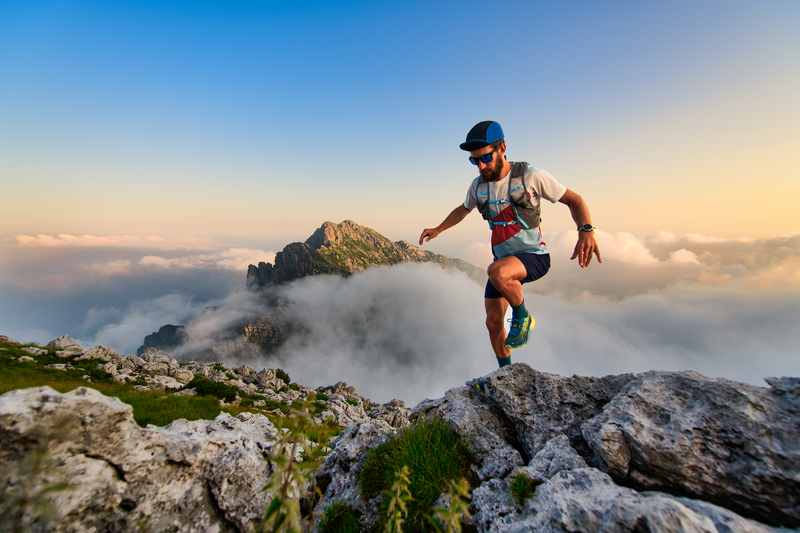
94% of researchers rate our articles as excellent or good
Learn more about the work of our research integrity team to safeguard the quality of each article we publish.
Find out more
REVIEW article
Front. Mater. , 06 July 2022
Sec. Energy Materials
Volume 9 - 2022 | https://doi.org/10.3389/fmats.2022.939173
This article is part of the Research Topic Fundamental Science on Triboelectric Nanogenerators View all 5 articles
Triboelectric nanogenerators are widely used in a variety of applications including wearable electronics, self-driven sensors, electrochemistry, and other fields. A lot of work has been done by researchers to increase the performance of triboelectric nanogenerators. Changing device structure, physical surface engineering and chemical composition modification are common effective methods. Some recent studies have found that the polarization of ferroelectric materials can regulate the output of triboelectric nanogenerators. Compared with other materials, ferroelectric materials have the advantages of polarization characteristics and large dielectric constant, which can improve the output performance by regulating the electric potential on the surface of the material, and can also collect the pyroelectric -piezoelectric-triboelectric coupling energy. However, most ferroelectric materials are rigid and therefore difficult to apply to flexible wearable electronics. In this paper, we briefly describe the mechanism of ferroelectric polarization triboelectric output and the working mechanism of coupled generators, then introduce some flexible ferroelectric materials and finally introduce some of their recent applications.
Triboelectrification effect is a kind of charged effect caused by contact, that is, in the process of triboelectric between a material and another material, one will take electrons and the other one will lost electrons, triboelectrification effect is the basic source of static charges in our life (Fan et al., 2012). The sign of a material’s charge depends on its relative polarity with the material in contact with it (Wang et al., 2013). Triboelectricity has been known for more than 2,600 years, but its effects have been largely negative (Xu et al., 2018). For example, an aircraft in flight will generate static charges due to triboelectric between the skin and the air, which will interfere with radio frequency signal transmission. In warehouses where flammable and explosive objects are stored, static charges can cause explosions and also create safety hazards. Some electronic components, such as semiconductor field-effect transistors, can be damaged by the high voltage generated by the electrostatic discharge on the gloves. Therefore, triboelectric electrification in our life is mostly regarded as a negative effect, has not been effectively used (Fan et al., 2016). Feng-Ru Fan et al. proposed the concept of triboelectric nanogenerator, which is the first time to use the triboelectric electrification effect to convert mechanical energy into electrical energy (Fan et al., 2012). The benefits of a triboelectric nanogenerator are its tiny size, light weight, and cheap cost, and it has a wide application promise in the future generation of portable wearable electronic gadgets.
With the development of research, the output performance of triboelectric nanogenerator has been greatly improved (Sahatiya et al., 2018; Zhang et al., 2021; Chai et al., 2022). Changing device structure, physical surface engineering and chemical composition modification are common effective methods (Li H. Y. et al., 2015; Shin et al., 2017; Singh and Khare, 2019). The triboelectric charge density generated between the contact surfaces of two different materials is the key factor to determine the output performance. The larger the potential difference between the contact surfaces of two materials, the easier it is to create a triboelectric charge, which boosts the output performance (Xu et al., 2018). Ferroelectric material is a kind of material with polarization orientation, which can change the polarization direction under the action of external electric field, so as to change the surface potential and improve the triboelectric output (Park et al., 2018; Zhang et al., 2021; Li B. et al., 2022). Peng Bai et al. proposed a method to regulate the output performance of triboelectric generators by polarization (Bai et al., 2014). By changing the polarization direction of PVDF, the output voltage can be increased to 240% or reduced to 70%. This paper proposes a novel way for controlling the output of triboelectric nanogenerators. Jie Wang et al. reported a method to increase triboelectric charge density. A triboelectric charge density of 1003 μC/cm2 is obtained, by coupling vacuum conditions with surface polarizing of ferroelectric materials, without the limitation of air breakdown, approaching dielectric breakdown limit (Wang et al., 2017). These works indicate the great application prospect of ferroelectric materials in triboelectric generators.
In this paper, we briefly describe the mechanism of ferroelectric polarization boosts triboelectric output and the working mechanism of coupled generators, then introduce some flexible ferroelectric materials and finally introduce some of their recent applications. We hope this paper can arouse the interest of relevant researchers.
Ferroelectric materials have the characteristics of large residual polarization, dielectric constant, pyroelectric coefficient and electro-optic modulation, and have been widely used in various fields, such as optical modulators, thermal devices, tunable microwave devices and so on (Omote et al., 1997; Trieloff et al., 2003; Acosta et al., 2017). Many research has lately shown that ferroelectric materials offer vast application potential in the realm of triboelectric generators. The output function equation of open circuit voltage (1) and short circuit current (2) of triboelectric nanogenerator is (Wang, 2017).
Where
The polarization of ferroelectric materials will change the electric potential of the material surface (Lee et al., 2016). Yoojeong Park et al. investigated the effect of polarization on surface potential (Park et al., 2020). Figure 1A shows the induced charge on surface of PVDF-TrFE non-poled, poled, and with BTO poled. The induced charge on the surface increases with the increase of polarization intensity. Figures 1B,C shows the impact of ferroelectric polarization increases the voltage and current of poled PVDF-TrFE by roughly 450 and 400%, respectively, as compared to unpoled PVDF-TrFE. The TENG results are consistent with the surface potential study. Figure 1D shows the surface potential of the poled PVDF-TrFE is greater (−2.85 V) than that of the non-poled PVDF-TrFE film (−0.65 V). The total of all dipole moments is little because the dipoles of non-poled PVDF-TrFE are randomly oriented. Deahoon Park et al. suggested a triboelectric generator (TENG) consisting of a P(VDF-TrFE) layer sandwiched by PDMS layers to increase TENG performance. (Park et al., 2021). They demonstrated that the structure and polarization orientation of PVDF-TrFE had a significant impact on the TENG’s output performance. The output performance of TENGs is improved because of the greater surface potential difference. The suggested design generates a peak output current and voltage of 50 μA/cm2 and 420 V, respectively. Lingxiao Gao et al. presented a DHS-TENG to drive temperature monitoring and smart-home management (Gao et al., 2018). Using the ferroelectric properties of PVDF, the DHS-TENG increases the triboelectric charge density of the contact area. The DHS-TENG can generate a maximum output power of 9.03 mW. It also provides improved output voltage and current of 460 V, 140 μA, with a force of 40 N, respectively. Huidrom Hemojit Singh et al. designed a PVDF-NaNbO3 film-based TENG and exhibited a great tuning (64 percent) of the TENG’s performance by modifying its electrical polarization orientation (Singh et al., 2021). Kelvin probe force microscopy experiments were performed to investigate the reason of the difference in output with polarization orientation of the films. The surface electric potential of the film was discovered to modify with polarization, leading to changes in output. The present study proved the benefit of employing ferroelectric materials for triboelectric nanogenerator systems, since the output properties of triboelectric nanogenerators can be adjusted according to the needs.
