were taken at random locations for each sample, with four samples yielding 8 images at each time point for each group. Live and dead cells were counted via Image-Pro Plus software. The percentages of live cells (PLive) were calculated. PLive = number of live cells/(number of live cells + number of dead cells).
The proliferation rate of hPDLSCs was investigated via Cell Counting Kit-8 (CCK-8, Dojindo, Tokyo, Japan), following the manufacturer’s protocol. At 1, 3, 7, and 14 days, the culture medium was carefully removed and placed with 500 µl DMEM containing 50 µl CCK-8 dye. After 2 h incubation at 37°C, a 200 µl aliquot from each well was placed in a 96-well plate and the absorbance at an optical density of 450 nm (OD450 nm) was measured with a microplate reader (SpectraMax M5, Molecular Devices, Sunnyvale, CA, United States).
To evaluate the number of hPDLSCs released from the degrading alginate microbeads, the samples were imaged via microscopy (Eclipse TE-2000S, Nikon). Two images were taken at random locations for each sample, with four samples yielding 8 images at each time point for each group. The number of the hPDLSCs with spindle-shape morphology was counted via Image-Pro Plus software. The density of the released hPDLSCs was calculated: DRelease = NRelease/A, where A is the area of the view field for DRelease.
Alkaline phosphatase activity assay
The hPDLSC-microbeads and ICPC scaffold were cultured in 24 well-plate with an osteogenic differentiation medium containing 10% FBS or 2.5% hPL. The basic osteogenic medium was DMEM growth medium with 100 nM dexamethasone (Millipore Sigma), 10 mM β-glycerophosphate (Millipore Sigma), 0.05 mM ascorbic acid (Millipore Sigma), 10 nM 1ɑ, 25-dihydroxy vitamin D3 (Millipore Sigma) and 1% P.S. Heparin was added to the hPL-based osteogenic medium at a final concentration of 2 U/mL. Negative control cultures were maintained in a medium containing 10% FBS. The medium was changed three times weekly. Four groups were tested to evaluate the osteogenic differentiation of hPDLSCs:
(1) DAMB + fibrin + FBS + control group (hPDLSCs cultured with DMEM +10% FBS +1% P.S);
(2) ICPC + DAMB + fibrin + FBS + osteo group (1 × 105 hPDLSCs encapsulated in DAMB + fibrin with 0.05 ml ICPC scaffold and cultured in DMEM +10% FBS +1% P.S + 100 nM dexamethasone +10 mM β-glycerophosphate + 0.05 mM ascorbic acid +10 nM 1ɑ, 25-dihydroxy vitamin D3);
(3) ICPC + DAMB + hPL + osteo group (1 × 105 hPDLSCs encapsulated in DAMB + hPL with 0.05 ml ICPC scaffold and cultured in DMEM +2.5% hPL +1% P.S + 2 U/mL heparin +100 nM dexamethasone +10 mM β-glycerophosphate + 0.05 mM ascorbic acid +10 nM 1ɑ, 25-dihydroxy vitamin D3);
(4) ICPC + DAMB + fibrin + hPL + osteo group (1 × 105 hPDLSCs encapsulated in DAMB + fibrin + hPL with 0.05 ml ICPC scaffold and cultured in DMEM +2.5% hPL +1% P.S + 2U/mL heparin +100 nM dexamethasone +10 mM β-glycerophosphate + 0.05 mM ascorbic acid +10 nM 1ɑ, 25-dihydroxy vitamin D3).
At 1, 7, and 14 days, cells were lysed in a 0.2% Triton X-100 (Millipore Sigma) solution. The ALP activity of the cell lysate was measured by using an ALP Assay kit (QuantiChrom, BioAssay Systems, Hayward, CA, United States) with p-Nitrophenylphosphate (pNPP) as a substrate. The ALP activity was determined by measuring the absorbance at an optical density of 405 nm using a microplate reader (SpectraMax M5). The protein of cell lysate was quantified using Protein Assay Kit (Pierce BCA, Thermo Scientific, Rockford, IL, United States) following the manufacturer’s protocol. The ALP activity was normalized to the protein amount and reported as mU/mg protein (Zhao et al., 2019).
Quantitative real-time PCR
The ICPC scaffold and hPDLSC-microbeads were cultured in 12 well-plates. Three groups were tested for the osteogenic gene expressions and Alizarin Red S (ARS, Millipore Sigma) staining:
1. ICPC + DAMB + fibrin + FBS + control group (2 × 105 hPDLSCs encapsulated in DAMB + fibrin and cultured with 0.1 ml ICPC scaffold in FBS-based growth medium)
2. ICPC + DAMB + fibrin + FBS + osteo group (2 × 105 hPDLSCs encapsulated in DAMB + fibrin and cultured with 0.1 ml ICPC scaffold in FBS-based osteogenenic medium);
3. ICPC + DAMB + fibrin + hPL + osteo group (2 × 105 hPDLSCs encapsulated in DAMB + fibrin + hPL microbeads and cultured with 0.1 ml ICPC scaffold in hPL-based osteogenenic medium).
The total cellular RNA of the cells was extracted using TRIzol reagent (Invitrogen) and reverse-transcribed into cDNA using a High-capacity cDNA Reverse Transcription kit (Applied Biosystems) in a thermal cycler (GenAmp PCR 2720, Applied Biosystems). RT2 SYBR® Green ROX qPCR Mastermix (Qiagen, Germantown, MD, United States) was used to quantify the transcript levels of glyceraldehyde 3-phosphate dehydrogenase (GAPDH), alkaline phosphatase (ALP), runt-related transcription factor-2 (RUNX2), Collagen type 1 (COL1), and Osteogenicpontin (OPN). The human-specific primers were synthesized commercially (Millipore Sigma), and the sequences of the primers are listed in Table 1. The qPCR data collection and analyses were performed using a QuantStudio™ 3 Real-Time PCR System (Thermo Scientific). Relative expressions were calculated using the 2−ΔΔCT method and normalized by the Ct value of the housekeeping gene GAPDH. The Ct value of hPDLSCs in the control group at 1 day served as the calibrator.
Bone mineral synthesis by hPDLSCs
At 1, 14, and 21 days, the hPDLSCs were fixed with 4% paraformaldehyde for 15 min and stained with ARS. After staining for 30 min, the ARS solution was removed and rinsed with distilled water to remove any loose ARS. The samples were imaged via microscopy (Eclipse TE-2000S, Nikon). For quantification, the stained mineralization was de-stained in 10% cetylpyridinium chloride (Millipore Sigma) for 30 min and the concentration was measured at an optical density of 652 nm using the microplate reader (SpectraMax M5). The ARS concentration of the control group at 1 day served as the calibrator.
Statistical analysis
One-way and two-way analyses of variance (ANOVA) were performed to detect the significant differences, followed by Tukey’s test as a post hoc comparison. Statistical analyses were performed by SPSS 19.0 software (SPSS, Chicago, IL, United States) at an alpha of 0.05.
