- Department of Orthodontics, School of Stomatology, Capital Medical University, Beijing, China
Bone tissue engineering, which involves scaffolds, growth factors, and cells, has been of great interest to treat bone defects in recent years. MicroRNAs (miRNAs or miRs) are small, single-stranded, noncoding RNAs that closely monitor and regulate the signaling pathway of osteoblast differentiation. Thus, the role of miRNAs in bone tissue engineering has attracted much attention. However, there are some problems when miRNAs are directly applied in the human body, including negative charge rejection of the cell membrane, nuclease degradation, immunotoxicity, and neurotoxicity. Therefore, it is necessary to use a suitable carrier to transfect miRNAs into cells. In contrast to viral vectors, nonviral vectors are advantageous because they are less immunogenic and toxic; they can deliver miRNAs with a higher molecular weight; and they are easier to construct and modify. This article reviews the application of different miRNAs or anti-miRNAs in bone tissue engineering and the related signaling pathways when they promote osteogenic gene expression and osteogenic differentiation of target cells. An overview of the properties of different types of nonviral miRNA-transfected biomaterials, including calcium phosphates, nanosystems, liposomes, nucleic acids, silk-based biomaterials, cell-penetrating peptides, bioactive glass, PEI, and exosomes, is also provided. In addition, the evaluations in load efficiency, release efficiency, cell uptake rate, biocompatibility, stability, and biological immunity of nonviral miRNA-transfected biomaterials are given. This article also confirms that these biomaterials stably deliver miRNA to promote osteogenic gene expression, osteogenic differentiation of target cells, and mineralization of the extracellular matrix. Because there are differences in the properties of various nonviral materials, future work will focus on identifying suitable transfection materials and improving the transfection efficiency and biocompatibility of materials.
1 Introduction
1.1 Bone Tissue Engineering
Bone defects caused by disease, trauma, or surgery are common clinical problems encountered by plastic surgeons. Severe bone defects can result in delayed bone union, leading to a high disability rate. The self-healing ability of bone defects is strong, but the self-healing of large-scale bone defects is often delayed or impossible, thereby requiring external intervention. Traditional bone defect repair methods include autogenous and allogeneic bone transplantation, but these methods have limitations. Although the former is the “gold standard” of bone transplantation, it has the disadvantages of limited materials, complications at the donor site, and the need for secondary surgery. Furthermore, the latter may lead to disease transmission, injection reactions, and poor prognosis due to its reduced osteoinduction capacity (Wang and Yeung, 2017; Han et al., 2020). Therefore, bone tissue engineering has emerged and has been developed in the past 2 decades. Bone tissue engineering involves culturing and expanding stem cells in vitro. The cells are then seeded onto a scaffold material with good biological activity and degradability, and the cells are cultivated for a period of time. Later, the scaffold with cells is transplanted to the corresponding defect site. Cells continue to grow and reproduce in vivo and secrete extracellular matrix. With the gradual degradation of the materials, the new bone tissue finally replaces the scaffold materials to repair the structure and restore the function (Qu et al., 2019). (Figure 1) As an alternative to bone defect repair, bone tissue engineering reduces the defects of autologous bone transplantation and allogeneic bone transplantation. The goal of bone tissue engineering is to employ the biochemical signaling pathway during the natural bone healing process to promote self-healing and bone regeneration to restore and maintain bone morphology and function (Arriaga et al., 2019). This technology involves the use of scaffold biomaterials and the introduction of appropriate growth factors and multipotent cells (Marew and Birhanu, 2021). Currently, through bone tissue engineering technology, many new bioactive materials and technologies have been developed, such as three-dimensional (3D)-printed scaffolds (Zhang et al., 2019) and gene delivery technology (Gantenbein et al., 2020), to reduce the shortcomings of traditional transplantation methods and improve the biocompatibility and the osteogenic, osteoconductive, and osteoinductive properties of grafts. The study of the molecular mechanism of osteogenesis induced by these biomaterials is also a hot field.
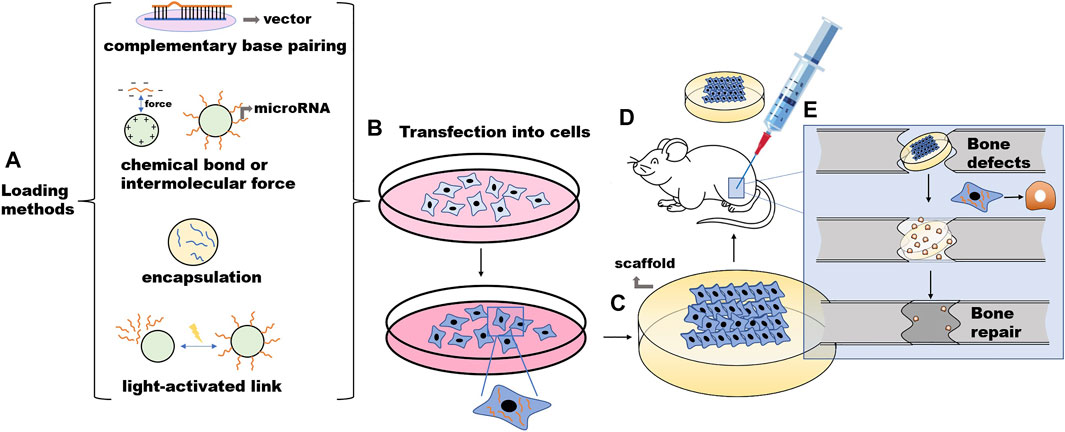
FIGURE 1. The procession of the microRNA-involved bone tissue engineering. (A) Nonviral vectors and microRNA are combined in vitro. (B) Stem cells are cultured, expanded, and transfected in vitro. (C) The cells are then seeded onto a scaffold material. (D) The scaffold with cells is transplanted to the corresponding defect site of the animal model. (E) Cells continue to grow and reproduce in vivo and secrete extracellular matrix. With the gradual degradation of the materials, the new bone tissue finally replaces the scaffold materials to repair the structure and restore the function.
1.2 miRNAs in Bone Tissue Engineering
1.2.1 Mechanism of miRNAs in Bone Tissue Engineering
Bioactive factors used in bone tissue engineering include the following three categories: 1) growth factors, 2) genetic substances, and 3) drugs (Dasari et al., 2022). The main effect of drug delivery is to resist local inflammation, to reduce the immune response, and to provide bone nutrition in bone tissue engineering. Drug delivery has a small effect on promoting osteogenesis. Growth factors have been widely used in bone tissue engineering; they bind to cell surface receptors to induce cell migration, differentiation, and proliferation, among which bone morphogenic protein (BMP) is the most commonly used (Fuerkaiti et al., 2022). However, the cost of synthesizing enough growth factors for clinical use is high, and proteolytic degradation easily occurs, resulting in a short half-life of the biological activity. Thus, the delivery of genetic material, which includes DNA and RNA, is a good alternative to growth factor delivery (Samorezov and Alsberg, 2015). This review mainly discusses the delivery of microRNAs (miRNAs or miRs).
miRNAs are small, single-stranded, noncoding RNAs that mainly exist in cells, and a small amount exist in extracellular environments, such as serum, plasma, and tears (Lanzillotti et al., 2021). MiRNAs are a class of regulators of gene expression (Reda El Sayed et al., 2021). By affecting the translation of target messenger RNAs (mRNAs), miRNAs can negatively regulate gene expression at the posttranscriptional level. miRNAs are able to bind the 3′ untranslated region (3′-UTR) of target mRNAs, which are subsequently degraded or translationally silenced, to regulate cell proliferation, differentiation and apoptosis (Figure 2) (Mazziotta et al., 2021).