FIGURE 1. (A) Schematic of the three TENGs: non-poled PVDF-TrFE, poled PVDF-TrFE, and poled PVDF-TrFE/BTO (B) Comparison of the output voltage and (C) current of the three TENGs (D) Comparison of surface potential of three different TENGs. Reproduced under the terms of a Creative Commons CC-BY license (Park et al., 2020). Copyright 2020, America Chemical Society.
The output power of triboelectric nanogenerators has increased significantly compared to the beginning, however, there are still output power limitations for further use in large-scale energy harvesting (Bhatta et al., 2022). As a result, the coupling of triboelectric electricity with other qualities is critical, and special attention should be made to the coupling of triboelectric electricity with many other effects. (Sun et al., 2020). In daily life, there is triboelectric electricity as well as pressure and temperature changes, which often occur simultaneously. Sliding triboelectric generators, for example, often generate triboelectric heat in the triboelectric process, especially in high-frequency sliding, but it is usually lost in waste. In addition, the work done by the unavoidable forces in the vertical direction leads to additional energy loss. Therefore, it is important to collect these energies at the same time.
Ferroelectric materials have excellent piezoelectric and pyroelectric coefficients and can convert piezoelectric and thermal energy into electrical energy. Yunlong Zi et al. proposed a piezoelectric-pyroelectric-triboelectric hybrid cell for harvesting coupled energy, as shown in Figure 2A (Zi et al., 2015). Figure 2B shows that triboelectric heat and pressure are inevitably generated during the operation of a sliding triboelectric generator. Figure 2C demonstrates the operating principle of pyroelectric generators. When heated, the polarization strength of the ferroelectric material decreases and electrons run from the top electrode to the bottom to balance the bound charges on both sides, thus generating an external current, and when the heat dissipates, the polarization strength increases and electrons run from the bottom electrode and to the top, generating the opposite current. This is the pyroelectric output. Figure 2D illustrates the operating principle of a piezoelectric generator. When the applied pressure becomes smaller, the polarization intensity increases and electrons run from the bottom to the top to balance the bound charges on both sides, thus generating an external current, when the pressure becomes larger, the polarization intensity increases and electrons run from the top to the bottom, generating the opposite current. This is the piezoelectric output. Figure 2E shows the hybridization circuits for TENG and PPENG outputs. As illustrated in Figure 2F, with the LED lit by the hybridization output while the motor was on and after the motor was turned off. Figure 2G shows that there is a significant increase in output power in the coupled output state. As illustrated in Figure 2H, Shuhua Wang et al. prepared coupled generators for the collection of pyroelectric-piezoelectric-triboelectric coupling energy using PVDF/PVDF-PDMS composites (Wang S. et al., 2016). As shown in Figure 2J, the hybrid nanogenerator offers superior charging performance than the energy harvesting unit alone (TENG-PiENG or PyENG) when both TENG-PiENG and PyENG are active. Energy harvesters that are environmentally safe and have a high output performance for successfully capturing mechanical energy across a wide temperature range are desired. Sun Yanhua et al. reported a coupled generator based on BFO-BTO ceramic powder and polyimide (PI) matrix, the output voltage and current are 175 V and 0.6 μA, respectively, and output power of 4.1 mW/cm3 is achieved (Sun et al., 2020). Jihye Kim et al. proposed a P(VDF-TrFE)-based coupled generators demonstrate that the higher polarization strength significantly improves the output of the nanogenerators, the improved performance is approximately 24 percent and 82 percent in output voltage and current, respectively, compare with piezoelectric nanogenerator (Kim et al., 2017). Abu Musa Abdullah et al. reported a hybrid generator based on KNN-PVDF (Abdullah et al., 2021). The voltage and current of the hybrid piezoelectric-triboelectric output were increased by 153 and 186%, respectively, over that of piezoelectricity alone. It can charge a 100 nF capacitor to 9.4 V within 60 s. The optimal output power is 0.164 W. These works demonstrate the superiority and great potential of coupled generators for energy harvesting.
FIGURE 2. (A) Schematic diagram of the structure of the hybrid generator (B) Schematic diagram of a sliding triboelectric process. (C) Schematic of a piezoelectric output process (D) Schematic of a pyroelectric output process. (E) External circuit of the hybrid generator. (F) The hybridized output current, with the LED illuminated by the hybridization output when the motor was turned on and off, as illustrated in the insets. (G) Comparison of hybridized output and single output. Reproduced with permission (Zi et al., 2015). Copyright 2015, Wiley-VCH. (H) Device schematic. (I) Schematic diagram of device bending. (J) Hybridized output is much higher than single output. Reproduced with permission (Wang S. et al., 2016). Copyright 2016, Wiley-VCH.
Ferroelectric materials are divided into two categories: organic and inorganic. The organic ferroelectric materials mainly include PVDF, PVDF-TrFE, nylon 11, nylon 7 and so on (Chang et al., 2018; Ram et al., 2019). At present, PVDF-based materials are the most widely used materials (Yao et al., 2020). Figure 3A illustrates the molecular structure of PVDF and the different linkages, TG'TG, TTTGTTTG′, and TTTT, corresponding to the a-phase, ?-phase, and ß-phase, respectively. Among them, ß phase has the highest polarization intensity, so to improve the ferroelectric properties of PVDF, the content of ß phase should be increased (Horiuchi and Tokura, 2008). PVDF-based materials can be easily crystallized directly from melt or solution into polar ß-phase under ambient conditions by introducing a certain percentage of structurally similar molecules such as trifluoro ethylene (TrFE) (Owczarek et al., 2016). TrFE, which one of the hydrogens is substituted with a slightly larger fluorine to form a stable copolymer with VDF. Due to spatial site resistance, the TrFE defect forces the copolymer chain into an all-trans conformation, resulting in a ferroelectric ß-phase, which is the only stable crystalline phase of P(VDF-TrFE).Inorganic ferroelectric materials mainly include BTO, PZT, etc (Liu et al., 2016; Acosta et al., 2017). Their molecular structures are all chalcogenide structures, as shown in Figure 3B. They have four phase structures as cubic, tetragonal, orthorhombic and rhombohedral, where the cubic phase is non-ferroelectric and the remaining three are ferroelectric with spontaneous polarization directions along (001) (011), and (111), respectively (Martin and Rappe, 2017). The temperature at which the transition from the ferroelectric to the non-ferroelectric occurs is called the Curie temperature (Bersuker, 1981). Inorganic ferroelectric materials have large residual polarization strengths and large dielectric constants, much larger than organic ferroelectric materials, yet are mostly rigid (Tsagarakis et al., 2006). The development of the preparation process allows the preparation of inorganic ferroelectric materials on flexible substrates, such as PLD, ALD, dissolution sacrificial layer, laser lift-off (LLO), etc (Yao et al., 2020). However, the lack of flexibility and wear resistance makes it difficult to be used in triboelectric electric generators (Lan et al., 2020). Figure 3C illustrates the principle of ferroelectric material polarization, in which the ferroelectric domains appear in a disordered state when unpolarized, when the macroscopic polarization intensity of the material is zero. After the electric field is applied, the ferroelectric domains are aligned in one direction under the action of the electric field and are in a state of macroscopic polarization, with the direction of polarization along with the applied electric field. The magnitude of the applied electric field depends on the magnitude of the coercivity field of the material itself. Researchers mixed inorganic ferroelectric materials with organic ferroelectric materials to enhance the polarization strength and dielectric constant of organic ferroelectric materials. Xiang Tao et al. reported a method to increase the performance adding BTO nanoparticles into the PVDF film, with a contact force and spacing distance of 180N and 100mm, respectively (Tao et al., 2019). At a contact frequency of 5Hz, peak voltage and charge density increased by 234 percent and 130 percent, respectively. The higher piezoelectric characteristic and permittivity are important for the greatly improved output of the TENGs, according to detailed analyses of the PVDF-BTO films. Daehoon Park et al. reported a TENG by constructing BaTiO3 nanoparticles (NPs) embedded sponge structures. The BaTiO3(NPs) serve a key role in raising surface charge density via polarization strength, allowing TENGs to be packaged with a small spacing gap (Park et al., 2018). An output voltage of 370 V and current of 10 μA were obtained. Even in adverse environments, the TENGs can dependably capture energy. The method presented here is a simple, practical, and repeatable method for fabricating tiny and packed TENGs for use in mechanical energy harvesting systems. Hyun Soo Kim et al. proposed a triboelectric generator consisting of PVDF and PDMS with ITO as the electrode (Kim H. S. et al., 2019). Compared to under-polarized and unpolarized PVDF, up-polarized PVDF creates large triboelectric charges, fast saturation voltages/currents, and large residual charges due to modulated surface potential and increased capacitance. Bin Li et al. proposed a TENG by adding BNT–BS/NN–ST nano powder into PDMS (Li B. et al., 2022). In instance, we discovered that BNT–BS/NN–ST/PDMS had much greater output voltages than pure PDMS. A maximum output voltage of 370V was obtained when the addition amount was 15%. Harishkumarreddy Patnam et al. reported a composite film by adding Ca-BZT nano particles into PDMS (Patnam et al., 2020). The films’ surface unevenness and charge, and dielectric constants are all greatly improved, leads to the increase in the Hybrid generator’s output properties. The max output voltage, current, and power density values are 550 V, 34 μA, and 23.6 W/m2. Woo-Suk Jung et al. reported a coupled generator made of PVDF and PTFE (Jung et al., 2015). The coupled generator generates 370 V and 12 μA/cm2, with a corresponding output power density of 4.4 mW/cm2, it can light 600 LEDs under a force of 0.2N, and can charge a capacitor of 10 μF to 9.4 V in 25 s. This is achieved by using both piezoelectric and triboelectric processes at the same in a single cycle. In addition to inorganic ferroelectric materials, some additives can also enhance the ferroelectricity of organic ferroelectric materials. Moon Hyun Chung et al. reported a TENG made of P(VDF-TrFE) and PEDOT:PSS (Chung et al., 2022). The peak output voltage and current are ∼15 V and ∼2.3 μA, respectively, under 1 Hz oscillation. The findings show that adding PEDOT: PSS nanofiller to PVDF-TrFE improves its ferroelectric characteristics.