Results
Load-bearing properties
The flexural strength and elastic modulus of the ICPC scaffolds are plotted in Figure 1. Flexural strength and elastic modulus of ICPC scaffolds was 8.4 ± 0.91 MPa and 1.56 ± 0.1 GPa, significantly higher than 6.4 ± 0.43 MPa and 1.12 ± 0.23 GPa of the control group, respectively (p < 0.05). This demonstrates that the incorporation of chitosan improved the mechanical properties of the ICPC scaffold. Both of these values are higher than those of cancellous bone reported in the literature (Damien and Parsons, 1991).
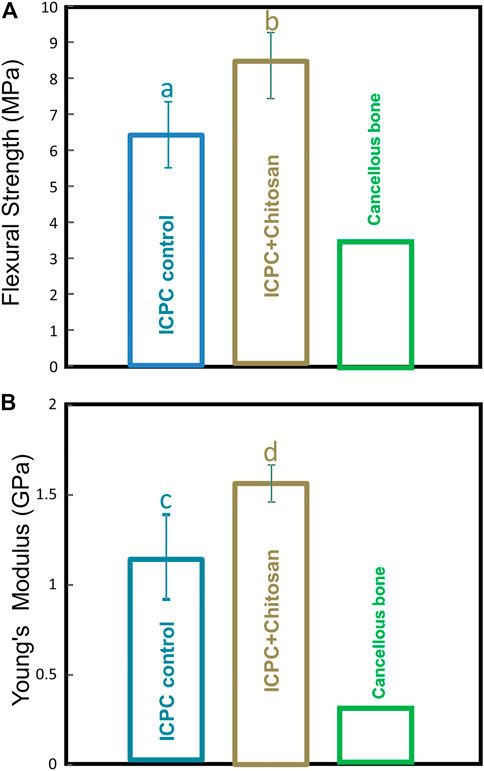
FIGURE 1. Load-bearing property of scaffolds in ICPC + Chitosan group and control group: (A) flexural strength; (B) elastic modulus (mean ± sd; n = 4). Values for cancellous bone were obtained from the literature (Damien and Parsons, 1991).
Encapsulated hPDLSC proliferation and viability in microbeads
The diameter of 100 random-selected alginate microbeads was measured by magnification for the three groups. These values (mean ± sd; n = 100) which were 312 ± 58 μm, 367 ± 56 μm and 297 ± 646 μm for DAMB + fibrin, DAMB + hPL, and DAMB + fibrin + hPL respectively.
Representative live/dead staining images from 1 to 14 days are shown in Figures 2(A–F). At 1 day, there were numerous live cells (stained green) and a few dead cells (stained red) in all groups (Figures 2A–E). At 7 days, the hPDLSCs were released from the degradable microbeads in the ICPC + DAMB + fibrin + hPL, ICPC + DAMB + hPL, and ICPC + DAMB + fibrin + FBS. The cells exhibited a polygonal morphology and a relatively high viability (Figures 2F–H). At 14 days, the degradation of microbeads accelerated and most of the cells were released (Figures 2J–L). The PLive increased with time due to proliferation in the ICPC + DAMB + fibrin + hPL, ICPC + DAMB + hPL, and ICPC + DAMB + fibrin + FBS. At 1 day, the PLive of hPDLSCs in ICPC + DAMB + fibrin + hPL and ICPC + DAMB + hPL was slightly higher than that in the ICPC + DAMB + fibrin + FBS group, indicating that incorporation of hPL into the microbeads could better protect the hPDLSCs from the pH change and ion activities during the ICPC setting (Figure 2M). In contrast, very few cells were observed to attach and spread in the ICPC + DAMB + fibrin + DMEM, the PLive of the hPDLSCs was lower, due to the lack of nutrition supplements.
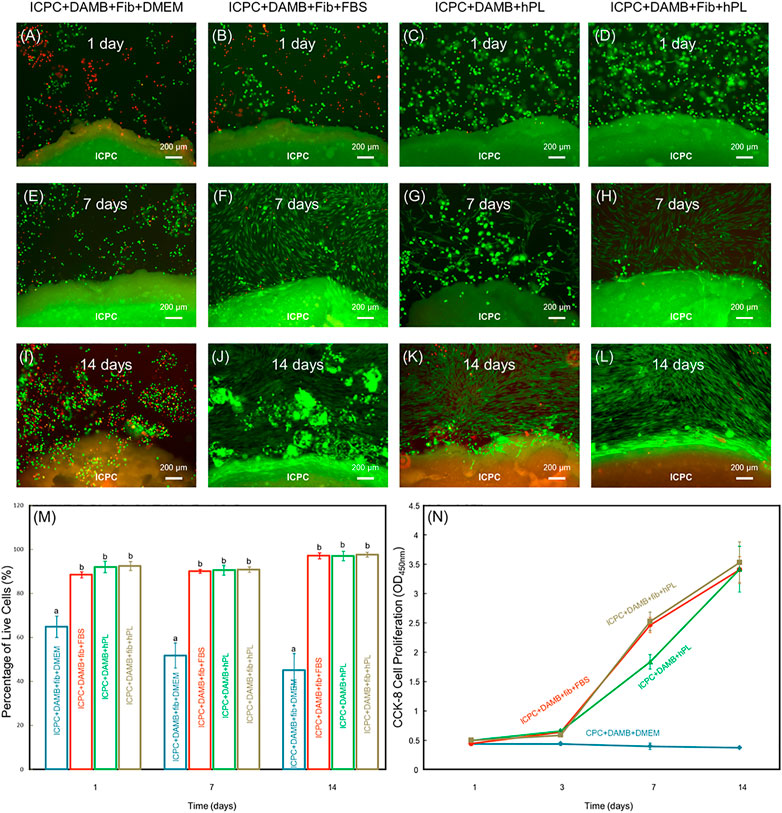
FIGURE 2. Live/dead results of human periodontal ligament stem cells (hPDLSCs) in ICPC + DAMB + fibrin + DMEM, ICPC + DAMB + fibrin + FBS, ICPC + DAMB + hPL, and ICPC + DAMB + fibrin + hPL constructs (A–L). Percentage of live cells at 1, 7, and 14 days (M). Each value is (mean ± sd; n = 5). CCK-8 cell viability (absorbance at 450 nm) increased with culture time (N). Each value is (mean ± sd; n = 6). Bars with dissimilar letters in the plot indicate values that are significantly different from each other (p < 0.05).
The cell growth rate was evaluated via the CCK-8 assay (Figure 2N). At 1 day and 3 days, the proliferation of hPDLSCs was low in the groups of ICPC + DAMB + fibrin, ICPC + DAMB + hPL, and ICPC + DAMB + fibrin + hPL. At 7 days, as more cells were released from the degradable microbeads, the cell growth was significantly enhanced. The proliferation rate in the ICPC + DAMB + fibrin and ICPC + DAMB + fibrin + hPL groups was higher than that of the ICPC + DAMB + hPL group (p < 0.05), indicating that the incorporation of fibrin accelerated the hPDLSCs migrated out from the microbeads. The absorbance of the hPDLSCs in the ICPC + DAMB + fibrin + DMEM group was lower over time, indicating that the DMEM did not support the proliferation of the hPDLSCs encapsulated in the microbeads.