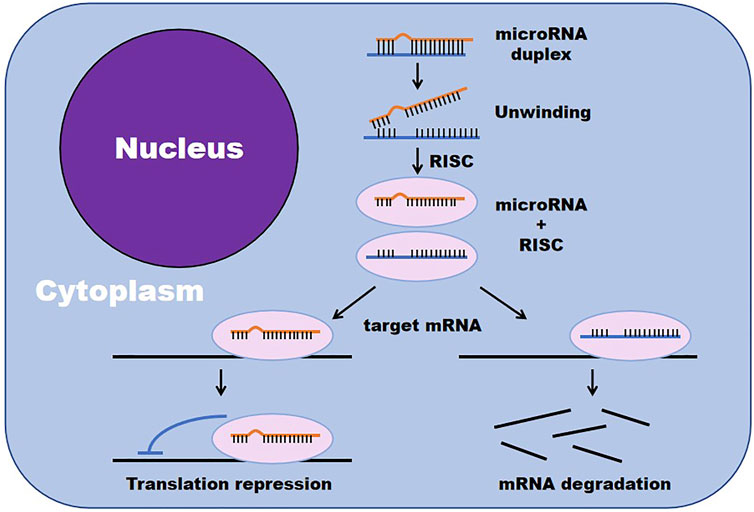
FIGURE 2. miRNAs negatively regulate gene expression by affecting the translation of target mRNAs. (RISC, RNA-induced silencing complex).
miRNAs play an important role in bone formation involving mesenchymal stem cells (MSCs) derived from blood, pericytes, and bone marrow (Iaquinta et al., 2019; Liu J. et al., 2019). miRNAs promote or inhibit osteogenic differentiation by targeting transcription factor gene expression or targeting either positive or negative regulatory genes associated with osteogenesis (Lanzillotti et al., 2021). During the process of MSC differentiation into osteoblasts, miRNAs closely monitor and regulate the following two key signaling cascades: the transforming growth factor-beta (TGF-β)/BMP pathway and the Wingless/Int-1(Wnt)/β-catenin signaling pathway (Mazziotta et al., 2021). For example, miR-93-5p suppresses osteogenic differentiation in a rabbit model of traumatic femoral head necrosis by binding the 3′-UTR of Smad5 and reducing BMP-2 and RUNX2 (Zhang Y. et al., 2021). Xu et al. confirmed that miR-889 binds the 3′UTR of WNT7A and negatively regulates the osteogenic differentiation of bone mesenchymal stem cells (BMSCs) through the Wnt/β-catenin signaling pathway (Xu et al., 2019). In addition, miR-486-3p activates the Wnt/β-catenin signaling pathway by targeting catenin beta interacting protein 1 (CTNNBIP1) to promote the osteogenesis of BMSCs, which has been confirmed in bone marrow samples from patients with osteoporosis and in mice undergoing ovariectomy (Zhang Z. et al., 2021). In addition, in other cells, such as fibroblasts, miRNAs regulate osteogenesis through similar signaling pathways. Ding et al. obtained fibroblasts from the capsular ligament of patients with ankylosing spondylitis and studied its osteogenic differentiation mechanism, and they reported that miR-214-3p targets the BMP2 gene and blocks the BMP-TGFβ axis, thereby preventing fibroblast osteogenesis (Ding et al., 2020). Apart from these two pathways, some miRNAs regulate bone differentiation through other signaling pathways, such as the Notch signaling pathway and Nrf2 pathway (Wang et al., 2013; Liu H. et al., 2019). In conclusion, the miRNAs that have been applied to bone tissue engineering and tested in vivo are listed in Table 1, and their known mechanisms of regulating osteogenic differentiation are listed in Table 2.
1.2.2 Evaluation of Osteogenic Effect of miRNA
The existing in vivo and in vitro studies have confirmed that miRNA can effectively promote bone regeneration. The main observation indexes of in vitro experiments are target mRNA and protein, Runx2, ALP, and the osteoblast-specific microRNA (Raj Preeth et al., 2021). The main indexes of in vivo experiments are as follows: significant improvement of bone volume fraction (bone volume/total volume, BV/TV), thickness of trabecularized spicules (Tb.Th), and trabecular number (Tb.N) (Kureel et al., 2017), bone mineral density (BMD) (Wu et al., 2018), bone mineral content (BMC) (Yang et al., 2019), bone surface density (bone surface/bone volume, BS/BV) (Gan et al., 2021), trabecular spacing (Tb.Sp), trabecular bone pattern factor (TbPF) (Zhou et al., 2021), and higher percentage of bone area to total area (BA/TA) (Liu H. et al., 2019).
1.3 Biomaterials for miRNA Transfection in Bone Tissue Engineering
Based on the understanding of the RNA expression profile in tissues and diseases, miRNA delivery strategies have been developed to enhance osteogenesis, such as using miRNA replacement therapy to administer double-stranded oligonucleotide miRNA mimics to treat conditions in which the target genes are overexpressed due to miRNA downregulation (Sriram et al., 2015). However, there are some difficulties when RNAs are directly applied in the human body. First, miRNA is a negatively charged molecule, which itself has difficulty penetrating the negatively charged cell membrane (Samorezov and Alsberg, 2015). Second, unmodified miRNA antagonists and miRNA mimics are rapidly degraded and eliminated in the blood circulation by nucleases that are rich in the patient bloodstream (Grixti et al., 2021). Third, miRNAs can lead to immunotoxicity by activating interferons or Toll-like receptors (Md et al., 2021) and neurotoxicity by triggering neurodegeneration through Toll-like receptors (Chen Y. et al., 2015). Therefore, it is necessary to use a suitable carrier to deliver miRNAs to protect them from inactivation in the process of matrix formation, storage, and release. The carrier should also deliver miRNA to specific tissues or organs continuously, stably and efficiently as well as ensure efficient cell uptake (Meng et al., 2016b). For most miRNA therapies developed thus far, cells are transfected or transduced with miRNAs or anti-miRNAs and loaded into scaffolds that are implanted into target sites rather than locally releasing the miRNAs/anti-miRNAs directly from scaffolds. Therefore, future research may focus on developing miR carriers to deliver miRNA/anti-miRNAs to cells in vivo (Arriaga et al., 2019).
RNA carriers are divided into viral vectors and nonviral vectors. Viral gene delivery methods present some intrinsic drawbacks, including difficult production processes (Tarach and Janaszewska, 2021), triggering acute inflammation, delayed humeral or cellular immune reactions (Levingstone et al., 2020), foreign DNA insertional mutagenesis (Dasgupta and Chatterjee, 2021), and limitation of insert molecule size (Nayerossadat et al., 2012). In contrast, nonviral vectors are advantageous due to their following properties: They are less immunogenic and toxic, they can deliver miRNAs with a higher molecular weight, and they are easier to construct and modify (Levingstone et al., 2020). The binding modes of miRNA and transfection agents (as illustrated in Table 3) mainly include electrostatic interactions (Liu et al., 2018b; Yang L. et al., 2021; Hosseinpour et al., 2021), hydrogen bonding (Meng et al., 2016b; Geng et al., 2018), polymer network wrapping (Geng et al., 2018), chemical crosslinking (Moncal et al., 2019), physical adsorption (Yu et al., 2017), and photosensitive linking (Qureshi et al., 2013; Gan et al., 2021). In addition, nonviral vectors can also transport synthetic siRNA and miRNA mimics, thus avoiding the need for nuclear localization performed according to plasmid DNA (pDNA) constructs containing RNA interference (RNAi) expression cassettes (Gantenbein et al., 2020). This effect allows siRNA/miRNA mimics to interact with the RNAi machinery directly in the cytosol, reducing the degree of intracellular trafficking required for RNAi-mediated gene repression and silencing (Levingstone et al., 2020).
To our knowledge, this article is the first review to summary and compare the characteristics of different types of nonviral miRNA-loaded biomaterials to provide examples of their application in in vitro or in vivo experiments in the future. In addition, the review confirms that nonviral miRNA vector materials can stably transmit miRNA. We also discuss the application prospects of miRNAs in bone tissue engineering.
2 Application of Different Nonviral Vectors
2.1 Calcium Phosphates as Nonviral Vectors
Calcium phosphate (CAP) has long been used as a nonviral gene delivery vector. Calcium phosphate precipitates oligonucleotides on cells, and the precipitate is adsorbed on the cell membrane. Cells take up oligonucleotides along with the natural calcium uptake (Ruedel and Bosserhoff, 2012). Among all CAP materials, hydroxyapatite (HA) remains the most frequently used CAP to date. In addition, amorphous calcium phosphate, beta tricalcium phosphate, and dicalcium phosphate dihydrate are promising (Levingstone et al., 2020). Calcium phosphate nanoparticles present good binding affinity for RNA molecules; CAP nanoparticles have good osteoinduction, osteoconduction (Levingstone et al., 2020), biocompatibility, and biodegradability, and they are nontoxic and nonimmunogenic (Bakan, 2018). Compared to cationic lipids, CAP nanoparticles show improved cytocompatibility, and spherical CAP nanoparticles increase osteoblast proliferation and osteogenic gene expression. However, the disadvantage of CAP nanoparticles is that the transfection efficiency of CAP is lower than that of viral vectors. Currently, surface functionalization, such as functionalization with cationic polymers, natural polymers, cell-penetrating peptides, biodegradable lipids, and polyethyleneimine/poly(ethylene)glycol, has been shown to improve cellular uptake and increase transfection efficiency (Levingstone et al., 2020). In addition, calcium phosphate material itself also promotes osteogenesis. Ca2+ and PO43- play an important role in regulating bone resorption and bone deposition. Ca2+ induces the chemotaxis of monocytes, osteoblasts, and hematopoietic stem cells to the injury site, and it induces osteoblast proliferation, osteoblast differentiation, and osteogenic gene expression. PO43- participates in the proliferation and differentiation of osteoblasts by entering the mitochondria and stimulating the production of adenosine triphosphate (ATP), which is converted to adenosine and promotes osteogenesis (Levingstone et al., 2020).