FIGURE 3. (A) Molecular structure of PVDF and how they are connected. Reproduced under the terms of a Creative Commons CC-BY license (Li et al., 2021). Copyright 2022, AIP Publishing LLC. (B) Schematic diagram of the structure of chalcogenide. (C) Schematic diagram of the polarization process.
By the increasing environmental problems, the search for green and renewable energy is particularly important for the sustainable development of human beings. There are four main research areas of triboelectric nanogenerators, micro and nano energy, self-driven sensing systems and blue energy, high voltage electrical power source (Wu et al., 2019). Flexible ferroelectric triboelectric generators for blue energy and high-voltage electrical power are still relatively little studied, so they are not described here. We present some generators made of flexible ferroelectric materials and compare their performance, as shown in Table 1. This section focuses on the application of flexible ferroelectric triboelectric nanogenerators to micro and nano energy and self-driven sensing systems.
In the future, everyone has several or even dozens of portable mobile electronic devices on them. Size and power supply problems limit the development of next-generation portable electronic devices, and the discarded batteries will bring pollution to the environment (Wang, 2008). As a simple and efficient alternative for converting mechanical energy into electrical energy, triboelectric nanogenerators have the potential to significantly reduce not just energy consumption but also the pollution created by discarded batteries (Kim et al., 2022). The availability, conversion efficiency, and stability of micro-nano energy are some of the most important performance factors (Fan et al., 2016). The use of solar energy is an obvious option when the device is in an open area with enough light. In situations when the device is adjacent to an engine but not illuminated, collecting energy through mechanical vibrations is the most effective method of generating power (Li H. Y. et al., 2015). In biological applications, collecting the deformation energy produced by muscle stretching is an effective method of doing this (Sim et al., 2016). Solar cells are very efficient, however when light is restricted due to environmental circumstances, high-efficiency solar cells may not be the ideal option for such devices, despite their great efficiency (Sun et al., 2020). So the sort of energy collected relies on the stability of the energy source, which is essential for the device to function well over an extended period of time (Singh and Khare, 2018). The performance of solar cells, for example, is impacted by a variety of elements including the time of day and night, meteorological conditions, and even the season. This is the reason why the triboelectric nanogenerator was selected as the energy source for the self-driven sensor and why it was utilised in the first place (Niu et al., 2013). Charge density is one of the most essential factors of TENG since it significantly influences output property, yet, it is severely limited by the phenomena of air breakdown, which occurs when the nanogenerator is exposed to air. Li Cheng et al. proposed a triboelectric nanogenerator that has a high charge density (Cheng et al., 2018). A peak triboelectric charge density of 490 μC/m2 is produced, as shown in Figure 4A. The generator is composed of two parts, the first of which contains a triboelectric layer made of PVDF and PA-6 film and the second part has two PET films. The output can be further enhanced by connecting multiple second part devices to the first SI-TENG device, as shown in Figure 4C. As introduced in Figure 4D, Minje Kim et al. described a way for successfully increasing the output current density of TENGs via the use of polarised ferroelectric polymers and MoS2 composite materials (Kim M. et al., 2019). As an additional benefit of having the right polarisation of both ferroelectric composite layers, the charge density has increased by a further factor of two. By optimising the manufacturing process, it is possible to obtain output voltages and current densities of up to 145 V and 350 mA/cm2, respectively, in the output circuit. As a result, the TENG has a high output power density of around 50 mW/cm2 as shown in Figure 4F.
FIGURE 4. (A) Schematic diagram of the generator. (B) Physical picture of the generator. (C) As the number of second parts increases, the output also increases. Reproduced under the terms of a Creative Commons CC-BY license (Cheng et al., 2018). Copyright 2018, Springer Nature. (D) Schematic diagram of generator structure. (E) Variation of output voltage and current with load resistance. (F) Comparison of the output of the two types of generators. Reproduced under the terms of a Creative Commons CC-BY license (Kim M. et al., 2019). Copyright 2019, America Chemical Society. (G) Schematic diagram of the structure of a water-drop friction generator. (H) Output Voltage and (I) Current of TENG with varying F-CNT concentrations. Reproduced under the terms of a Creative Commons CC-BY license (Lan et al., 2021). Copyright 2021, America Chemical Society.
Triboelectric nanogenerators based on flexible ferroelectric material also has important applications in agriculture (Chen et al., 2021; Li X. et al., 2022). Plants are crucial to agricultural productivity. The continual presence of abiotic stressors such as drought, salt, incidence sunlight, and temperature fluctuations, on the other hand, may possibly harm plant viability and consequently contribute to poor production (Torquebiau, 2017). As a result, effective and timely plant health monitoring has become an essential tool for increasing agricultural yields. However, sustaining power supply for these electrical devices remains a critical concern. Batteries are now mostly employed as temporary power sources (Wang, 2008). Conventional batteries must be changed or recharged on a regular basis, which may cause maintenance issues and environmental issues. As a result, it is preferable to develop maintenance-free and long-term power sources in order to build “smart agriculture” systems that run continuously. Zong-Hong Lin et al. showed that contact between water and polymer may be helpful for triboelectric nanogenerator, resulting in a novel use of triboelectric nanogenerator, particularly in liquid conditions for sensing (Lin et al., 2013). Zong-Hong Lin et al. created the water-TENG with PTFE to collect water-related energy derived from moving water and water droplets, which differs significantly from the Kelvin water dropper’s operating mechanism (Lin et al., 2014). The output of a 30 μL water drops water-TENG produce a max output voltage of 9.3 V and max output current of 17 μA. When the device is loading a 5 MΩ resistor, it produces a peak output power of 145 μW. Wanghuai Xu et al. created a device that harvests energy from impinging water droplets by combining a polytetrafluoroethylene film on an ITO substrate with an Al electrode (Xu et al., 2020) (171) Several orders of magnitude increase in the production of water droplet triboelectric electricity. These works demonstrate the great potential of water droplet triboelectric electricity. Water droplet triboelectric electricity is a significant source of triboelectric electricity in agricultural systems, both in rain and in watering, where energy may be gathered from water droplets (Chen et al., 2021)As shown in Figure 4G, Lingyi Lan et al. proposed a nanogenerator for collecting triboelectric electricity from water droplets (Lan et al., 2021). It has great output power of 330.6 μW/cm2, permeability and hydrophobicity, and it was fabricated using (PVDF-HFP) and F-CNT). The device is capable of harvesting energy during rain and watering to drive sensors that detect plant health without damaging the plant’s physiological activity. This allows the sensors used to detect the health of crops to function without additional power, eliminating the need for maintenance. Fibbers made of ferroelectric materials have a variety of application scenarios, such as wearable power supplies, motion sensors, etc (Wang et al., 2015; Sebastian et al., 2017; Eom et al., 2020). As shown in Figure 5A, S M Sohel Rana et al. reported a triboelectric generator made of PVDF-TrFE)/MXene used to harvest energy from human movement to drive small electronic devices (Rana et al., 2021). It produces the maximum output power of 4.02 W/m2 with a resistor of 4 MΩ. It can capture energy from the smallest movement of the human body, allowing it to power tiny electrical equipment in the house.