The DRelease was evaluated for ICPC + DAMB + fibrin, ICPC + DAMB + hPL, and ICPC + DAMB + fibrin + hPL. At 7 days, hPDLSCs were released from the degradable microbeads (Figures 3A–F). The DRelease of ICPC + DAMB + fibrin + hPL was significantly higher than that of ICPC + DAMB + hPL (Figure 3G). This demonstrated that adding fibrin increased the degradation rate of the microbeads. As the microbeads degraded, cells were released, and the DRelease of each group increased. There was no difference in DRelease between each group at 14 days (Figure 3G).
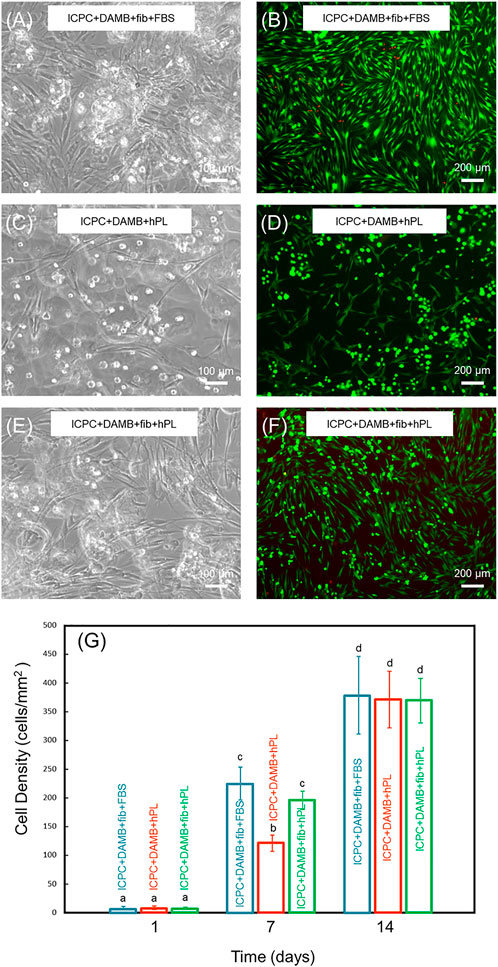
FIGURE 3. Representative images of the released hPDLSCs in ICPC + DAMB + fibrin + FBS group (A,B), ICPC + DAMB + hPL group (C,D), and ICPC + DAMB + fibrin + hPL group (E,F) at 7 days. The cell density of the released cells at 1, 7, and 14 days (G). Each value is (mean ± sd; n = 4). Bars with dissimilar letters indicate significantly different values.
Alkaline phosphatase activity of hPDLSCs
The ALP activity of hPDLSCs encapsulated in various types of alginate-based microbeads increased with time in osteogenic medium supplemented with 10% FBS or 2.5% hPL, indicating successful differentiation towards osteoblasts (Figure 4). At 14 days, the ALP activity was 69.97 ± 16.96 mU/mg for the ICPC + DAMB + fibrin + hPL group, significantly higher than 30.68 ± 2.86 mU/mg of the ICPC + DAMB + fibrin group (p < 0.05) and 4.12 ± 1.65 mU/mg of the control group (p < 0.01). These results indicate that the hPL-based osteogenic medium significantly enhanced the osteogenic differentiation of the hPDLSCs in ICPC scaffold.
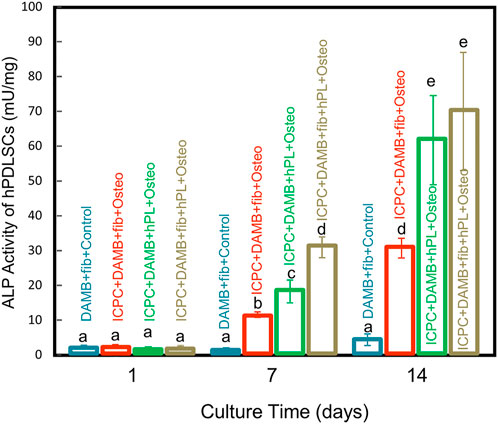
FIGURE 4. ALP activity of encapsulated hPDLSCs inside alginate hydrogel microbeads in the DAMB + fibrin + FBS + control group, ICPC + DAMB + fibrin + FBS + osteo group, ICPC + DAMB + hPL + osteo group, and ICPC + DAMB + fibrin + hPL + osteo group at 1, 7 and 14 days (mean ± sd, n = 4). Dissimilar letters indicate values that are significantly different from each other (p < 0.05).
Osteogenic gene expression
Up-regulations of ALP, RUNX2, COL1, and OPN were observed in hPDLSCs in the ICPC + DAMB + fibrin + FBS + osteo group and ICPC + DAMB + fibrin + hPL + osteo group. At 7 days, the values of ALP, RUNX2, COL1, and OPN in the ICPC + DAMB + fibrin + hPL + osteo group and ICPC + DAMB + fibrin + FBS + osteo group were 6–11 folds and 4-7 folds those of the control group, respectively (Figure 5). Furthermore, significantly higher expressions of ALP and RUNX2 were observed in ICPC + DAMB + fibrin + hPL + osteo than in ICPC + DAMB + fibrin + FBS + osteo. This indicates that the hPL promoted higher osteogenic differentiation of hPDLSCs than the commonly used 10% FBS.
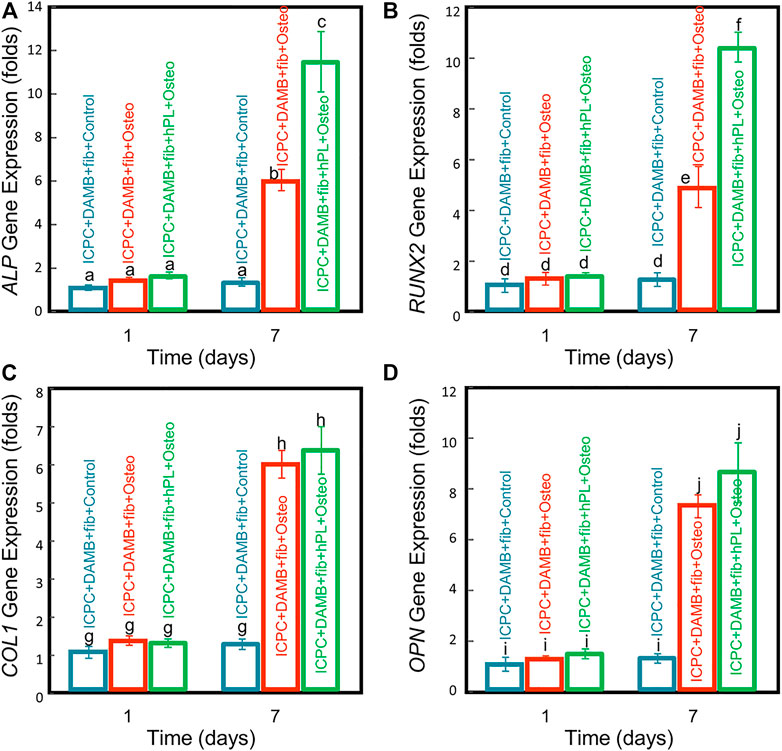
FIGURE 5. qRT-PCR assay of osteogenic differentiation of hPDLSC-microbeads co-cultured with ICPC scaffolds for 1 and 7 days: (A) ALP, (B) RUNX2, (C) COL1, and (D) OPN gene expressions (n = 5). Values with dissimilar letters are significantly different from each other (p < 0.05).