Irene et al. combined nanohydroxyapatite (nHA) particles with collagen-nHA scaffolds to deliver antagomiR-16 to human bone mesenchymal stem cells (hMSCs). The levels of Runx2 (the key transcription factor for osteogenesis) and osteocalcin as well as the mineral calcium deposition of hMSCs were significantly increased, indicating the bone repair potential of the combination (Mencía Castaño et al., 2019) (Figure 3). Castaño et al. implanted collagen-nanohydroxyapatite (coll-nHA) scaffolds without cells into calvarial defects of rats to deliver antagomir-133a; 1 week after implantation, antagomir-133a began to be released at the implantation site, and the bone repair volume was ten times that in the negative control group after 4 weeks, indicating that the platform accelerates bone repair in vivo without the participation of exogenous cells (Castaño et al., 2020). This system did not inoculate cells before implantation because recent studies have emphasized that adding cells to scaffolds is a limiting factor in the field of tissue engineering (Zhang et al., 2016). Coll-nHA has been demonstrated to be both a nonviral vector and a scaffold (Curtin et al., 2015).
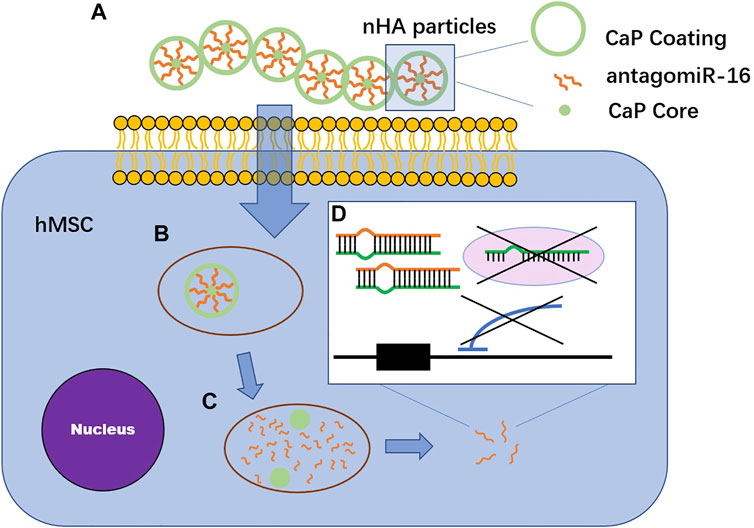
FIGURE 3. The mechanism of calcium phosphates vectors transfecting antagomiR-16. (A) AntagomiR-16 binds to nHA particles through electrostatic interaction between Ca2+ in CaP vector and phosphate groups in miRNA structure. The complex are found in multiparticulate formations. (B) nHA-antagomiR-16 particles pass through lipid bilayer cell membranes along endocytosis. (C) AntagomiR-16 undergoes endosomal escape before the fusion of endosome with lysosome. (D) AntagomiR specifically complement to their mature target miRNA, inducing the repression of miRNA, preventing translation repression or Smad5 and AcvR2a mRNA degradation via RISC.
2.2 Nanosystem as a Nonviral Vector
Nanotransfection materials mainly include nanoparticles and nanocapsules. Nanoparticles are structures with a size (1–100 nm) similar to that of biomolecules (protein, DNA, and RNA) (Rahim et al., 2018; Chiang et al., 2021). Nanoparticles covalently bind biomaterials, and the physicochemical properties of a variety of biomedical applications are met through surface modification (Kashapov et al., 2021). The types of nanoparticles commonly used for miRNA delivery applications include organic nanoparticles, such as lipid nanoparticles, as well as inorganic nanoparticles, such as metal nanoparticles and silica nanoparticles. Despite this classification, many inorganic and organic composite systems have been developed to achieve synergy (Pan et al., 2016; Kashapov et al., 2021). Because miRNAs are negatively charged, the nanoparticles are adjusted to be neutral or slightly negatively charged (Chiang et al., 2021; Kashapov et al., 2021).
Gold nanoparticles (GNPs) and silver nanoparticles (SNPs) are commonly used metal nanoparticles. In a previous study, researchers encapsulated antagomiR-31 with GNPs and delivered them to preosteoblastic and primary human mesenchymal stem cells (hMSCs) in vitro, which resulted in increased osterix protein and osteocalcin in the 2 cell types, indicating cell osteogenesis (McCully et al., 2018). Pan et al. combined polyethyleneimine (PEI)-capped gold nanoparticles with miR-29b, which effectively entered hMSCs and mouse embryonic osteoblasts (MC3T3-E1 cells), promoting the expression of alkaline phosphatase (ALP), the early-stage osteogenic gene, and medium-stage marker Runx2 as well as the expression of OCN and OPN, the late-stage osteogenic differentiation markers. Moreover, the combination showed negligible cytotoxicity (Pan et al., 2016). Moncal et al. synthesized (hydroxypropyl) cellulose (HPC)-modified SNPs and functionalized them with photolytic miR-148b. miR-148b mimics were photoactivated at wavelengths ranging from 350 to 450 nm, causing them to be released from the surface of SNPs. The proliferation rate of rat bone marrow-derived mesenchymal stem cells (rBMSCs) transfected with miR-148b was significantly higher than that of the control group. Calvarial defects in rats were almost completely repaired (Moncal et al., 2019) (Figure 4). Abu-Laban et al. cotransfected human adipose stem cells (hASCs) with SNP-miR-21 and GNP-miR-148b and activated the constructs at wavelengths of 405 and 503 nm; they reported that the degree of cell mineralization in the cotransfection group was higher than that in the group treated with one particle alone (Abu-Laban et al., 2019).
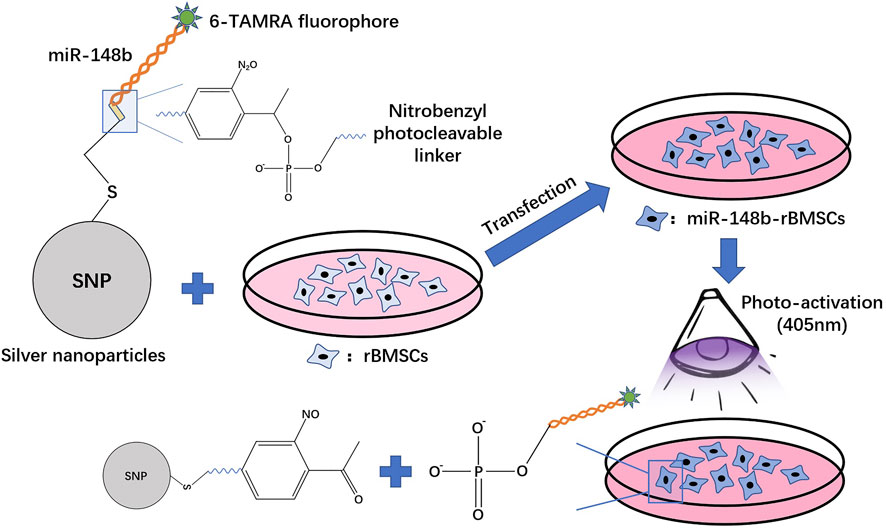
FIGURE 4. The procession of silver nanoparticles (SNPs) with a nitrobenzyl photocleavable group transfecting miR-148b into rBMSCs.