FIGURE 5. (A) Chematic diagram of the structure of the generator and its various applications. (B) Dielectric constant and (C) Charge density with varying MXene concentrations. Reproduced under the terms of a Creative Commons CC-BY license (Rana et al., 2021). Copyright 2021, America Chemical Society. (D) SEMs and schematic illustration of the PVDF-TrFE manufacturing. (E) Illustration of the triboelectrically charged PVDF-TrFE filter for enhanced trapping of PM1.0. (F) The PM1.0 filtering efficiency and QF with varying PVDF-TrFE concentrations. Reproduced with permission (Han et al., 2019). Copyright 2019, Wiley-VCH.
Air quality is currently a serious social and environmental problem, factory emissions, car exhaust pollution, etc. all bring serious challenges to air quality (Wang et al., 2022). Diseases due to air problems are on the increase year by year (Barkjohn et al., 2020). In poorer air conditions, the use of air filters has become the choice of many people (Zhang et al., 2020). As shown in Figure 5B, Kyung Seok Han makes a triboelectric generator to filter air with PVDF-TrFE nanofibers (Han et al., 2019). Following polarisation, this air filter has a PM1.0 filtering effectiveness of more than 88 percent, which improves to 94 percent after triboelectric commencement. This work provides a simple and effective way to improve the air quality at home. The triboelectric electricity generated by walking, running, and other motions in the body provides a rich source of triboelectric electricity. Static electricity on our clothing used to worry us, but today we can utilise triboelectric energy to power tiny wearable electrical devices. By making ferroelectric fibbers into textiles, energy can be collected from the body during movement. As shown in Figure 6A, Weifeng Yang et al. reports on a textile made from PVDF fibbers (Yang et al., 2019). This textile has excellent comfort properties. Under the slow movement of the human body, it can generate an output power of 5 W/cm2 to drive portable low power electronic devices. As shown in Figure 6B, Feng Jiang prepared a triboelectric generator from a fibber material made of PVDF-HFP and SEBS (Jiang et al., 2022). The fibber material has excellent comfort and stretchability. Generators made of this fibber material have an output voltage of 400 V, a current of 1.63 μA/cm2, and a power density of 2.34 W/m2, and has excellent water resistance. It can collect energy from bending, tapping, walking/holding and other actions.
FIGURE 6. (A) The e-textile offers excellent wearability and functions such as breathability, moisture permeability, and moisture wicking. Reproduced under the terms of a Creative Commons CC-BY license (Yang et al., 2019). Copyright 2019, Springer Nature. (B) Conceptual LPPS-NFC demonstrations for harvesting energy from different human movements, as well as a schematic representation of the construction process of an LPPS-NFC-based TPENG device. Reproduced with permission (Jiang et al., 2022). Copyright 2022, Wiley-VCH.
In addition to being used to harvest human motion energy, ferroelectric polymer fibbers can also be used to harvest acoustic energy. Airport noise, traffic noise, and building site noise have all been identified as issues that must be addressed. However, they may also be utilised as a source of energy. The energy created by sound is all around us, yet it is often squandered. As a result, we need a good method of collecting sound energy. Qichen Fang et al. proposed a triboelectric nanogenerator made of PVDF fibbers that can collect sound energy and sensing (Chen et al., 2019). The device generates a peak voltage of 400 V and a peak current of 170 μA/cm2. At 170 Hz and 115 dB, the device can provide an output power of 7 W/m2. This work provides a low-cost, simple and effective method for harvesting sound energy. Jiadong Liu et al. proposed a generator made of BFO-GFF/PDMS composite for collecting piezoelectric and triboelectric energy (Liu et al., 2021). The device’s maximum output voltage and current at 1 Hz are 110 V and 3.67 μA/cm2, respectively. Xingzhao Wang et al. prepared P(VDF-TrFe) nanofibers for triboelectric nanogenerator and obtained an output performance of 25 V, 98.56 μW and 1.98 mW/cm3 at a force of 5 N (Wang X. et al., 2016). These works show great promise for ferroelectric fibbers in smart textiles and wearable power.
In the future era of Internet of Everything, achieving object-to-object connectivity is the foundation of IoT intelligence. If a network of sensors were installed in every corner of the globe, a huge number of sensors would be located in every corner of the world and it's unlikely that batteries could power such a staggering number of trillions of sensors. Because people need to find the location of the battery from time to time, replace the battery and test whether the battery is working properly. In this case, a possible alternative is to harvest the energy present in the environment where the sensor is located (Wang, 2017). This is a new field. Because it translates mechanical inputs directly into electrical impulses without the need of extra transducers, the TENG offers a lot of promise in the area of active sensing and self-powered sensors. It requires simpler control circuit transistors and less standby power than conventional passive. Haptic touch sensors, acoustic sensors, motion and acceleration sensors (Zhang et al., 2017), and chemical sensors (Li Z. et al., 2015; Wen et al., 2015) are examples of related ground-breaking work.
A cochlear implant is a piece of electronic equipment that transforms external sound into electrical impulses. It repairs, enhances, and rebuilds deaf people’s hearing function. Cochlear implants have advanced fast in the last two decades, thanks to advances in high technology, going from experimental research to clinical use. Cochlear implants are being utilised as a standard therapy for severe to complete deafness all around the globe. The cochlear implant is the most successful biomedical engineering device in use today. The demand for adaptable, efficient, and self-powered cochlear implants is still pressing. As shown in Figure 7A, Jiaqi Zheng et al. proposed a cochlear implant based on the piezoelectric-triboelectric effect made of BTO and PVDF (Zheng et al., 2021). By dispersing the BTO nano powder in PVDF, a shell-core structure is formed that enhances the piezoelectric and triboelectric response. The device can produce an open circuit voltage of 15.24 V and a maximum short circuit of 9.22 mA/m2. With the improvement of living standard, people pay more and more attention to their health condition. Green, healthy life concept is deeply rooted. It is important for timely monitoring of health conditions. However, the majority of existing health monitoring devices are battery-powered and need periodic battery change, which is inconvenient. Therefore, it is particularly important to develop self-driven wearable sensors that can be used for medical health detection. As shown in Figure 7C, Kijoo Eom et al. demonstrated that PVDF-TrFE prepared on chitin film has a high degree of orientation and excellent stability beyond expectations (Eom et al., 2020). Triboelectric generators made by this method have superior performance and can detect fine pressure. They applied this device to detect the human pulse.
FIGURE 7. (A) The acoustic harvest membrane’s working mechanism schematic illustration for use as an artificial cochlea. (B) output power with different resistance. Reproduced under the terms of a Creative Commons CC-BY license (Zheng et al., 2021). Copyright 2021, America Chemical Society (C) Structure schematic and polarization orientation diagram; (D) Schematic of the device for pulse detection. Reproduced under the terms of a Creative Commons CC-BY license (Eom et al., 2020). Copyright 2020, America Chemical Society.