Alizarin Red staining of minerals synthesized by hPDLSCs
At 21 days of osteogenic differentiation, the hPDLSCs from the microbeads exhibited a high viability and proliferation. Figures 6A,D,G show that the hPDLSCs released from the microbeads significantly proliferated and adhered to ICPC scaffold. This indicates that the ICPC scaffold was cytocompatible.
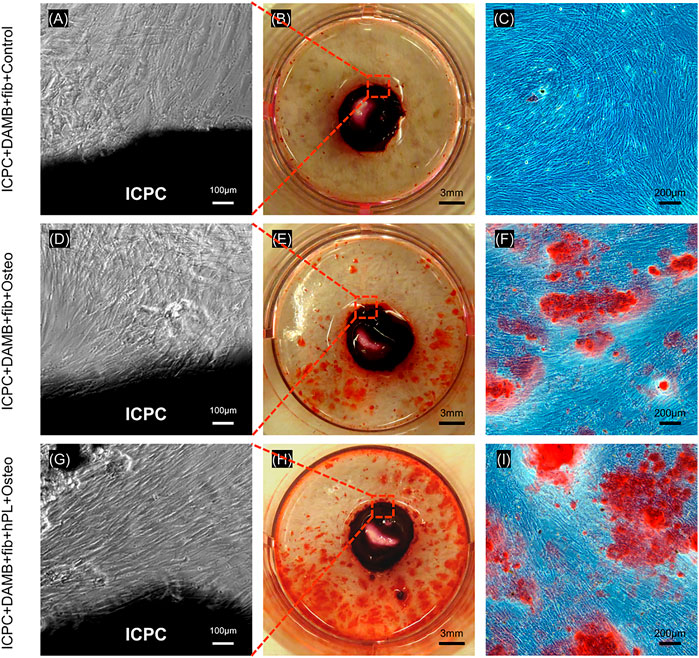
FIGURE 6. hPDLSCs showed high proliferation in ICPC for 21 days (A,D,G). Representative ARS staining images of mineral synthesis by hPDLSCs with ICPC (B,E,H). Representative images of mineralized nodules formed by the hPDLSCs. More mineralized nodules were present in the ICPC + DAMB + fibrin + hPL + osteo group than in ICPC + DAMB + fibrin + FBS + osteo group and control group (C,F,I).
Representative ARS staining images of bone mineral secretion by hPDLSCs in the 12-well plate with ICPC are shown in Figures 6B,E,H. The red staining of mineralized nodules formed by the hPDLSCs was denser in the ICPC + DAMB + fibrin + hPL + osteo group than that of the ICPC + DAMB + fibrin + FBS + osteo group Figures 6C,F,I. The synthesized bone mineral in ICPC + DAMB + fibrin + FBS + osteo and ICPC + DAMB + fibrin + hPL + osteo increased with culture time from 1 to 21 days. The synthesized bone mineral amount in the ICPC + DAMB + fibrin + hPL + osteo was 6.9-fold and 13.2-fold that of the control group, at 14 days and 21 days, respectively (Figure 7). These results demonstrate that the hPL-based osteogenic medium significantly enhanced the osteogenic differentiation of the hPDLSCs.
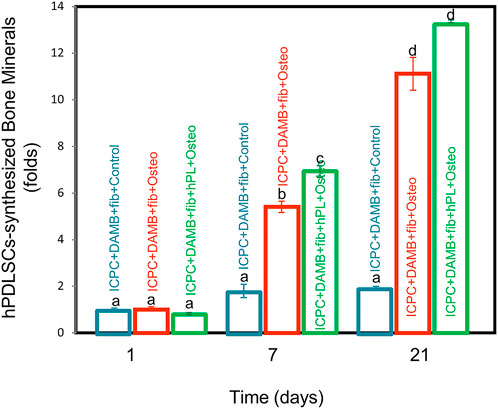
FIGURE 7. Quantitative bone mineral synthesis by hPDLSCs. The hPDLSC-synthesized bone mineral in ICPC + DAMB + fibrin + hPL + osteo group and ICPC + DAMB + fibrin + FBS + osteo group was 13.2 folds and 11.1 folds that of the control group, respectively (mean ± sd; n = 6). Values with dissimilar letters are significantly different from each other (p < 0.05).
Discussion
This study represents the first report to investigate degradable alginate hydrogel incorporated with hPL inside an injectable calcium phosphate scaffold as a cell delivery system for bone regeneration. The hPDLSCs showed excellent viability while being encapsulated in the DAMB + fibrin + hPL microbeads in ICPC scaffold. When the setting of the ICPC was complete, the hPDLSCs were gradually released from the degradable hydrogel microbeads and grew rapidly in the scaffold. Furthermore, the osteogenic differentiation of hPDLSCs was significantly enhanced by the osteogenic medium supplemented with hPL. This study is schematically shown in Figure 8. These findings demonstrate a promising and novel xeno-free approach to delivering hPDLSCs in ICPC scaffold for bone regeneration.
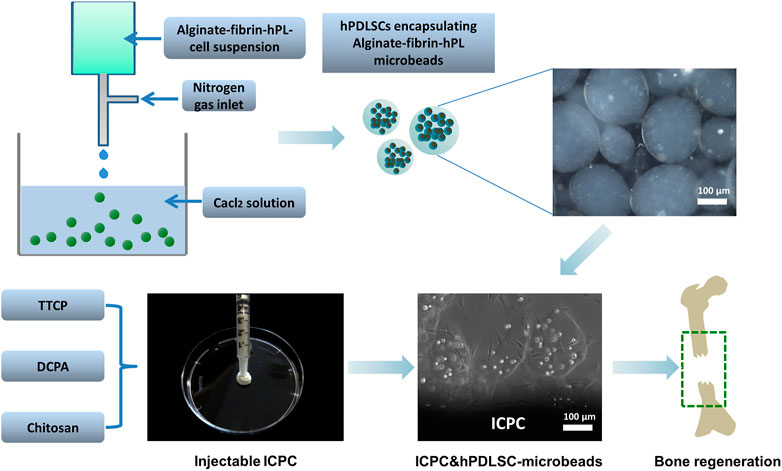
FIGURE 8. Schematic illustration showing the encapsulation of hPDLSCs in alginate microbeads incorporated with hPL and delivery with ICPC for bone regeneration.
The ICPC paste can be filled into a bone defect with an intimate adaptation to complex defect cavities and set in situ to form bioresorbable hydroxyapatite (Weir et al., 2006; Xu et al., 2006). However, ionic activities and pH variations during the setting of ICPC paste could exert a cytotoxic effect on the cells (Matsuya et al., 2000; Simon et al., 2004; Przekora, 2019). TTCP and DCPA dissolved in the chitosan solution as Ca2+, PO43-, and OH− ions (Weir et al., 2006), which then re-precipitated to form hydroxyapatite: 2Ca4(PO4)2O+2CaHPO4 → Ca10(PO4) 6(OH)2 (Weir et al., 2006). It was reported that the pH during the setting of ICPC could be increased to approximately 10 (Simon et al., 2004). Therefore, there was a need to protect the cells from the ICPC setting reaction.