There are many other inorganic nanoparticles as well as the calcium phosphate nanoparticles mentioned above. Through appropriate synthesis and functionalization technology, inorganic nanoparticles show unique optical, magnetic, and electrical properties as well as strong loading capacity, mechanical stability, controllable size, and controllable porosity (Yang K. et al., 2021; Kashapov et al., 2021). Mesoporous silica nanoparticles (MSNs), for instance, have many favorable properties, such as low toxicity, ideal degradability, flexible design, tunable size, and high porosity. Hosseinpour et al. and Yan et al. loaded the miR-26a simulant into MSNs and delivered the complex to rBMSCs. miRNA stably bonded to the surface of nanoparticles via electrostatic attractions, and the carrier protected the miRNA from degradation by RNase A. The complex significantly enhanced osteogenic differentiation and extracellular matrix deposition and mineralization (Yan et al., 2020; Hosseinpour et al., 2021). Liu et al. constructed silica nanoparticles containing poly(lactic acid-coglycolic acid) (PLGA) microspheres (MSs). PLGA MSs release miR-10a, locally recruit T cells, and stimulate them to differentiate into Treg cells, mediating immunotherapy against bone loss in a mouse periodontitis model (Liu et al., 2018b). In addition, nanoparticles made of pinecone-like bioactive glasses have shown excellent apatite mineralization properties. After chemical modification of miR-7 to produce a miR-7-FAM complex, both the miRNA-loading efficiency and cell transfection efficiency of the complex are greater than 90% (Li X. et al., 2017).
The common organic material is chitosan, which has good aqueous solubility, good biocompatibility, controllable biodegradability, and strong bioactivity. Jiang et al. used CTH nanoparticles (chitosan solution, CS; sodium tripolyphosphate, TPP; and hyaluronic acid, HA) to transfect antagomiR-133a/b into murine BMSCs. The loading efficiency was over 90% when the N/P ratio was 15:1 and exhibited no cytotoxicity in BMSCs (Jiang et al., 2020). Chen et al. reported that approximately 30%, 55%, and 65% of agomiR-199a-5p was released within 7, 14, and 21 days, respectively, from chitosan nanoparticles, indicating that this transfection agent could continuously release miRNA in long-term culture (Chen X. et al., 2015). Another common organic material is polyethyleneimine (PEI), which is often combined with bioactive glass nanoparticles (BGNs) or MSNs. Xue et al. used monodispersed BGNs with PEI to transfect miR-5106 into BMSCs. The complex effectively protected miRNA from degradation, and more than 80% of the intact miRNA existed after 24 h of nuclease incubation compared to 35% in the Lipo group (Xue et al., 2017).
The formation of nanocapsules begins with the enrichment of monomers and crosslinkers around miRNA molecules. Monomers and crosslinkers form polymer shells around miRNA molecules through in situ polymerization, thereby forming nanocapsules (Meng et al., 2016b). Meng et al. used O-carboxymethyl chitosan (CMCS) to encapsulate miRNA-21 mimics. CMCS provides protection for the miRNA from heparin and improves transfection efficiency to 61.6% after 48 h and by 1.6-fold at 3 days, significantly promoting the osteogenic differentiation of human umbilical cord mesenchymal stem cells (HUMSCs) and bone formation (Meng et al., 2016b). Sun et al. first designed a metalloproteinase-sensitive nanocapsule, which was bound to the surface of miRNA-21 through in situ free radical polymerization; they mixed the miR-21/nanocapsule with CMCS until a gel material with good fluidity and injection was formed, and they applied the material to a rat fracture model to promote bone repair (Sun et al., 2020). Geng et al. used N-(3-aminopropyl) methylacrylamide, acrylamide, and ethylene glycol dimethacrylate nanocapsules to encapsulate miR-21 and delivered it to osteoblast-like MG63 cells, promoting the formation and mineralization of new bone (Geng et al., 2018). The nanocapsules showed more than twice the transfection capacity of commercial Lipofectamine transfectants (Liu et al., 2015).
2.3 Liposomes as Nonviral Vectors
Lipofection is an effective method to transfect miRNA into cells (Ruedel and Bosserhoff, 2012). In lipid transfection, microvesicular liposomes are formed by cationic lipids. The hydrophilic head of cationic lipids can condense with nucleic acids, and the hydrophobic tail of cationic lipids can form micelles or lipid bilayer structures arranged in spherical shell shapes to wrap the cargo in the middle (Goodwin and Huang, 2014; Carter and Shieh, 2015). When the liposome collides or attaches with the cell membrane, it fuses with the cell membrane or undergoes endocytosis to release the cargo (Carter and Shieh, 2015; Hori, 2019). A large number of commercial liposome transfection agents have been used in bone tissue engineering. It is convenient to use liposomes to study the effect of miRNA or scaffolds on osteogenesis because liposomes have good biocompatibility, and researchers can easily functionalize the surface of liposomes to carry certain targeted ligands for cell recruitment or to anchor to scaffolds (Kang et al., 2021; Scheideler et al., 2020). In addition, because of the clear transfection effect of liposomes, they are now the control group in many studies of nonviral vectors. Lipofectamine RNAi Max transfection agent has become the gold standard of miRNA nonviral vectors, and its cell transfection efficiency has reached 97% (Carthew et al., 2020). Meng et al. used the CMCS/Lipofectamine 2000 complex as the positive control of CMCS powder to deliver miR-21 to hUMSCs. The results showed that the delivery efficiency of CMCS/n (miR-21) (61.6%) was approximately 3.6 times that of the positive control group (17.2%) (Meng et al., 2016b). Other scholars have used Lipofectamine 2000 as a control to verify that there is no significant cytotoxicity of R9-LK15 nanocomplexes to cells, which is lower than lipo (Liu Q. et al., 2019). In addition, lipid vectors also include stable nuclear acid lipid particles (SNALPs), solid lipid nanoparticles (SLNs), and pH-responsive lipids. SLNs are defined as colloidal drug carriers that are 50 nm to 1 μm in diameter (Mishra and Singh, 2020). SLNs with cations condense with anionic miRNA through electrostatic interactions to form SLN/miRNA complexes (Liu et al., 2016). The other two are mainly used in delivering siRNA and drugs. At present, there is no case of delivering miRNA in bone tissue engineering using these three vectors.
2.4 Nucleic Acids as Nonviral Vectors
Transporters based on nucleic acid structures have natural advantages, including high precision brought by Watson–Crick base pairing, structural predictability, good biocompatibility, simple manufacture, and high yield (Tian et al., 2020). Li et al. made sticky-end tetrahedral framework nucleic acids (stFNAs) that carry double-stranded miRNA, including a guide chain and a passenger chain with sticky ends. miRNA and stFNAs are combined by base complementary pairing. When the complex enters the BMSCs through the cell membrane, RNase H cuts the complex to unload miRNA (Li S. et al., 2021) (Figure 5). Li et al. used tetrahedral DNA nanostructures (TDNs) to carry miR-335-5p (Li et al., 2022). TDNs have been demonstrated to have high mechanical stiffness, high stability and rich functional modification sites as well as to be able to carry siRNA, CGP, and miRNA (Zhang et al., 2018; Li et al., 2022).