The skin is an organ that is wrapped on the body’s surface, in direct touch with the external environment, and has the job of protection, excretion, body temperature management, and sensing external stimuli. It is the biggest organ in the human body. Artificial electronic skin offers a broad variety of potential applications, including prosthetic limbs, robotics, mobile phones and computers touch screens, car steering wheels, medicine, and so on (Ma et al., 2017; Dolbashid et al., 2018; Rahman et al., 2020). As shown in Figure 8A, Yu Rim Lee et al. developed a sensitive artificial electronic skin inspired by Merkel cells (Lee et al., 2020). This electronic skin consists of lead-free ferroelectric material barium titanate powder and organic polymer. As shown in Figure 8B, When the finger touches and leaves the device, rising and falling currents are generated, thus enabling the perception of the sense of touch. As shown in Figure 8C, Trilochan Bhatta et al. proposed a compressible S-PVDF composite film prepared by electrostatic spinning method for electronic skin, with a power density of 13.25 W/m2 (Bhatta et al., 2022). As shown in Figure 8D, the 2*2 array made by this electronic skin can recognize different users, due to the individual differences of each person, different signal sizes are generated, the recognition accuracy rate reaches 98%. These works demonstrate the superiority and great promise of flexible ferroelectric materials in the field of self-driven artificial electronic skin. Hyeon Jun Sim et al. reported a stretchable fibber with ultra-high stability for triboelectric generators that can be stretched more than 10,000 times (Sim et al., 2016). A sensing system that is not disturbed by the surrounding environment is a key factor in achieving human-machine interaction (HMI) (Hoc, 2000). Jonghwa Park et al. proposed a sound-tactile sensor for dual-mode human-computer interaction (Park et al., 2022). The sensor is based on the triboelectric electric effect and is made of BTO nanopowder and PVDF with high sensitivity and linearity. In the presence of ambient noise interference, the sensor achieves a speech recognition reliability of 95%. They also utilized the sensor to create a smart glove that recognizes and distinguishes the texture of materials. Acceleration sensors are essential components in vibration monitoring and are used in a variety of applications including global positioning systems, biomedical devices, smart electronics, automobile safety, mechanical equipment vibration monitoring, monitoring for earthquakes, and troubleshooting (Gao and Zhang, 2010; Pang et al., 2015). In general, acceleration sensors are divided into three types: capacitive, piezoelectric, and piezoresistive. (Sobocinski et al., 2014). The piezoelectric sensors is self-powered, but its electrical output is quite modest and susceptible to external noise (Shen et al., 2016). Furthermore, capacitive and piezoresistive accelerometers are primarily fueled by traditional power supply units, limiting their possible uses (Roy et al., 2014). As a result, manufacturing acceleration sensors with big output signals and simultaneous self-powered capabilities is desirable. Binbin Zhang et al. demonstrated a self-driven acceleration sensor made of liquid mercury and PVDF nanofibers (Zhang et al., 2017). The sensor has ultra-high stability, and excellent sensitivity. The acceleration sensor detects accelerations ranging from 0 to 60 m/s2 and has an excellent sensitivity of 0.26 V·s/m2. These works demonstrate the great potential of flexible ferroelectric materials for self-driven sensing systems.
FIGURE 8. (A) Schematic diagram of the device structure corresponding to human cells. (B) PSC during a tactile stimulus. Reproduced under the terms of a Creative Commons CC-BY license (Lee et al., 2020). Copyright 2020, Springer Nature. (C) Schematic illustration of the device structure. (D) SEM of S-PVDF. (E) By merging dual functionality, the SP-HPS array-based user authentication platform for highly protected electronic access systems is created (simultaneous sensing of static and dynamic input features of the user). Reproduced with permission (Bhatta et al., 2022). Copyright 2022, Wiley-VCH.
In this paper, we demonstrate that flexible ferroelectric materials have a lot of promise in the area of triboelectric generators. The polarization-regulated triboelectric electric output is a distinct benefit of ferroelectric materials over other materials. However, if we want to make flexible ferroelectric materials for further applications in triboelectric electricity, there are still many areas that need to be enhanced. For example, the dielectric constant is relatively low, the polarization strength is substantially lower than in inorganic ceramic ferroelectric materials, and wear resistance and stretchability may still be improved. Therefore, we expect that this work will pique the attention of relevant researchers and encourage the study and development of flexible ferroelectric materials as well as the creation of novel applications.
All authors contributed to the manuscript by discussion of the results. HD and YY wrote this article.
This work was supported by the National Key R and D Project from Minister of Science and Technology in China (No. 2021YFA1201604), the National Natural Science Foundation of China (No. 52072041), and the University of Chinese Academy of Sciences (Grant No. Y8540XX2D2).
The authors declare that the research was conducted in the absence of any commercial or financial relationships that could be construed as a potential conflict of interest.
All claims expressed in this article are solely those of the authors and do not necessarily represent those of their affiliated organizations, or those of the publisher, the editors and the reviewers. Any product that may be evaluated in this article, or claim that may be made by its manufacturer, is not guaranteed or endorsed by the publisher.
Abdullah, A. M., Sadaf, M. U. K., Tasnim, F., Vasquez, H., Lozano, K., and Uddin, M. J. (2021). KNN Based Piezo-Triboelectric Lead-free Hybrid Energy Films. Nano Energy 86, 106133. doi:10.1016/j.nanoen.2021.106133
Acosta, M., Novak, N., Rojas, V., Patel, S., Vaish, R., Koruza, J., et al. (2017). BaTiO3-based Piezoelectrics: Fundamentals, Current Status, and Perspectives. Appl. Phys. Rev. 4, 041305. doi:10.1063/1.4990046
Bai, P., Zhu, G., Zhou, Y. S., Wang, S., Ma, J., Zhang, G., et al. (2014). Dipole-moment-induced Effect on Contact Electrification for Triboelectric Nanogenerators. Nano Res. 7, 990–997. doi:10.1007/s12274-014-0461-8
Barkjohn, K. K., Norris, C., Cui, X., Fang, L., He, L., Schauer, J. J., et al. (2020). Children's Microenvironmental Exposure to PM2.5 and Ozone and the Impact of Indoor Air Filtration. J. Expo. Sci. Environ. Epidemiol. 30, 971–980. doi:10.1038/s41370-020-00266-5
Bersuker, I. B. (1981). On the Foundation of the Vibronic Theory of Ferroelectricity and Structural Phase Transitions. Phase Transitions 2, 53–65. doi:10.1080/01411598108241309