Alginate is among the most common natural polymers for the encapsulation and delivery of cells because of its many outstanding properties such as biocompatibility, gel-forming ability, non-toxicity, and ease of process (Gasperini et al., 2014; Izeia et al., 2020; Xu et al., 2021). However, the absence of alginate degrading enzyme (alginase) in the human body limits the degradability of the alginate. As the setting reaction of ICPC paste is largely complete after 1 day, it would be desirable for the alginate microbeads to quickly degrade, thus releasing the cells from the microbeads for enhanced cell viability and proliferation. A previous study reported that alginate could be chemically modified using oxidizing agents such as sodium periodate to produce oxidized alginate that was hydrolytically degradable (Zhou and Xu, 2011). In addition, it has been reported that adding fibrin could improve the attachment and proliferation of the cells in the alginate hydrogel, as well as to accelerate the release of cells from the alginate-based microbeads (Liu et al., 2013b). Fibrinogen was converted to fibrin via the mediation of thrombin and self-assembles into fibrin mesh, providing cell binding sites for cell attachment, migration, and proliferation (Li et al., 2015). As the cells migrated out of the microbeads, the porosity of the hydrogel increased, thus accelerating the degradation of the hydrogel. A previous study showed that the cell-encapsulating-alginate-fibrin-microbeads had diameters of about 100–500 μm, and the microbeads started to degrade at day 4 and released the encapsulated hiPSCs (Wang et al., 2016). Another study encapsulated hUCMSCs into the oxidized alginate-fibrin microbeads of about 300 μm in sizes (Chen et al., 2012). The microbeads started to release the cells at 4 days and completely degraded at 21 days (Chen et al., 2012). Therefore, microbeads with sizes of several hundred microns were suitable for injection, and were quickly degraded to release the cells.
FBS is the most widely-used supplement for the cultivation and expansion of eukaryotic cells. FBS contains essential components for cell growth and maintenance such as hormones, vitamins, transport proteins, trace elements, spreading, and growth factors (Brunner et al., 2010), which is effective on most types of human and animal cells. However, FBS-expanded MSCs could evoke immune responses against xenogenic serum antigens in the human body (Spees et al., 2004; Tonti and Mannello, 2008). In addition, FBS is a potential source of microbial contaminants such as fungi, bacteria, viruses, or prions. Indeed, a 20%–50% contamination rate of the virus was reported for FBS (Even et al., 2006). Furthermore, the ingredients of FBS are not precisely defined, and lot-to-lot variability and unintended interactions with test substances can lead to unexpected or undesired outcomes in the clinical application (Jochems et al., 2002). Therefore, it is critically important and highly desirable to develop a FBS-free medium as a cell nutrition supplement for cell-based therapies.
The use of hPL for MSC expansion was first reported by Doucet et al., in 2005 (Doucet et al., 2005). Since then, hPL has been proven as a viable alternative to FBS, enabling efficient propagation of MSC under animal serum-free conditions for clinical application. There are several advantages for hPL for cell growth supplement: 1) hPL can be easily obtained and produced using freeze-thaw procedures (Dessels et al., 2016); 2) Because hPL is derived from humans, neither bovine viruses nor immune reactions against bovine proteins are a concern (Saury et al., 2018); 3) hPL can be used in autologous settings to minimize the risk of immunological reactions (Sánchez et al., 2003); 4) hPL is more efficient in terms of costs and proliferation rate than FBS for certain types of MSCs (Burnouf et al., 2016).
In the present study, 2.5% hPL promoted the proliferation of hPDLSCs when compared with the 10% FBS. In a previous study, the use of 10% FBS showed a slightly higher proliferation of hPDLSCs as compared to 5% hPL. However, the difference in colony number was not statistically significant (Abuarqoub et al., 2019). In another study, hPDLSCs were cultured using a medium supplemented with 10% FBS, 5% PL + 5% FBS, or 10% PL. It was revealed that the media containing 5% PL + 5% FBS resulted in more significant stimulation of cell growth, when compared with those containing either 10% FBS or 10% PL. Furthermore, a tendency toward enhanced proliferation was exhibited in media containing 10% PL as compared with media containing 10% FBS (Wu et al., 2017). The differences of hPL concentration or hPDLSCs could be associated with the difference in the preparation method, the variability in the donors, and the storage conditions. Hence, the quality of hPL used by different laboratories reported in the literature may be somewhat different from each other (Christgau et al., 2006; Ogino et al., 2006; Schallmoser et al., 2007).
The implantation of ICPC scaffold with MSCs has achieved ectopic and orthotopic bone formation and critical-sized defect healing (Wang P. et al., 2014; Xu et al., 2017). The seeded MSCs could directly deposit bone matrix minerals in the scaffold due to their osteogenic differentiation potential. The therapeutic benefit of the transplanted MSCs was associated with a paracrine mechanism that stimulated the recruitment of host cells. These host cells included osteoblast progenitors, endothelial cells, and osteoclasts, which took over the responsibility of subsequent bone formation and remodeling (Wang et al., 2011; Wang J. et al., 2014). Interestingly, it has been revealed that MSCs could enhance bone repair by modulating the foreign body reaction to ICPC, attracting circulating monocytes, and inducing their differentiation into osteoclasts, thus favoring bone formation (Gamblin et al., 2014).
In the present study, the hPDLSCs in ICPC were gradually released from the degradable hydrogel microbeads and underwent differentiation into osteogenic lineage by the hPL-based medium, without exposure to the animal serum. Moreover, higher ALP activity, osteogenic expression, and bone mineralization were achieved in ICPC + DAMB + fibrin + hPL construct than in ICPC + DAMB + fibrin + FBS construct. Therefore, the novel hPDLSC-hPL-microbeads-ICPC construct is a highly promising xeno-free approach for bone regeneration. Future in vivo studies are needed to evaluate the bone regenerative capacity of the hPDLSC-hPL-microbeads-ICPC construct for the treatment of bone defects in animal models.
Conclusion
This study demonstrated for the first time the hPDLSC-encapsulation in degradable alginate hydrogel microbeads with hPL inside an injectable calcium phosphate scaffold for bone regeneration. The ICPC scaffold was biocompatible, mechanically load-bearing, while supporting hPDLSC attachment, proliferation, and osteogenic differentiation with the hPL as xeno-free cell supplement. The microbeads incorporating with hPL protected the hPDLSCs from the setting reaction of ICPC. The encapsulated hPDLSCs in ICPC with hPL-based osteogenic medium underwent successful differentiation into the osteoblast lineage, with highly elevated ALP, RUNX2, COL1, and OPN as well as bone mineral synthesis. Therefore, the novel hPDLSC-hPL-microbeads-ICPC construct is highly promising for bone regeneration without the risk of infection from unknown pathogens by using animal-origin serums.
Data availability statement
The original contributions presented in the study are included in the article/Supplementary Material, further inquiries can be directed to the corresponding authors.
Author contributions
Study conception and design: GQ, MH, JL, and HX Acquisition of data: GQ, TM, and DK Analysis and interpretation of data: GQ, TM, MW, AS, and TO Critical revision: GQ, PW, LZ, HX, and YX.