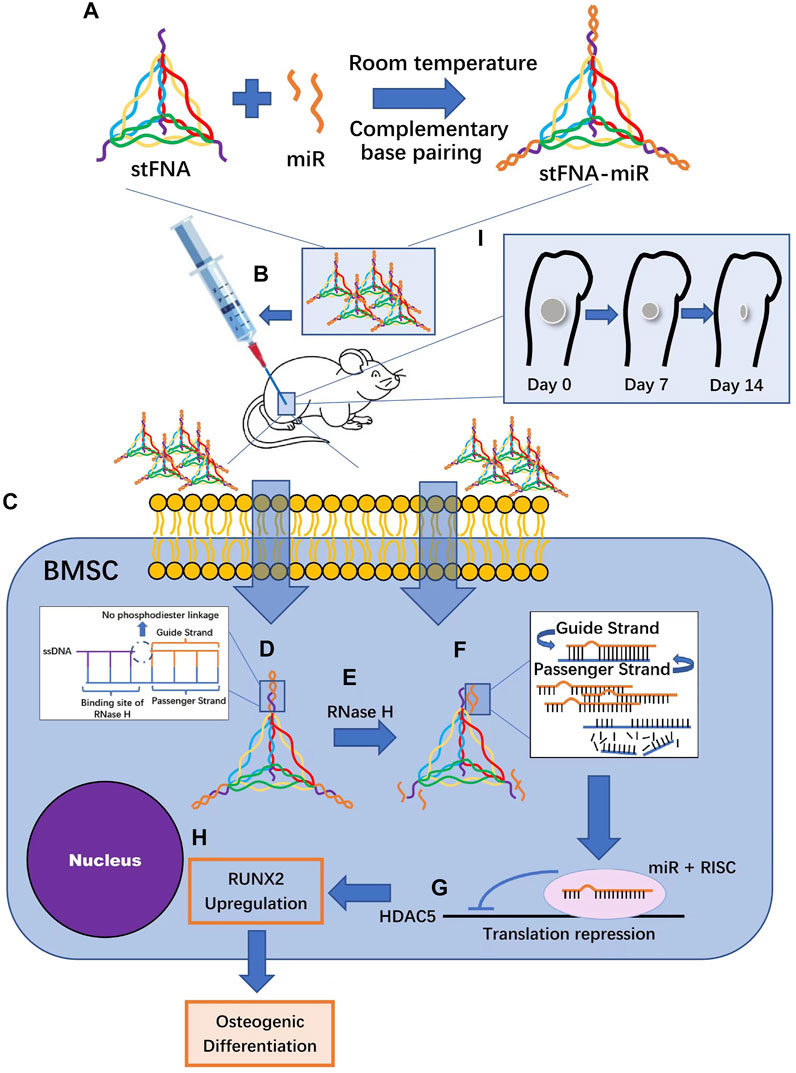
FIGURE 5. StFNA-miRs promote the healing of mice femoral defects. (A) StFNA-miRs are synthesized at room temperature. (B) The drugs are injected locally around the operation area of 1 mm diameter spherical femoral defect in mice. (C) StFNA-miRs pass through cell membranes along a caveolin-mediated pathway. (D) Double-stranded miR and stFNA form stFNA–miRs through sticky ends complementary pairing. The structures contain the binding site of RNase (H) (E) RNase H degrades the miR strands of heterozygous RNA/DNA molecules. (F) The strands that is less thermodynamically stable at the 5′ end are actively chose as the guide strands and the passenger strands undergo degradation. (G) RNA-induced silencing complex binds to target histone deacetylase 5 (HDAC5)mRNA and the translation is inhibited. (H) The expression of the runt-related transcription factor 2 (Runx2) protein is upregulated and the osteogenic differentiation of bone marrow mesenchymal stem cells (BMSCs) is ultimately promoted. (I) There is obvious bone regeneration in the bone defects of mice.
2.5 Other Types of Materials as Nonviral Vectors
Other biomaterials that can be applied for miRNA delivery include silk-based biomaterials, cell-penetrating peptides, and PEI. Silk-based bioactive materials have attracted much attention due to their good orthopedic repair ability (James et al., 2019). James et al. delivered antisense miRNA-214 (AS-miR-214), which inhibited the endogenous expression of osteoinductive antagonists via a silk-based orthopedic device. This device released miRNA continuously for 7 days, promoting osteogenic gene expression and increasing ALP levels and calcium deposition of hMSCs (James et al., 2019).
Suh et al. used a cell-penetrating peptide rich in arginine, called low molecular weight protamine (LMWP), whose transfection efficacy increased 6.5-fold that of the cationic lipids in 5 h. The LMW1/miR-29b complex enhanced the expression of ALP, OCN, OPN, and Runx2 as well as induced the differentiation of hMSCs into osteoblasts (Suh et al., 2013). Additionally, there is a new cell-penetrating peptide called R9-LK15. R9-LK15/miRNA-29b nanocomplexes maintained the stability of miR-29b in serum for up to 24 h. Moreover, the efficiency of R9-LK15 in delivering miR-29b to BMSCs was 10 times higher than that of Lipofectamine 2000. The complex promoted osteogenic differentiation and extracellular matrix mineralization of BMSCs by upregulating the expression of ALP and downregulating the expression of histone deacetylase-4 (Liu Q. et al., 2019).
PEI is also a common transfectant. PEI is a positively charged cationic polymer that combines with negatively charged miRNA through electrostatic interactions, and it promotes miRNA escape from lysosomes through the “proton sponge” effect to avoid degradation (Carthew et al., 2020; Hosseinpour et al., 2021). However, the cytotoxicity of PEI is its disadvantage. Therefore, constructing low toxicity PEI-based transport materials is a research direction (Bu et al., 2020). Lim et al. constructed ascorbic acid-PEI carbon dots (CDs) by taking advantage of the characteristics of low toxicity, high biocompatibility and chemical inertia of carbon dots, and they reported that the transfection efficiency of miR-2861 into BMSCs was 47.44% (Lim et al., 2015; Bu et al., 2020) (Figure 6). Ou et al. constructed a PEI-functionalized graphene oxide (GO) complex to transfect miR-214 inhibitor into mouse osteoblastic cells (MC3T3-E1), which did not show significant cytotoxicity (Ou et al., 2019).
Information on the transfection vectors, including loading mode, and efficiency as well as release mode, miRNA stability, biocompatibility, and cytotoxicity (Arriaga et al., 2019), is summarized in Table 3.
In addition, some materials that have not yet been used in bone tissue engineering can deliver miRNA, such as carbon nanotubes. Carbon nanotubes (CNTs) are nanomaterials whose advantages include high surface-to-volume ratios, needle-like structures, high strength, high stability, good biocompatibility, flexible interactions with carrying materials, high drug-loading capacity and ability to release drugs at specific targets. However, the disadvantages include a lack of biodegradability and toxicity (Zare et al., 2021). Andrea et al. used coated low toxicity carbon nanotubes to deliver miR-503 to mouse endothelial cells, which not only improved the stability of miR-503 but also promoted angiogenesis in vivo (Masotti et al., 2016). These studies provide ideas for the formation of vascularized bone in bone defects.
3 Other Types of Methods to Transfect miRNA Into Cells
Exosomes are lipid structural vesicles with a diameter of 50–100 nm formed by stem cells. Exosomes deliver bioactive proteins, lipids, and RNA to target cells for intercellular communication, and they have characteristics of high compatibility, low toxicity, and low immune stimulation (Chiang et al., 2021; Nan et al., 2021). Exosomes secreted by osteoblasts inhibit the differentiation of osteoclast progenitor cells (Wang Q. et al., 2021). Exosomes derived from M2 macrophages (M2D-Exos) inhibit adipogenesis and promote osteogenesis of BMSCs (Li Z. et al., 2021). Therefore, Nan et al. constructed exosomes from miRNA-378-transfected adipose-derived stem cells (ASCs) and cocultured the extracted exosomes with BMSCs and human umbilical vein endothelial cells (HUVECs). The results showed that miR-378-ASCs-Exos promoted the osteogenic differentiation of BMSCs and improved the angiogenesis of HUVECs in vitro, and they enhanced neovascularization and osteogenesis in vivo in a glucocorticoid (GC)-induced osteonecrosis of the femoral head (ONFH) rat model (Nan et al., 2021). Peng et al. extracted exosomes produced by BMSCs transfected with a miR-196a simulant or inhibitor and cocultured them with HFOB1.19 osteoblasts. The results showed that the BMSC-Exos entered HFOB1.19 cells and that exosomes overexpressing miR-196a promoted osteogenic differentiation and inhibited HFOB1.19 cell apoptosis (Peng et al., 2021). However, at present, the loading and excretion mechanism of exosomes is still unclear, and the cargo is often retained in exosomes (Chiang et al., 2021).
In addition, there are some carrier-free delivery methods. Lee et al. used a microbubble-ultrasound system. These researchers first constructed the femoral fracture in mice, and miR-29b-3p was injected through the tail vein. The probe was then immediately placed on the skin of the fracture site, and the site was irradiated with ultrasound for 4 min, which allowed the miRNA to be delivered to highly vascularized callus (Lee et al., 2016). Tu et al. injected agomiR-142-5p directly at the periosteal of the fracture site once a week for 4 weeks (Tu et al., 2017). Qin et al. isolated an extracellular vesicle (EV) delivery system from BMSCs, and the resulting EVs were rich in miR-196a, miR-27a, and miR-206. The results showed that after the EVs entered osteoblasts through endocytosis, they existed in the endoplasmic reticulum, Golgi apparatus, and lysosomes, releasing cargo along the way (Qin et al., 2016). However, the transfection efficiency and the tissue toxicity of the methods were not shown in the above studies.