Bhatta, T., Sharma, S., Shrestha, K., Shin, Y., Seonu, S., Lee, S., et al. (2022). Siloxene/PVDF Composite Nanofibrous Membrane for High‐Performance Triboelectric Nanogenerator and Self‐Powered Static and Dynamic Pressure Sensing Applications. Adv. Funct. Mater. 32, 2202145. doi:10.1002/adfm.202202145
Cao, V. A., Lee, S., Kim, M., Alam, M. M., Park, P., and Nah, J. (2020). Output Power Density Enhancement of Triboelectric Nanogenerators via Ferroelectric Polymer Composite Interfacial Layers. Nano Energy 67, 104300. doi:10.1016/j.nanoen.2019.104300
Chai, B., Shi, K., Zou, H., Jiang, P., Wu, Z., and Huang, X. (2022). Conductive Interlayer Modulated Ferroelectric Nanocomposites for High Performance Triboelectric Nanogenerator. Nano Energy 91, 106668. doi:10.1016/j.nanoen.2021.106668
Chang, L., You, L., and Wang, J. (2018). The Path to Flexible Ferroelectrics: Approaches and Progress. Jpn. J. Appl. Phys. 57, 0902A3. doi:10.7567/jjap.57.0902a3
Chen, F., Wu, Y., Ding, Z., Xia, X., Li, S., Zheng, H., et al. (2019). A Novel Triboelectric Nanogenerator Based on Electrospun Polyvinylidene Fluoride Nanofibers for Effective Acoustic Energy Harvesting and Self-Powered Multifunctional Sensing. Nano Energy 56, 241–251. doi:10.1016/j.nanoen.2018.11.041
Chen, P. F., An, J., Shu, S., Cheng, R. W., Nie, J. H., Jiang, T., et al. (2021). Super‐Durable, Low‐Wear, and High‐Performance Fur‐Brush Triboelectric Nanogenerator for Wind and Water Energy Harvesting for Smart Agriculture. Adv. Energy Mat. 11, 2003066. doi:10.1002/aenm.202003066
Cheng, L., Xu, Q., Zheng, Y., Jia, X., and Qin, Y. (2018). A Self-Improving Triboelectric Nanogenerator with Improved Charge Density and Increased Charge Accumulation Speed. Nat. Commun. 9, 3773. doi:10.1038/s41467-018-06045-z
Chung, M. H., Kim, H.-J., Yoo, S., Jeong, H., and Yoo, K.-H. (2022). Enhancement of Triboelectricity Based on Fully Organic Composite Films with a Conducting Polymer. RSC Adv. 12, 2820–2829. doi:10.1039/d1ra07408c
Dolbashid, A. S., Mokhtar, M. S., Muhamad, F., and Ibrahim, F. (2018). Potential Applications of Human Artificial Skin and Electronic Skin (E-skin): a Review. Bioinspired, Biomim. Nanobiomaterials 7, 53–64. doi:10.1680/jbibn.17.00002
Eom, K., Shin, Y.-E., Kim, J.-K., Joo, S. H., Kim, K., Kwak, S. K., et al. (2020). Tailored Poly(vinylidene Fluoride-Co-Trifluoroethylene) Crystal Orientation for a Triboelectric Nanogenerator through Epitaxial Growth on a Chitin Nanofiber Film. Nano Lett. 20, 6651–6659. doi:10.1021/acs.nanolett.0c02488
Fan, F.-R., Tian, Z.-Q., and Lin Wang, Z. (2012). Flexible Triboelectric Generator. Nano Energy 1, 328–334. doi:10.1016/j.nanoen.2012.01.004
Fan, F. R., Tang, W., and Wang, Z. L. (2016). Flexible Nanogenerators for Energy Harvesting and Self-Powered Electronics. Adv. Mat. 28, 4283–4305. doi:10.1002/adma.201504299
Gao, L., Hu, D., Qi, M., Gong, J., Zhou, H., Chen, X., et al. (2018). A Double-Helix-Structured Triboelectric Nanogenerator Enhanced with Positive Charge Traps for Self-Powered Temperature Sensing and Smart-Home Control Systems. Nanoscale 10, 19781–19790. doi:10.1039/c8nr05957h
Gao, Z., and Zhang, D. (2010). Design, Analysis and Fabrication of a Multidimensional Acceleration Sensor Based on Fully Decoupled Compliant Parallel Mechanism. Sensors Actuators A Phys. 163, 418–427. doi:10.1016/j.sna.2010.08.021
Han, K. S., Lee, S., Kim, M., Park, P., Lee, M. H., and Nah, J. (2019). Electrically Activated Ultrathin PVDF‐TrFE Air Filter for High‐Efficiency PM1.0 Filtration. Adv. Funct. Mat. 29, 1903633. doi:10.1002/adfm.201903633
Hoc, J.-M. (2000). From Human - Machine Interaction to Human - Machine Cooperation. Ergonomics 43, 833–843. doi:10.1080/001401300409044
Horiuchi, S., and Tokura, Y. (2008). Organic Ferroelectrics. Nat. Mater 7, 357–366. doi:10.1038/nmat2137
Jiang, F., Zhou, X., Lv, J., Chen, J., Chen, J., Kongcharoen, H., et al. (2022). Stretchable, Breathable, and Stable Lead-Free Perovskite/Polymer Nanofiber Composite for Hybrid Triboelectric and Piezoelectric Energy Harvesting. Adv. Mat. 34, e2200042. doi:10.1002/adma.202200042
Jung, W.-S., Kang, M.-G., Moon, H. G., Baek, S.-H., Yoon, S.-J., Wang, Z.-L., et al. (2015). High Output Piezo/triboelectric Hybrid Generator. Sci. Rep. 5, 9309. doi:10.1038/srep09309
Kim, H. S., Hur, S., Lee, D.-G., Shin, J., Qiao, H., Mun, S., et al. (2022). Ferroelectrically Augmented Contact Electrification Enables Efficient Acoustic Energy Transfer through Liquid and Solid Media. Energy Environ. Sci. 15, 1243–1255. doi:10.1039/d1ee02623b
Kim, H. S., Kim, D. Y., Kim, J. E., Kim, J. H., Kong, D. S., Murillo, G., et al. (2019a). Ferroelectric‐Polymer‐Enabled Contactless Electric Power Generation in Triboelectric Nanogenerators. Adv. Funct. Mat. 29, 1905816. doi:10.1002/adfm.201905816
Kim, J., Lee, J. H., Ryu, H., Lee, J.-H., Khan, U., Kim, H., et al. (2017). High-Performance Piezoelectric, Pyroelectric, and Triboelectric Nanogenerators Based on P(VDF-TrFE) with Controlled Crystallinity and Dipole Alignment. Adv. Funct. Mat. 27, 1700702. doi:10.1002/adfm.201700702
Kim, M., Park, D., Alam, M. M., Lee, S., Park, P., and Nah, J. (2019b). Remarkable Output Power Density Enhancement of Triboelectric Nanogenerators via Polarized Ferroelectric Polymers and Bulk MoS2 Composites. ACS Nano 13, 4640–4646. doi:10.1021/acsnano.9b00750
Lan, L., Xiong, J., Gao, D., Li, Y., Chen, J., Lv, J., et al. (2021). Breathable Nanogenerators for an On-Plant Self-Powered Sustainable Agriculture System. ACS Nano 15, 5307–5315. doi:10.1021/acsnano.0c10817
Lan, S., Pan, H., and Lin, Y.-H. (2020). Fabrication and Applications of Flexible Inorganic Ferroelectric Thin Films. Acta Phys. Sin. 69, 217708. doi:10.7498/aps.69.20201365
Lee, K. Y., Kim, S. K., Lee, J.-H., Seol, D., Gupta, M. K., Kim, Y., et al. (2016). Controllable Charge Transfer by Ferroelectric Polarization Mediated Triboelectricity. Adv. Funct. Mat. 26, 3067–3073. doi:10.1002/adfm.201505088
Lee, Y. R., Trung, T. Q., Hwang, B.-U., and Lee, N.-E. (2020). A Flexible Artificial Intrinsic-Synaptic Tactile Sensory Organ. Nat. Commun. 11, 2753. doi:10.1038/s41467-020-16606-w
Li, B., Liu, H., Sun, Y., Cao, Y., and Guo, Y. (2022a). Improved Triboelectric Performance of Polydimethylsiloxane Reinforced with Ferroelectric Composite Oxide. J. Mater Sci. Mater Electron 33, 5335–5340. doi:10.1007/s10854-022-07727-7
Li, H. Y., Su, L., Kuang, S. Y., Pan, C. F., Zhu, G., and Wang, Z. L. (2015a). Significant Enhancement of Triboelectric Charge Density by Fluorinated Surface Modification in Nanoscale for Converting Mechanical Energy. Adv. Funct. Mat. 25, 5691–5697. doi:10.1002/adfm.201502318
Li, Q., Zhao, J., He, B., and Hu, Z. (2021). Solution Processable Poly(vinylidene Fluoride)-Based Ferroelectric Polymers for Flexible Electronics. Apl. Mater. 9, 010902. doi:10.1063/5.0035539
Li, X., Cao, Y., Yu, X., Xu, Y., Yang, Y., Liu, S., et al. (2022b). Breeze-driven Triboelectric Nanogenerator for Wind Energy Harvesting and Application in Smart Agriculture. Appl. Energy 306, 117977. doi:10.1016/j.apenergy.2021.117977
Li, Z., Chen, J., Yang, J., Su, Y., Fan, X., Wu, Y., et al. (2015b). β-Cyclodextrin Enhanced Triboelectrification for Self-Powered Phenol Detection and Electrochemical Degradation. Energy Environ. Sci. 8, 887–896. doi:10.1039/c4ee03596h