Funding
This study was supported by National Natural Science Foundation of China 31771051 (LZ) and National Institutes of Health grant R21 DE029611 (AS and HX).
Conflict of interest
The authors declare that the research was conducted in the absence of any commercial or financial relationships that could be construed as a potential conflict of interest.
Publisher’s note
All claims expressed in this article are solely those of the authors and do not necessarily represent those of their affiliated organizations, or those of the publisher, the editors and the reviewers. Any product that may be evaluated in this article, or claim that may be made by its manufacturer, is not guaranteed or endorsed by the publisher.
References
Abuarqoub, D. A., Aslam, N., Barham, R. B., Ababneh, N. A., Shahin, D. A., Al-Oweidi, A. A., et al. (2019). The effect of platelet lysate in culture of PDLSCs: An in vitro comparative study. PeerJ 7, e7465. doi:10.7717/peerj.7465
Barralet, J. E., Gaunt, T., Wright, A. J., Gibson, I. R., and Knowles, J. C. (2002). Effect of porosity reduction by compaction on compressive strength and microstructure of calcium phosphate cement. J. Biomed. Mat. Res. 63 (1), 1–9. doi:10.1002/jbm.1074
Becquart, P., Cambon-Binder, A., Monfoulet, L. E., Bourguignon, M., Vandamme, K., Bensidhoum, M., et al. (2012). Ischemia is the prime but not the only cause of human multipotent stromal cell death in tissue-engineered constructs in vivo. Tissue Eng. Part A 18 (19-20), 2084–2094. doi:10.1089/ten.TEA.2011.0690
Bernardo, M. E., Avanzini, M. A., Perotti, C., Cometa, A. M., Moretta, A., Lenta, E., et al. (2007). Optimization of in vitro expansion of human multipotent mesenchymal stromal cells for cell-therapy approaches: Further insights in the search for a fetal calf serum substitute. J. Cell. Physiol. 211 (1), 121–130. doi:10.1002/jcp.20911
Bodde, E. W., Habraken, W. J., Mikos, A. G., Spauwen, P. H., and Jansen, J. A. (2009). Effect of polymer molecular weight on the bone biological activity of biodegradable polymer/calcium phosphate cement composites. Tissue Eng. Part A 15 (10), 3183–3191. doi:10.1089/ten.TEA.2008.0694
Bohner, M., Gbureck, U., and Barralet, J. E. (2005). Technological issues for the development of more efficient calcium phosphate bone cements: A critical assessment. Biomaterials 26 (33), 6423–6429. doi:10.1016/j.biomaterials.2005.03.049
Bouhadir, K. H., Lee, K. Y., Alsberg, E., Damm, K. L., Anderson, K. W., and Mooney, D. J. (2001). Degradation of partially oxidized alginate and its potential application for tissue engineering. Biotechnol. Prog. 17 (5), 945–950. doi:10.1021/bp010070p
Brunner, D., Frank, J., Appl, H., Schöffl, H., Pfaller, W., and Gstraunthaler, G. (2010). Serum-free cell culture: The serum-free media interactive online database. Altex 27 (1), 53–62. doi:10.14573/altex.2010.1.53
Burnouf, T., Strunk, D., Koh, M. B., and Schallmoser, K. (2016). Human platelet lysate: Replacing fetal bovine serum as a gold standard for human cell propagation? Biomaterials 76, 371–387. doi:10.1016/j.biomaterials.2015.10.065
Chen, L., Liu, J., Guan, M., Zhou, T., Duan, X., and Xiang, Z. (2020). <p>Growth factor and its polymer scaffold-based delivery system for cartilage tissue engineering</p>. Int. J. Nanomedicine 15, 6097–6111. doi:10.2147/ijn.s249829
Chen, W., Zhou, H., Weir, M. D., Bao, C., and Xu, H. H. (2012). Umbilical cord stem cells released from alginate-fibrin microbeads inside macroporous and biofunctionalized calcium phosphate cement for bone regeneration. Acta Biomater. 8 (6), 2297–2306. doi:10.1016/j.actbio.2012.02.021
Christgau, M., Moder, D., Hiller, K. A., Dada, A., Schmitz, G., and Schmalz, G. (2006). Growth factors and cytokines in autologous platelet concentrate and their correlation to periodontal regeneration outcomes. J. Clin. Periodontol. 33 (11), 837–845. doi:10.1111/j.1600-051X.2006.00991.x
Damien, C. J., and Parsons, J. R. (1991). Bone graft and bone graft substitutes: A review of current technology and applications. J. Appl. Biomater. 2 (3), 187–208. doi:10.1002/jab.770020307
Dessels, C., Potgieter, M., and Pepper, M. S. (2016). Making the switch: Alternatives to fetal bovine serum for adipose-derived stromal cell expansion. Front. Cell Dev. Biol. 4, 115. doi:10.3389/fcell.2016.00115
Doucet, C., Ernou, I., Zhang, Y., Llense, J. R., Begot, L., Holy, X., et al. (2005). Platelet lysates promote mesenchymal stem cell expansion: A safety substitute for animal serum in cell-based therapy applications. J. Cell. Physiol. 205 (2), 228–236. doi:10.1002/jcp.20391
Even, M. S., Sandusky, C. B., and Barnard, N. D. (2006). Serum-free hybridoma culture: Ethical, scientific and safety considerations. Trends Biotechnol. 24 (3), 105–108. doi:10.1016/j.tibtech.2006.01.001
Fernandez-Rebollo, E., Mentrup, B., Ebert, R., Franzen, J., Abagnale, G., Sieben, T., et al. (2017). Human platelet lysate versus fetal calf serum: These supplements do not select for different mesenchymal stromal cells. Sci. Rep. 7 (1), 5132. doi:10.1038/s41598-017-05207-1
Friedman, C. D., Costantino, P. D., Takagi, S., and Chow, L. C. (1998). BoneSource hydroxyapatite cement: A novel biomaterial for craniofacial skeletal tissue engineering and reconstruction. J. Biomed. Mat. Res. 43 (4), 428–432. doi:10.1002/(sici)1097-4636(199824)43:4<428:aid-jbm10>3.0.co;2-0