4 Evaluation of Nonviral Vectors
4.1 The Physicochemical and Biological Properties of Nonviral miRNA-Transfected Biomaterials
Various physicochemical and biological properties of nonviral miRNA-transfected biomaterials deserve attention, which are necessary to promote osteogenic gene expression and osteogenic differentiation of target cells.
Most studies have reported the zeta potential of carrier materials. Zeta potential is the potential difference between the mobile dispersion medium and the fluid stationary layer attached to the dispersed particles, which can be directly measured by electromotive phenomena (Lu and Gao, 2010). Zeta potential is generally used to evaluate or predict the physical stability of particle dispersion systems (Ding et al., 2018). Generally, the higher the absolute value of zeta potential, the greater the electrostatic repulsion between particles, that is, dissolution or dispersion can resist aggregation, and the better the physical stability (Lu and Gao, 2010). It is generally believed that the zeta potential value of an electrostatically stable suspension should reach at least ±30 mV. On the other hand, low values, less than 5 mV, can cause agglomeration (Gumustas et al., 2017). In the experiment of Professor Liu (Liu et al., 2018b), a multibiological delivery vector was created to encapsulate miR-10a, which contained poly(l-lactic acid) (PLLA)/polyethylene glycol (PEG) co-functionalized mesoporous silica nanoparticles (MSN), and poly(lactic acid-co-glycolic acid) microspheres (PLGA MS). However, the zeta potential of the complex was 12 mV. Pan et al. created a PEI-capped gold nanoparticle to deliver miR-29b, and the zeta potential was +9.34 mV when the optimal w/w ratio between nanoparticles and miR-29b was selected as 3 (Pan et al., 2016). The stability of the above system deserves attention.
In terms of loading modes, nucleic acid materials combine with miRNA mainly through complementary base pairing (Li S. et al., 2021). The combination mode of calcium phosphates is electrostatic interaction (Mencía Castaño et al., 2019). Nanoparticles load miRNA through chemical bond, including coordination bond (Liu et al., 2021) and covalent bond (Abu-Laban et al., 2019), or intermolecular force, including electrostatic interaction (Yang L. et al., 2021), physical adsorption (Yu et al., 2017), or light-activated connection (Qureshi et al., 2013; Moncal et al., 2019). Nanocapsules encapsulate miRNA through electrostatic interaction, hydrogen bonding, or a network polymer formed by free radical polymerization (Liu et al., 2015; Meng et al., 2016b; Geng et al., 2018). Other types of nonviral vehicles bind miRNA mainly by electrostatic interaction, such as ascorbic Acid-PEI Carbon Dots (CD) (Bu et al., 2020), PEI-functionalized graphene oxide (GO) complex (Ou et al., 2019), silk-based orthopedic devices (James et al., 2019), low molecular weight protamine (LMWP) (Suh et al., 2013), and comb-shaped polycation (HA-SS-PGEA) consisting of hyaluronic acid (HA), disulfide groups, and ethanolamine (EA)-functionalized poly(glycidyl methacrylate) (PGMA) (Li et al., 2020).
The way for virus vectors to enter cells is gathering on the cell surface through adhesion factors, and then endocytosis is started by the real signal protein, invasion receptor (Mercer et al., 2020). Similarly, nonviral vectors carrying miRNAs enter cells mainly through endocytosis. Receptor-mediated endocytosis is currently recognized as a main way for organisms to ingest biological macromolecules. Li et al. created a unique tetrahedral DNA framework structure so that it can smoothly pass through cell membranes along caveolin-mediated endocytosis (Li S. et al., 2021). Another common endocytosis is mediated by clathrin (Bu et al., 2020). It has been reported that the pits of clathrin coating cover 2% of the plasma membrane. Because their life span is about 1 min on average, about 2% of the cell surface membrane is internalized every minute (Donaldson, 2013). It is reported that positively charged nanoparticles are generally considered to be able to electrostatically combine with anionic cell membranes to produce positive endocytosis and improve transfection efficiency (Lei et al., 2019; Yang L. et al., 2021).
For the unloading mode, nucleic acid vectors separated from miRNA by the cut of RNase H (Li S. et al., 2021). Calcium phosphates dissolve in the acidic environment of endocytic vesicles to release miRNA (Mencía Castaño et al., 2019). For nanoparticles, there are many ways to release miRNA. For some cationic nanoparticles, such as PEI nanoparticles, they first efficiently escape from the endosomes mediated by the proton sponge effect into the cytoplasm. PEI contain different types of amino groups, and their pKa values span the physiological pH range, resulting in a buffer capacity. When miR-PEI complexes are encapsulated in the membrane invagination to form endosomes, the environmental pH is in normal physiological range, so the PEI nanoparticles are inactive. However, when the endosomes are combined with lysosomes, the pH value decreases. The unsaturated amino groups on the particles chelate the protons captured by a vacuolar-type H+-ATPase (V-ATPase) proton pump, which cause lysosomes capturing a large number of protons, and Cl− and water molecules influx. Cl− and water molecules cause retention in lysosomes, causing lysosomes swelling and rupture, and the release of particles (Omote and Moriyama, 2013; Bu et al., 2020; Yang L. et al., 2021; Wang et al., 2022). When the complex is released into the cytoplasm, glutathione (GSH) can break down disulfide bonds (Lei et al., 2019), irradiation or discrete photo-trigger can release miRNA from the light-activated link (Qureshi et al., 2013; Moncal et al., 2019), photothermal release at temperature no less than 60°C or the irradiation that is about 400 nm causes the decomposition of covalent bonds (Abu-Laban et al., 2019). For nanocapsules, the acid environment decomposes the electrostatic interactions, hydrogen bonds, and the wrapping of free radical polymerization nets between the carrier material and miRNA (Liu et al., 2015).
In terms of stability, all types of carriers performed well. PBS (Lei et al., 2019), FBS (Yu et al., 2017), serum (Yang L. et al., 2021), heparin (Liu et al., 2015; Meng et al., 2016b), and nuclease (Xue et al., 2017) including RNase A (Li S. et al., 2021)are commonly used materials to detect the ability of the vectors protecting miRNA. Generally, the carriers and miRNA complex is cultured with one of the above materials for 24 h, and then the integrity of miRNA is tested to verify the ability of the vectors to protect miRNA from degradation.
4.2 Comparison of Various Nonviral miRNA-Transfected Biomaterials
For the selection of different types of miRNA vectors, many studies have utilized commercial lipid products because they have clear transfection effects; therefore, commercial lipid products are often the control group in the research of other miRNA vectors (Carthew et al., 2020). Moreover, commercial lipid products still have low toxicity to cells (Liu Q. et al., 2019).
In contrast, nucleic acid transporters have better biocompatibility. Lipofectamine 2000 was obviously toxic to BMSCs when carrying 500 nm miR, while stFNA changed cell viability only when carrying 4 times the amount of miR carried by Lipofectamine 2000 (Li S. et al., 2021). However, the transfection efficiency of nucleic acid transporters may be lower than that of other types of vectors (Li et al., 2022).
Similarly, The disadvantage of calcium phosphates is that the delivery efficiency is lower than that of Lipofectamine 2000, PEG or PEI, but calcium phosphates are low-toxicity, biodegradable and easy to use (Mencía Castaño et al., 2015).
In addition, nanoparticles and nanocapsules are currently the most studied carriers. Nanomaterials are mostly connected to miRNA through electrostatic interactions. Nanoparticles include inorganic nanoparticles and organic nanoparticles. Inorganic nanoparticles are relatively smaller. For example, the volumes of silica nanoparticles (SNs) and bioactive glass nanoparticles (BGNs) are less than one hundredth of the volume of nanohydroxyapatite (nHA) particles or Lipofectamine RNAi max (Kureel et al., 2017; Yu et al., 2017; Mencía Castaño et al., 2019). However, the release time of organic particles is relatively long, ranging from 100 h to 50 days (Chen X. et al., 2015; Wang et al., 2016; Liu et al., 2018b; Wu et al., 2018; Geng et al., 2020; Jiang et al., 2020). At the same time, nanoparticles well protect miRNA from the degradation of nuclease, serum, and heparin (Meng et al., 2016b; Yan et al., 2020; Yang L. et al., 2021). However, the transfection efficiencies of various types of nanoparticles are quite different, but the efficiencies may also be related to the different types of transfected cells and the different types of miRNA (Liu et al., 2015; Wu G. et al., 2016; Pan et al., 2016; Xue et al., 2017; Yu et al., 2017; Abu-Laban et al., 2019; Liu Q. et al., 2019).