Lin, Z.-H., Cheng, G., Lee, S., Pradel, K. C., and Wang, Z. L. (2014). Harvesting Water Drop Energy by a Sequential Contact-Electrification and Electrostatic-Induction Process. Adv. Mat. 26, 4690–4696. doi:10.1002/adma.201400373
Lin, Z.-H., Cheng, G., Lin, L., Lee, S., and Wang, Z. L. (2013). Water-solid Surface Contact Electrification and its Use for Harvesting Liquid-Wave Energy. Angew. Chem. Int. Ed. 52, 12545–12549. doi:10.1002/anie.201307249
Liu, J., Yu, D., Zheng, Z., Huangfu, G., and Guo, Y. (2021). Lead-Free BiFeO3 Film on Glass Fiber Fabric: Wearable Hybrid Piezoelectric-Triboelectric Nanogenerator. Ceram. Int. 47, 3573–3579. doi:10.1016/j.ceramint.2020.09.205
Liu, S., Grinberg, I., and Rappe, A. M. (2016). Intrinsic Ferroelectric Switching from First Principles. Nature 534, 360–363. doi:10.1038/nature18286
Ma, M., Zhang, Z., Liao, Q., Yi, F., Han, L., Zhang, G., et al. (2017). Self-powered Artificial Electronic Skin for High-Resolution Pressure Sensing. Nano Energy 32, 389–396. doi:10.1016/j.nanoen.2017.01.004
Martin, L. W., and Rappe, A. M. (2017). Thin-film Ferroelectric Materials and Their Applications. Nat. Rev. Mat. 2, 16087. doi:10.1038/natrevmats.2016.87
Min, G., Pullanchiyodan, A., Dahiya, A. S., Hosseini, E. S., Xu, Y., Mulvihill, D. M., et al. (2021). Ferroelectric-assisted High-Performance Triboelectric Nanogenerators Based on Electrospun P(VDF-TrFE) Composite Nanofibers with Barium Titanate Nanofillers. Nano Energy 90, 106600. doi:10.1016/j.nanoen.2021.106600
Niu, S., Wang, S., Lin, L., Liu, Y., Zhou, Y. S., Hu, Y., et al. (2013). Theoretical Study of Contact-Mode Triboelectric Nanogenerators as an Effective Power Source. Energy Environ. Sci. 6, 3576–3583. doi:10.1039/c3ee42571a
Omote, K., Ohigashi, H., and Koga, K. (1997). Temperature Dependence of Elastic, Dielectric, and Piezoelectric Properties of "single Crystalline'' Films of Vinylidene Fluoride Trifluoroethylene Copolymer. J. Appl. Phys. 81, 2760–2769. doi:10.1063/1.364300
Owczarek, M., Hujsak, K. A., Ferris, D. P., Prokofjevs, A., Majerz, I., Szklarz, P., et al. (2016). Flexible Ferroelectric Organic Crystals. Nat. Commun. 7, 13108. doi:10.1038/ncomms13108
Pang, Y. K., Li, X. H., Chen, M. X., Han, C. B., Zhang, C., and Wang, Z. L. (2015). Triboelectric Nanogenerators as a Self-Powered 3D Acceleration Sensor. ACS Appl. Mat. Interfaces 7, 19076–19082. doi:10.1021/acsami.5b04516
Park, D., Kim, M. C., Kim, M., Park, P., and Nah, J. (2021). Performance Enhancement of Flexible Polymer Triboelectric Generator through Polarization of the Embedded Ferroelectric Polymer Layer. Appl. Sci. 11, 1284. doi:10.3390/app11031284
Park, D., Shin, S.-H., Yoon, I.-J., and Nah, J. (2018). Ferroelectric Nanoparticle-Embedded Sponge Structure Triboelectric Generators. Nanotechnology 29, 185402. doi:10.1088/1361-6528/aaafa3
Park, J., Kang, D.-H., Chae, H., Ghosh, S. K., Jeong, C., Park, Y., et al. (2022). Frequency-selective Acoustic and Haptic Smart Skin for Dual-Mode Dynamic/static Human-Machine Interface. Sci. Adv. 8, eabj9220. doi:10.1126/sciadv.abj9220
Park, Y., Shin, Y.-E., Park, J., Lee, Y., Kim, M. P., Kim, Y.-R., et al. (2020). Ferroelectric Multilayer Nanocomposites with Polarization and Stress Concentration Structures for Enhanced Triboelectric Performances. ACS Nano 14, 7101–7110. doi:10.1021/acsnano.0c01865
Patnam, H., Dudem, B., Alluri, N. R., Mule, A. R., Graham, S. A., Kim, S.-J., et al. (2020). Piezo/triboelectric Hybrid Nanogenerators Based on Ca-Doped Barium Zirconate Titanate Embedded Composite Polymers for Wearable Electronics. Compos. Sci. Technol. 188, 107963. doi:10.1016/j.compscitech.2019.107963
Patnam, H., Dudem, B., Graham, S. A., and Yu, J. S. (2021). High-performance and Robust Triboelectric Nanogenerators Based on Optimal Microstructured Poly(vinyl Alcohol) and Poly(vinylidene Fluoride) Polymers for Self-Powered Electronic Applications. Energy 223, 120031. doi:10.1016/j.energy.2021.120031
Pongampai, S., Charoonsuk, T., Pinpru, N., Pulphol, P., Vittayakorn, W., Pakawanit, P., et al. (2021). Triboelectric-piezoelectric Hybrid Nanogenerator Based on BaTiO3-Nanorods/Chitosan Enhanced Output Performance with Self-Charge-Pumping System. Compos. Part B Eng. 208, 108602. doi:10.1016/j.compositesb.2020.108602
Rahman, M. A., Walia, S., Naznee, S., Taha, M., Nirantar, S., Rahman, F., et al. (2020). Artificial Somatosensors: Feedback Receptors for Electronic Skins. Adv. Intell. Syst. 2, 2000094. doi:10.1002/aisy.202000094
Ram, F., Radhakrishnan, S., Ambone, T., and Shanmuganathan, K. (2019). Highly Flexible Mechanical Energy Harvester Based on Nylon 11 Ferroelectric Nanocomposites. ACS Appl. Polym. Mat. 1, 1998–2005. doi:10.1021/acsapm.9b00246
Rana, S. M. S., Rahman, M. T., Salauddin, M., Sharma, S., Maharjan, P., Bhatta, T., et al. (2021). Electrospun PVDF-TrFE/MXene Nanofiber Mat-Based Triboelectric Nanogenerator for Smart Home Appliances. ACS Appl. Mat. Interfaces 13, 4955–4967. doi:10.1021/acsami.0c17512
Roy, A. L., Sarkar, H., Dutta, A., and Bhattacharyya, T. K. (2014). A High Precision SOI MEMS-CMOS ±4g Piezoresistive Accelerometer. Sensors Actuators A Phys. 210, 77–85. doi:10.1016/j.sna.2014.01.036
Sahatiya, P., Kannan, S., and Badhulika, S. (2018). Few Layer MoS2 and In Situ Poled PVDF Nanofibers on Low Cost Paper Substrate as High Performance Piezo-Triboelectric Hybrid Nanogenerator: Energy Harvesting from Handwriting and Human Touch. Appl. Mater. Today 13, 91–99. doi:10.1016/j.apmt.2018.08.009
Sebastian, T., Lusiola, T., and Clemens, F. (2017). Ferroelectric Hybrid Fibers to Develop Flexible Sensors for Shape Sensing of Smart Textiles and Soft Condensed Matter Bodies. Smart Mat. Struct. 26, 045003. doi:10.1088/1361-665x/aa5bd2
Shen, Z., Tan, C. Y., Yao, K., Zhang, L., and Chen, Y. F. (2016). A Miniaturized Wireless Accelerometer with Micromachined Piezoelectric Sensing Element. Sensors Actuators A Phys. 241, 113–119. doi:10.1016/j.sna.2016.02.022
Shin, S.-H., Bae, Y. E., Moon, H. K., Kim, J., Choi, S.-H., Kim, Y., et al. (2017). Formation of Triboelectric Series via Atomic-Level Surface Functionalization for Triboelectric Energy Harvesting. ACS Nano 11, 6131–6138. doi:10.1021/acsnano.7b02156
Sim, H. J., Choi, C., Kim, S. H., Kim, K. M., Lee, C. J., Kim, Y. T., et al. (2016). Stretchable Triboelectric Fiber for Self-Powered Kinematic Sensing Textile. Sci. Rep. 6, 35153. doi:10.1038/srep35153
Singh, H. H., and Khare, N. (2018). Flexible ZnO-PVDF/PTFE Based Piezo-Tribo Hybrid Nanogenerator. Nano Energy 51, 216–222. doi:10.1016/j.nanoen.2018.06.055
Singh, H. H., and Khare, N. (2019). Improved Performance of Ferroelectric Nanocomposite Flexible Film Based Triboelectric Nanogenerator by Controlling Surface Morphology, Polarizability, and Hydrophobicity. Energy 178, 765–771. doi:10.1016/j.energy.2019.04.150
Singh, H. H., Kumar, D., and Khare, N. (2021). Tuning the Performance of Ferroelectric Polymer-Based Triboelectric Nanogenerator. Appl. Phys. Lett. 119, 053901. doi:10.1063/5.0057640
Sobocinski, M., Leinonen, M., Juuti, J., Mantyniemi, N., and Jantunen, H. (2014). A Co-fired LTCC-PZT Monomorph Bridge Type Acceleration Sensor. Sensors Actuators A Phys. 216, 370–375. doi:10.1016/j.sna.2014.06.017