Gamblin, A. L., Brennan, M. A., Renaud, A., Yagita, H., Lézot, F., Heymann, D., et al. (2014). Bone tissue formation with human mesenchymal stem cells and biphasic calcium phosphate ceramics: The local implication of osteoclasts and macrophages. Biomaterials 35 (36), 9660–9667. doi:10.1016/j.biomaterials.2014.08.018
Gasperini, L., Mano, J. F., and Reis, R. L. (2014). Natural polymers for the microencapsulation of cells. J. R. Soc. Interface 11 (100), 20140817. doi:10.1098/rsif.2014.0817
Giannoni, P., Scaglione, S., Daga, A., Ilengo, C., Cilli, M., and Quarto, R. (2010). Short-time survival and engraftment of bone marrow stromal cells in an ectopic model of bone regeneration. Tissue Eng. Part A 16 (2), 489–499. doi:10.1089/ten.TEA.2009.0041
Ginebra, M. P., Espanol, M., Montufar, E. B., Perez, R. A., and Mestres, G. (2010). New processing approaches in calcium phosphate cements and their applications in regenerative medicine. Acta Biomater. 6 (8), 2863–2873. doi:10.1016/j.actbio.2010.01.036
Gstraunthaler, G. (2003). Alternatives to the use of fetal bovine serum: Serum-free cell culture. Altex 20 (4), 257–281. doi:10.14573/altex.2003.4.257
He, F., Li, J., and Ye, J. (2013). Improvement of cell response of the poly(lactic-co-glycolic acid)/calcium phosphate cement composite scaffold with unidirectional pore structure by the surface immobilization of collagen via plasma treatment. Colloids Surfaces B Biointerfaces 103, 209–216. doi:10.1016/j.colsurfb.2012.10.018
Humbert, P., Brennan, M. A., Davison, N., Rosset, P., Trichet, V., Blanchard, F., et al. (2019). Immune modulation by transplanted calcium phosphate biomaterials and human mesenchymal stromal cells in bone regeneration. Front. Immunol. 10, 663. doi:10.3389/fimmu.2019.00663
Izeia, L., Eufrasio-da-Silva, T., Dolatshahi-Pirouz, A., Ostrovidov, S., Paolone, G., Peppas, N. A., et al. (2020). Cell-laden alginate hydrogels for the treatment of diabetes. Expert Opin. Drug Deliv. 17 (8), 1113–1118. doi:10.1080/17425247.2020.1778667
Jochems, C. E., van der Valk, J. B., Stafleu, F. R., and Baumans, V. (2002). The use of fetal bovine serum: Ethical or scientific problem? Altern. Lab. Anim. 30 (2), 219–227. doi:10.1177/026119290203000208
Li, Y., Meng, H., Liu, Y., and Lee, B. P. (2015). Fibrin gel as an injectable biodegradable scaffold and cell carrier for tissue engineering. ScientificWorldJournal 2015, 685690. doi:10.1155/2015/685690
Li, Y., Rodrigues, J., and Tomás, H. (2012). Injectable and biodegradable hydrogels: Gelation, biodegradation and biomedical applications. Chem. Soc. Rev. 41 (6), 2193–2221. doi:10.1039/c1cs15203c
Lin, Y., Huang, S., Zou, R., Gao, X., Ruan, J., Weir, M. D., et al. (2019). Calcium phosphate cement scaffold with stem cell co-culture and prevascularization for dental and craniofacial bone tissue engineering. Dent. Mat. 35 (7), 1031–1041. doi:10.1016/j.dental.2019.04.009
Liu, J., Chen, W., Zhao, Z., and Xu, H. H. (2013a). Reprogramming of mesenchymal stem cells derived from iPSCs seeded on biofunctionalized calcium phosphate scaffold for bone engineering. Biomaterials 34 (32), 7862–7872. doi:10.1016/j.biomaterials.2013.07.029
Liu, J., Xu, H. H., Zhou, H., Weir, M. D., Chen, Q., and Trotman, C. A. (2013b). Human umbilical cord stem cell encapsulation in novel macroporous and injectable fibrin for muscle tissue engineering. Acta Biomater. 9 (1), 4688–4697. doi:10.1016/j.actbio.2012.08.009
Liu, X., Wang, P., Chen, W., Weir, M. D., Bao, C., and Xu, H. H. (2014). Human embryonic stem cells and macroporous calcium phosphate construct for bone regeneration in cranial defects in rats. Acta Biomater. 10 (10), 4484–4493. doi:10.1016/j.actbio.2014.06.027
Matsuya, S., Takagi, S., and Chow, L. C. (2000). Effect of mixing ratio and pH on the reaction between Ca4(PO4)2O and CaHPO4. J. Mat. Sci. Mat. Med. 11 (5), 305–311. doi:10.1023/a:1008961314500
Ogino, Y., Ayukawa, Y., Kukita, T., and Koyano, K. (2006). The contribution of platelet-derived growth factor, transforming growth factor-β1, and insulin-like growth factor-I in platelet-rich plasma to the proliferation of osteoblast-like cells. Oral Surg. Oral Med. Oral Pathology Oral Radiology Endodontology 101 (6), 724–729. doi:10.1016/j.tripleo.2005.08.016
Pal, K., Banthia, A. K., and Majumdar, D. K. (2014). Hydrogels for biomedical applications: A short review. J. Mat. Sci. Mat. Med. 25 (9), 2215. doi:10.1007/s10856-007-3145-z
Przekora, A. (2019). The summary of the most important cell-biomaterial interactions that need to be considered during in vitro biocompatibility testing of bone scaffolds for tissue engineering applications. Mater. Sci. Eng. C 97, 1036–1051. doi:10.1016/j.msec.2019.01.061
Rauch, C., Feifel, E., Amann, E. M., Spötl, H. P., Schennach, H., Pfaller, W., et al. (2011). Alternatives to the use of fetal bovine serum: Human platelet lysates as a serum substitute in cell culture media. Altex 28 (4), 305–316. doi:10.14573/altex.2011.4.305
Sánchez, A. R., Sheridan, P. J., and Kupp, L. I. (2003). Is platelet-rich plasma the perfect enhancement factor? A current review. Int. J. Oral Maxillofac. Implants 18 (1), 93–103.