At present, the transfection efficiencies of bioactive glass and Lipofectamine RNAi Max are the highest, more than 90% (Carthew et al., 2020). However, studies have shown that bioactive glass has cytotoxicity. When the concentration of bioactive glass was greater than 100 μg/ml, the cell morphology became irregular, and the live cell attachment was not good with concentrations greater than 240 μg/ml (Li H et al., 2017; Yu et al., 2017).
In addition, the “indirect” transfection method of extracting exosomes from miRNA-transfected cells and coculturing them with target cells seems to reduce cytotoxicity, which is an interesting new idea.
4.3 The Superiority and Insufficiency of Nonviral miRNA-Transfected Biomaterials
The performance of a carrier can be comprehensively evaluated from the aspects of load efficiency, release efficiency, cell uptake rate, biocompatibility, stability, biological immunity, manufacturing difficulty, cost, osteogenesis time, and in vivo experimental osteogenic effect. It is certain that the materials with high loading and unloading efficiency, high transfection efficiency, favorable stability, and low toxicity are the most ideal. These properties will allow miRNA to fully promote bone regeneration.
Compared to viral materials, nonviral materials have unique advantages, including low toxicity, low immunogenicity, good stability, high loading capacity, flexible design, controllable biodegradability, and relatively simple production and construction processes, and they lack the insertion mutation risk brought by viral vectors. In addition, nonviral materials are less likely to cause local acute reactions, thus allowing repeated administrations (Al-Dosari and Gao, 2009). Most importantly, various studies have shown that the application of nonviral miRNA delivery materials stably and efficiently deliver miRNAs, significantly enhancing the expression levels of osteogenic genes in target cells, the activity of osteogenic-related enzymes, the differentiation of stem cells into osteoblasts, and the deposition of calcifications, ultimately promoting osteogenesis. Some miR delivery material complexes also enhance nerve and vascular regeneration to assist bone regeneration.
However, there are still some insufficiency of nonviral miRNA-transfected biomaterials. To date, the transfection efficiency of nonviral biological delivery materials is still generally lower than that of viral vectors (Jiao et al., 2020). The reason may be that the viral vectors have adhesion factors and specific invasion receptors. The former can make the vectors gather on the cell surface, and the latter can promote endocytosis and improve the efficiency of the vectors entering the cell. Hence, it is needy to enhance the surface specificity of nonviral vectors through physical and chemical methods to increase the efficiency of cellular uptake. Second, some materials have slight toxicity to cells, tissues, and organs. It is important to reduce or even eliminate their toxicity and further improve histocompatibility. Third, the types of carriers, the types of miRNA, the types of stem cells, material concentrations, and the animal models used in different experiments are quite different, and the observation time reported in different literatures is also different. Quantitative comparison in the loading efficiency and the cell transfection efficiency between different materials is absent. Only a few experiments use commercial lipofectamine transfectants as the control group for comparison. More experiments that control the variables of miRNA, cell types, and animal models are needed to detect the transfection ability of different transfection materials. Fourth, few literatures report the reason why they chose the certain kind of vector. Different kinds of miRNA and stem cells may have their own suitable transfection materials to meet the best their unique properties, but unfortunately, there is no related discussion in the literatures. Finally, there are still some new and promising biological materials that can deliver miRNA but have not been sufficiently applied to bone tissue engineering, such as carbon nanotubes and exosomes. These new materials can be tested more for their miRNA transfection efficiency and bone regeneration effects in vivo and in vitro to develop new or compound carriers to improve the safety, efficiency, and targeting of miRNA delivery materials in bone tissue engineering.
5 Conclusion
With the development of bone tissue regeneration engineering, researchers have gradually realized that miRNAs play an important role in bone regeneration. Research on miRNA-loaded biomaterials is of great significance in bone tissue regeneration engineering because miRNA delivery materials protect miRNAs from degradation in the release process, extending the release time and making the process more controllable, stable, and efficient. Among existing delivery biomaterials, nonviral miRNA delivery materials are increasingly used in bone tissue regeneration engineering because they overcome the shortcomings of viral materials.
In short, we reviewed the properties of miRNA-transfected materials used in different bone regeneration engineering studies, including calcium phosphates, nanosystems, liposomes, nucleic acids, silk-based biomaterials, cell-penetrating peptides, bioactive glass, and PEI (Figure 7).
For the existing defects of nonviral vectors, such as relatively low transfection efficiency and the lack of quantitative comparison, future research should focus on overcoming these problems, developing new or compound carriers, improving the safety, specificity, and the transfection efficiency of the materials through physical or chemical methods, so that miRNA transfection vectors can be better used in bone tissue engineering.
Author Contributions
Conceptualization, NZ, XX, and YB; writing—original draft preparation, MZ, and YG; writing—review and editing, YG and CB; supervision, NZ; funding acquisition, NZ.
Funding
This study was supported by Beijing Municipal Education Commission (KM202010025012), Innovation Research Team Project of Beijing Stomatological Hospital, Capital Medical University (CXTD202203), Beijing Hospitals Authority’ Ascent Plan (DFL20191501), and Young Scientist Program of Beijing Stomatological Hospital Capital Medical University (YSP202001).
Conflict of Interest
The authors declare that the research was conducted in the absence of any commercial or financial relationships that could be construed as a potential conflict of interest.
Publisher’s Note
All claims expressed in this article are solely those of the authors and do not necessarily represent those of their affiliated organizations, or those of the publisher, the editors, and the reviewers. Any product that may be evaluated in this article, or claim that may be made by its manufacturer, is not guaranteed or endorsed by the publisher.
References
Abu‐Laban, M., Hamal, P., Arrizabalaga, J. H., Forghani, A., Dikkumbura, A. S., Kumal, R. R., et al. (2019). Combinatorial Delivery of miRNA‐Nanoparticle Conjugates in Human Adipose Stem Cells for Amplified Osteogenesis. Small 15 (50), 1902864. doi:10.1002/smll.201902864
Akkouch, A., Eliason, S., Sweat, M. E., Romero-Bustillos, M., Zhu, M., Qian, F., et al. (2019). Enhancement of MicroRNA-200c on Osteogenic Differentiation and Bone Regeneration by Targeting Sox2-Mediated Wnt Signaling and Klf4. Hum. Gene Ther. 30 (11), 1405–1418. doi:10.1089/hum.2019.019