Soin, N., Zhao, P., Prashanthi, K., Chen, J., Ding, P., Zhou, E., et al. (2016). High Performance Triboelectric Nanogenerators Based on Phase-Inversion Piezoelectric Membranes of Poly(vinylidene Fluoride)-Zinc Stannate (PVDF-ZnSnO3) and Polyamide-6 (PA6). Nano Energy 30, 470–480. doi:10.1016/j.nanoen.2016.10.040
Sun, Y., Lu, Y., Li, X., Yu, Z., Zhang, S., Sun, H., et al. (2020). Flexible Hybrid Piezo/triboelectric Energy Harvester with High Power Density Workable at Elevated Temperatures. J. Mat. Chem. A 8, 12003–12012. doi:10.1039/d0ta04612d
Suo, G., Yu, Y., Zhang, Z., Wang, S., Zhao, P., Li, J., et al. (2016). Piezoelectric and Triboelectric Dual Effects in Mechanical-Energy Harvesting Using BaTiO3/Polydimethylsiloxane Composite Film. ACS Appl. Mat. Interfaces 8, 34335–34341. doi:10.1021/acsami.6b11108
Tao, X., Jin, H., Ma, M., Quan, L., Chen, J., Dong, S., et al. (2019). Significantly Enhanced Performance of Triboelectric Nanogenerator by Incorporating BaTiO 3 Nanoparticles in Poly(vinylidene Fluoride) Film. Phys. Status Solidi A 216, 1900068. doi:10.1002/pssa.201900068
Torquebiau, E. (2017). Climate-smart Agriculture : Pour Une Agriculture Climato-Compatible. Cah. Agric. 26, 66001. doi:10.1051/cagri/2017048
Trieloff, M., Jessberger, E. K., Herrwerth, I., Hopp, J., Fiéni, C., Ghélis, M., et al. (2003). Structure and Thermal History of the H-Chondrite Parent Asteroid Revealed by Thermochronometry. Nature 422, 502–506. doi:10.1038/nature01499
Tsagarakis, E. D., Lew, C., Thompson, M. O., and Giannelis, E. P. (2006). Nanocrystalline Barium Titanate Films on Flexible Plastic Substrates via Pulsed Laser Annealing. Appl. Phys. Lett. 89, 202910. doi:10.1063/1.2387964
Wang, H., Wu, Y., and Wang, J. (2022). Triboelectric Charging of Melt-Blown Nonwoven Filters with High Filtration Efficiency. Sci. Rep. 12, 1146. doi:10.1038/s41598-022-04838-3
Wang, J., Wu, C., Dai, Y., Zhao, Z., Wang, A., Zhang, T., et al. (2017). Achieving Ultrahigh Triboelectric Charge Density for Efficient Energy Harvesting. Nat. Commun. 8, 88. doi:10.1038/s41467-017-00131-4
Wang, S., Lin, L., Xie, Y., Jing, Q., Niu, S., and Wang, Z. L. (2013). Sliding-Triboelectric Nanogenerators Based on In-Plane Charge-Separation Mechanism. Nano Lett. 13, 2226–2233. doi:10.1021/nl400738p
Wang, S., Mu, X., Wang, X., Gu, A. Y., Wang, Z. L., and Yang, Y. (2015). Elasto-Aerodynamics-Driven Triboelectric Nanogenerator for Scavenging Air-Flow Energy. ACS Nano 9, 9554–9563. doi:10.1021/acsnano.5b04396
Wang, S., Wang, Z. L., and Yang, Y. (2016a). A One-Structure-Based Hybridized Nanogenerator for Scavenging Mechanical and Thermal Energies by Triboelectric-Piezoelectric-Pyroelectric Effects. Adv. Mat. 28, 2881–2887. doi:10.1002/adma.201505684
Wang, X., Yang, B., Liu, J., Zhu, Y., Yang, C., and He, Q. (2016b). A Flexible Triboelectric-Piezoelectric Hybrid Nanogenerator Based on P(VDF-TrFE) Nanofibers and PDMS/MWCNT for Wearable Devices. Sci. Rep. 6, 36409. doi:10.1038/srep36409
Wang, Z. L. (2017). On Maxwell's Displacement Current for Energy and Sensors: the Origin of Nanogenerators. Mater. Today 20, 74–82. doi:10.1016/j.mattod.2016.12.001
Wang, Z. L. (2008). Self-Powered Nanotech. Sci. Am. 298, 82–87. doi:10.1038/scientificamerican0108-82
Wen, Z., Chen, J., Yeh, M.-H., Guo, H., Li, Z., Fan, X., et al. (2015). Blow-driven Triboelectric Nanogenerator as an Active Alcohol Breath Analyzer. Nano Energy 16, 38–46. doi:10.1016/j.nanoen.2015.06.006
Wu, C., Wang, A. C., Ding, W., Guo, H., and Wang, Z. L. (2019). Triboelectric Nanogenerator: A Foundation of the Energy for the New Era. Adv. Energy Mat. 9, 1802906. doi:10.1002/aenm.201802906
Xia, J., Zheng, Z., and Guo, Y. (2022). Mechanically and Electrically Robust, Electro-Spun PVDF/PMMA Blend Films for Durable Triboelectric Nanogenerators. Compos. Part A Appl. Sci. Manuf. 157, 106914. doi:10.1016/j.compositesa.2022.106914
Xu, C., Zi, Y., Wang, A. C., Zou, H., Dai, Y., He, X., et al. (2018). On the Electron-Transfer Mechanism in the Contact-Electrification Effect. Adv. Mat. 30, e1706790. doi:10.1002/adma.201706790
Xu, W., Zheng, H., Liu, Y., Zhou, X., Zhang, C., Song, Y., et al. (2020). A Droplet-Based Electricity Generator with High Instantaneous Power Density. Nature 578, 392–396. doi:10.1038/s41586-020-1985-6
Yang, W., Gong, W., Hou, C., Su, Y., Guo, Y., Zhang, W., et al. (2019). All-fiber Tribo-Ferroelectric Synergistic Electronics with High Thermal-Moisture Stability and Comfortability. Nat. Commun. 10, 5541. doi:10.1038/s41467-019-13569-5
Yao, M., Cheng, Y., Zhou, Z., and Liu, M. (2020). Recent Progress on the Fabrication and Applications of Flexible Ferroelectric Devices. J. Mat. Chem. C 8, 14–27. doi:10.1039/c9tc04706a
Zhang, B., Zhang, L., Deng, W., Jin, L., Chun, F., Pan, H., et al. (2017). Self-Powered Acceleration Sensor Based on Liquid Metal Triboelectric Nanogenerator for Vibration Monitoring. ACS Nano 11, 7440–7446. doi:10.1021/acsnano.7b03818
Zhang, G.-H., Zhu, Q.-H., Zhang, L., Yong, F., Zhang, Z., Wang, S.-L., et al. (2020). High-performance Particulate Matter Including Nanoscale Particle Removal by a Self-Powered Air Filter. Nat. Commun. 11, 1653. doi:10.1038/s41467-020-15502-7
Zhang, J.-H., Zhang, Y., Sun, N., Li, Y., Du, J., Zhu, L., et al. (2021). Enhancing Output Performance of Triboelectric Nanogenerator via Large Polarization Difference Effect. Nano Energy 84, 105892. doi:10.1016/j.nanoen.2021.105892
Zheng, J., Yu, Z., Wang, Y., Fu, Y., Chen, D., and Zhou, H. (2021). Acoustic Core-Shell Resonance Harvester for Application of Artificial Cochlea Based on the Piezo-Triboelectric Effect. ACS Nano 15, 17499–17507. doi:10.1021/acsnano.1c04242
Keywords: flexible ferroelectric material, triboelectric nanogenerator, micro nano energy, self-driven sensing system, mechanical energy harvesting
Citation: Dan H, Li H and Yang Y (2022) Flexible Ferroelectric Materials-Based Triboelectric Nanogenerators for Mechanical Energy Harvesting. Front. Mater. 9:939173. doi: 10.3389/fmats.2022.939173
Received: 08 May 2022; Accepted: 30 May 2022;
Published: 06 July 2022.
Edited by:
Sihong Wang, The University of Chicago, United StatesReviewed by:
Yanchao Mao, Zhengzhou University, ChinaCopyright © 2022 Dan, Li and Yang. This is an open-access article distributed under the terms of the Creative Commons Attribution License (CC BY). The use, distribution or reproduction in other forums is permitted, provided the original author(s) and the copyright owner(s) are credited and that the original publication in this journal is cited, in accordance with accepted academic practice. No use, distribution or reproduction is permitted which does not comply with these terms.
*Correspondence: Ya Yang, eWF5YW5nQGJpbm4uY2FzLmNu
Disclaimer: All claims expressed in this article are solely those of the authors and do not necessarily represent those of their affiliated organizations, or those of the publisher, the editors and the reviewers. Any product that may be evaluated in this article or claim that may be made by its manufacturer is not guaranteed or endorsed by the publisher.
Research integrity at Frontiers
Learn more about the work of our research integrity team to safeguard the quality of each article we publish.