Sandri, G., Bonferoni, M. C., Rossi, S., Ferrari, F., Mori, M., Cervio, M., et al. (2015). Platelet lysate embedded scaffolds for skin regeneration. Expert Opin. Drug Deliv. 12 (4), 525–545. doi:10.1517/17425247.2015.961421
Saporito, F., Baugh, L. M., Rossi, S., Bonferoni, M. C., Perotti, C., Sandri, G., et al. (2019). In situ gelling scaffolds loaded with platelet growth factors to improve cardiomyocyte survival after ischemia. ACS Biomater. Sci. Eng. 5 (1), 329–338. doi:10.1021/acsbiomaterials.8b01064
Saury, C., Lardenois, A., Schleder, C., Leroux, I., Lieubeau, B., David, L., et al. (2018). Human serum and platelet lysate are appropriate xeno-free alternatives for clinical-grade production of human MuStem cell batches. Stem Cell Res. Ther. 9 (1), 128. doi:10.1186/s13287-018-0852-y
Schallmoser, K., Bartmann, C., Rohde, E., Reinisch, A., Kashofer, K., Stadelmeyer, E., et al. (2007). Human platelet lysate can replace fetal bovine serum for clinical-scale expansion of functional mesenchymal stromal cells. Transfusion 47 (8), 1436–1446. doi:10.1111/j.1537-2995.2007.01220.x
Seo, B. M., Miura, M., Gronthos, S., Bartold, P. M., Batouli, S., Brahim, J., et al. (2004). Investigation of multipotent postnatal stem cells from human periodontal ligament. Lancet 364 (9429), 149–155. doi:10.1016/s0140-6736(04)16627-0
Simon, C. G., Guthrie, W. F., and Wang, F. W. (2004). Cell seeding into calcium phosphate cement. J. Biomed. Mat. Res. 68 (4), 628–639. doi:10.1002/jbm.a.20008
Spees, J. L., Gregory, C. A., Singh, H., Tucker, H. A., Peister, A., Lynch, P. J., et al. (2004). Internalized antigens must be removed to prepare hypoimmunogenic mesenchymal stem cells for cell and gene therapy. Mol. Ther. 9 (5), 747–756. doi:10.1016/j.ymthe.2004.02.012
Szpalski, C., Barbaro, M., Sagebin, F., and Warren, S. M. (2012a). Bone tissue engineering: Current strategies and techniques--part II: Cell types. Tissue Eng. Part B Rev. 18 (4), 258–269. doi:10.1089/ten.TEB.2011.0440
Szpalski, C., Wetterau, M., Barr, J., and Warren, S. M. (2012b). Bone tissue engineering: Current strategies and techniques--part I: Scaffolds. Tissue Eng. Part B Rev. 18 (4), 246–257. doi:10.1089/ten.TEB.2011.0427
Tonti, G. A., and Mannello, F. (2008). From bone marrow to therapeutic applications: Different behaviour and genetic/epigenetic stability during mesenchymal stem cell expansion in autologous and foetal bovine sera? Int. J. Dev. Biol. 52 (8), 1023–1032. doi:10.1387/ijdb.082725gt
van der Valk, J., Bieback, K., Buta, C., Cochrane, B., Dirks, W. G., Fu, J., et al. (2018). Fetal bovine serum (FBS): Past - present - future. ALTEX 35 (1), 99–118. doi:10.14573/altex.1705101
Wang, J., Qiao, P., Dong, L., Li, F., Xu, T., and Xie, Q. (2014). Microencapsulated rBMMSCs/calcium phosphate cement for bone formation in vivo. Biomed. Mat. Eng. 24 (1), 835–843. doi:10.3233/bme-130875
Wang, P., Song, Y., Weir, M. D., Sun, J., Zhao, L., Simon, C. G., et al. (2016). A self-setting iPSMSC-alginate-calcium phosphate paste for bone tissue engineering. Dent. Mat. 32 (2), 252–263. doi:10.1016/j.dental.2015.11.019
Wang, P., Zhao, L., Chen, W., Liu, X., Weir, M. D., and Xu, H. H. (2014). Stem cells and calcium phosphate cement scaffolds for bone regeneration. J. Dent. Res. 93 (7), 618–625. doi:10.1177/0022034514534689
Wang, X. J., Huang, H., Yang, F., Xia, L. G., Zhang, W. J., Jiang, X. Q., et al. (2011). Ectopic study of tissue-engineered bone complex with enamel matrix proteins, bone marrow stromal cells in porous calcium phosphate cement scaffolds, in nude mice. Cell Prolif. 44 (3), 274–282. doi:10.1111/j.1365-2184.2011.00750.x
Weir, M. D., and Xu, H. H. (2008). High-strength, in situ-setting calcium phosphate composite with protein release. J. Biomed. Mat. Res. A 85 (2), 388–396. doi:10.1002/jbm.a.31347
Weir, M. D., Xu, H. H., and Simon, C. G. (2006). Strong calcium phosphate cement-chitosan-mesh construct containing cell-encapsulating hydrogel beads for bone tissue engineering. J. Biomed. Mat. Res. A 77 (3), 487–496. doi:10.1002/jbm.a.30626
Wu, R. X., Yu, Y., Yin, Y., Zhang, X. Y., Gao, L. N., and Chen, F. M. (2017). Platelet lysate supports the in vitro expansion of human periodontal ligament stem cells for cytotherapeutic use. J. Tissue Eng. Regen. Med. 11 (8), 2261–2275. doi:10.1002/term.2124
Xu, H. H., Quinn, J. B., Takagi, S., and Chow, L. C. (2002). Processing and properties of strong and non-rigid calcium phosphate cement. J. Dent. Res. 81 (3), 219–224. doi:10.1177/154405910208100315
Xu, H. H., and Simon, C. G. (2005). Fast setting calcium phosphate-chitosan scaffold: Mechanical properties and biocompatibility. Biomaterials 26 (12), 1337–1348. doi:10.1016/j.biomaterials.2004.04.043
Xu, H. H., Wang, P., Wang, L., Bao, C., Chen, Q., Weir, M. D., et al. (2017). Calcium phosphate cements for bone engineering and their biological properties. Bone Res. 5, 17056. doi:10.1038/boneres.2017.56
Xu, H. H., Weir, M. D., Burguera, E. F., and Fraser, A. M. (2006). Injectable and macroporous calcium phosphate cement scaffold. Biomaterials 27 (24), 4279–4287. doi:10.1016/j.biomaterials.2006.03.001
Xu, M., Qin, M., Cheng, Y., Niu, X., Kong, J., Zhang, X., et al. (2021). Alginate microgels as delivery vehicles for cell-based therapies in tissue engineering and regenerative medicine. Carbohydr. Polym. 266, 118128. doi:10.1016/j.carbpol.2021.118128
Zhao, L., Weir, M. D., and Xu, H. H. (2010). An injectable calcium phosphate-alginate hydrogel-umbilical cord mesenchymal stem cell paste for bone tissue engineering. Biomaterials 31 (25), 6502–6510. doi:10.1016/j.biomaterials.2010.05.017
Zhao, Z., Liu, J., Schneider, A., Gao, X., Ren, K., Weir, M. D., et al. (2019). Human periodontal ligament stem cell seeding on calcium phosphate cement scaffold delivering metformin for bone tissue engineering. J. Dent. (Shiraz). 91, 103220. doi:10.1016/j.jdent.2019.103220
Keywords: periodontal ligament stem cells, calcium phosphate scaffold, alginate microbeads, osteogenic differentiation, human platelet lysate
Citation: Qiu G, Huang M, Ke D, Liu J, Weir MD, Ma T, Wang P, Oates TW, Schneider A, Xia Y, Xu HHK and Zhao L (2022) Novel injectable calcium phosphate scaffold with human periodontal ligament stem cell encapsulation in microbeads for bone regeneration. Front. Mater. 9:977853. doi: 10.3389/fmats.2022.977853
Received: 25 June 2022; Accepted: 31 October 2022;
Published: 16 November 2022.
Edited by:
Hafiz M. N. Iqbal, Monterrey Institute of Technology and Higher Education (ITESM), MexicoReviewed by:
Ciara M Murphy, Royal College of Surgeons in Ireland, IrelandTianzhu Zhang, Southeast University, China
Copyright © 2022 Qiu, Huang, Ke, Liu, Weir, Ma, Wang, Oates, Schneider, Xia, Xu and Zhao. This is an open-access article distributed under the terms of the Creative Commons Attribution License (CC BY). The use, distribution or reproduction in other forums is permitted, provided the original author(s) and the copyright owner(s) are credited and that the original publication in this journal is cited, in accordance with accepted academic practice. No use, distribution or reproduction is permitted which does not comply with these terms.
*Correspondence: Liang Zhao, bHpoYW9uZkAxMjYuY29t; Abraham Schneider, c2NobmVpZGVyNjZAdW1hcnlsYW5kLmVkdQ==; Yang Xia, eGlheWFuZ0Buam11LmVkdS5jbg==; Hockin H. K. Xu, SFh1MkB1bWFyeWxhbmQuZWR1