Al-Dosari, M. S., and Gao, X. (2009). Nonviral Gene Delivery: Principle, Limitations, and Recent Progress. Aaps J. 11 (4), 671–681. doi:10.1208/s12248-009-9143-y
Arriaga, M. A., Ding, M. H., Gutierrez, A. S., and Chew, S. A. (2019). The Application of microRNAs in Biomaterial Scaffold‐Based Therapies for Bone Tissue Engineering. Biotechnol. J. 14 (10), 1900084. doi:10.1002/biot.201900084
Bakan, F. (2018). “Gene Delivery by Hydroxyapatite and Calcium Phosphate Nanoparticles: A Review of Novel and Recent Applications,” in Hydroxyapatite - Advances in Composite Nanomaterials, Biomedical Applications and its Technological Facets. doi:10.5772/intechopen.71062
Balagangadharan, K., Viji Chandran, S., Arumugam, B., Saravanan, S., Devanand Venkatasubbu, G., and Selvamurugan, N. (2018). Chitosan/nano-hydroxyapatite/nano-zirconium Dioxide Scaffolds with miR-590-5p for Bone Regeneration. Int. J. Biol. Macromol. 111, 953–958. doi:10.1016/j.ijbiomac.2018.01.122
Bose, S., and Tarafder, S. (2012). Calcium Phosphate Ceramic Systems in Growth Factor and Drug Delivery for Bone Tissue Engineering: a Review. Acta Biomater. 8 (4), 1401–1421. doi:10.1016/j.actbio.2011.11.017
Brenner, T., Posa-Markaryan, K., Posa-Markaryan, K., Hercher, D., Sperger, S., Heimel, P., et al. (2021). Evaluation of BMP2/miRNA Co-Expression Systems for Potent Therapeutic Efficacy in Bone-Tissue Regeneration. eCM 41, 245–268. doi:10.22203/eCM.v041a18
Bu, W., Xu, X., Wang, Z., Jin, N., Liu, L., Liu, J., et al. (2020). Ascorbic Acid-PEI Carbon Dots with Osteogenic Effects as miR-2861 Carriers to Effectively Enhance Bone Regeneration. ACS Appl. Mat. Interfaces 12 (45), 50287–50302. doi:10.1021/acsami.0c15425
Carter, M., and Shieh, J. (2015). “Gene Delivery Strategies,” in Guide to Research Techniques in Neuroscience, 239–252. doi:10.1016/b978-0-12-800511-8.00011-3
Carthew, J., Donderwinkel, I., Shrestha, S., Truong, V. X., Forsythe, J. S., and Frith, J. E. (2020). In Situ miRNA Delivery from a Hydrogel Promotes Osteogenesis of Encapsulated Mesenchymal Stromal Cells. Acta Biomater. 101, 249–261. doi:10.1016/j.actbio.2019.11.016
Castaño, I. M., Raftery, R. M., Chen, G., Cavanagh, B., Quinn, B., Duffy, G. P., et al. (2020). Rapid Bone Repair with the Recruitment Of Cd206 + M2-Like Macrophages Using Non-Viral Scaffold-Mediated Mir-133a Inhibition Of Host Cells. Acta Biomater. 109, 267–279. doi:10.1016/j.actbio.2020.03.042
Chang, C.-C., Venø, M. T., Chen, L., Ditzel, N., Le, D. Q. S., Dillschneider, P., et al. (2018). Global MicroRNA Profiling in Human Bone Marrow Skeletal-Stromal or Mesenchymal-Stem Cells Identified Candidates for Bone Regeneration. Mol. Ther. 26 (2), 593–605. doi:10.1016/j.ymthe.2017.11.018
Chen, X., Gu, S., Chen, B.-F., Shen, W.-L., Yin, Z., Xu, G.-W., et al. (2015). Nanoparticle Delivery of Stable miR-199a-5p Agomir Improves the Osteogenesis of Human Mesenchymal Stem Cells via the HIF1a Pathway. Biomaterials 53, 239–250. doi:10.1016/j.biomaterials.2015.02.071
Chen, Y., Gao, D.-Y., and Huang, L. (2015). In Vivo delivery of miRNAs for Cancer Therapy: Challenges and Strategies. Adv. Drug Deliv. Rev. 81, 128–141. doi:10.1016/j.addr.2014.05.009
Chen, M., Zhou, M., Fu, Y., Li, J., and Wang, Z. (2021). Effects of miR-672 on the Angiogenesis of Adipose-Derived Mesenchymal Stem Cells during Bone Regeneration. Stem Cell Res. Ther. 12 (1), 85. doi:10.1186/s13287-021-02154-7
Chiang, C.-L., Cheng, M.-H., and Lin, C.-H. (2021). From Nanoparticles to Cancer Nanomedicine: Old Problems with New Solutions. Nanomaterials 11 (7), 1727. doi:10.3390/nano11071727
Curtin, C. M., Tierney, E. G., McSorley, K., Cryan, S.-A., Duffy, G. P., and O'Brien, F. J. (2015). Combinatorial Gene Therapy Accelerates Bone Regeneration: Non-viral Dual Delivery of VEGF and BMP2 in a Collagen-Nanohydroxyapatite Scaffold. Adv. Healthc. Mat. 4 (2), 223–227. doi:10.1002/adhm.201400397
Dasari, A., Xue, J., and Deb, S. (2022). Magnetic Nanoparticles in Bone Tissue Engineering. Nanomaterials 12 (5), 757. doi:10.3390/nano12050757
Dasgupta, I., and Chatterjee, A. (2021). Recent Advances in miRNA Delivery Systems. Methods Protoc. 4 (1), 10. doi:10.3390/mps4010010
Delyagina, E., Li, W., Ma, N., and Steinhoff, G. (2011). Magnetic Targeting Strategies in Gene Delivery. Nanomedicine 6 (9), 1593–1604. doi:10.2217/nnm.11.143
Deng, Y., Zhou, H., Zou, D., Xie, Q., Bi, X., Gu, P., et al. (2013). The Role of miR-31-Modified Adipose Tissue-Derived Stem Cells in Repairing Rat Critical-Sized Calvarial Defects. Biomaterials 34 (28), 6717–6728. doi:10.1016/j.biomaterials.2013.05.042
Deng, Y., Bi, X., Bi, X., Zhou, H., You, Z., Wang, Y., et al. (2014). Repair of Critical-Sized Bone Defects with Anti-miR-31-expressing Bone Marrow Stromal Stem Cells and Poly(glycerol Sebacate) Scaffolds. Eur. Cell Mater 27, 13–25. doi:10.22203/ecm.v027a02
Ding, Z., Jiang, Y., and Liu, X. (2018). “Nanoemulsions-Based Drug Delivery for Brain Tumors,” in Nanotechnology-Based Targeted Drug Delivery Systems for Brain Tumors. Editors P. Kesharwani, and U. Gupta (Academic Press), 327–358. doi:10.1016/b978-0-12-812218-1.00012-9
Ding, L., Yin, Y., Hou, Y., Jiang, H., Zhang, J., Dai, Z., et al. (2020). microRNA-214-3p Suppresses Ankylosing Spondylitis Fibroblast Osteogenesis via BMP-Tgfβ Axis and BMP2. Front. Endocrinol. 11, 609753. doi:10.3389/fendo.2020.609753
Ding, R., Liu, X., Zhang, J., Yuan, J., Zheng, S., Cheng, X., et al. (2021). Downregulation of miR-1-3p Expression Inhibits the Hypertrophy and Mineralization of Chondrocytes in DDH. J. Orthop. Surg. Res. 16 (1), 512. doi:10.1186/s13018-021-02666-1
Donaldson, J. G. (2013). “Endocytosis,” in Encyclopedia of Biological Chemistry. Editors W. J. Lennarz, and M. D. Lane. Second Edition (Waltham: Academic Press), 197–199. doi:10.1016/b978-0-12-378630-2.00121-3
Fang, T., Wu, Q., Zhou, L., Mu, S., and Fu, Q. (2016). miR-106b-5p and miR-17-5p Suppress Osteogenic Differentiation by Targeting Smad5 and Inhibit Bone Formation. Exp. Cell Res. 347 (1), 74–82. doi:10.1016/j.yexcr.2016.07.010
Fuerkaiti, S. N., Çakmak, A. S., Karaaslan, C., and Gümüşderelioğlu, M. (2022). Enhanced Osteogenic Effect in Reduced BMP-2 Doses with siNoggin Transfected Pre-osteoblasts in 3D Silk Scaffolds. Int. J. Pharm. 612, 121352. doi:10.1016/j.ijpharm.2021.121352
Gan, M., Zhou, Q., Ge, J., Zhao, J., Wang, Y., Yan, Q., et al. (2021). Precise In-Situ Release of microRNA from an Injectable Hydrogel Induces Bone Regeneration. Acta Biomater. 135, 289–303. doi:10.1016/j.actbio.2021.08.041
Gantenbein, B., Tang, S., Guerrero, J., Higuita-Castro, N., Salazar-Puerta, A. I., Croft, A. S., et al. (2020). Non-viral Gene Delivery Methods for Bone and Joints. Front. Bioeng. Biotechnol. 8, 598466. doi:10.3389/fbioe.2020.598466
Geng, Z., Wang, X., Zhao, J., Li, Z., Ma, L., Zhu, S., et al. (2018). The Synergistic Effect of Strontium-Substituted Hydroxyapatite and microRNA-21 on Improving Bone Remodeling and Osseointegration. Biomater. Sci. 6 (10), 2694–2703. doi:10.1039/c8bm00716k
Geng, Z., Yu, Y., Li, Z., Ma, L., Zhu, S., Liang, Y., et al. (2020). miR-21 Promotes Osseointegration and Mineralization through Enhancing Both Osteogenic and Osteoclastic Expression. Mater. Sci. Eng. C 111, 110785. doi:10.1016/j.msec.2020.110785
Goodwin, T., and Huang, L. (2014). Nonviral Vectors. Adv. Genet. 88, 1–12. doi:10.1016/B978-0-12-800148-6.00001-8
Grixti, J. M., Ayers, D., and Day, P. J. R. (2021). An Analysis of Mechanisms for Cellular Uptake of miRNAs to Enhance Drug Delivery and Efficacy in Cancer Chemoresistance. Noncoding RNA 7 (2), 27. doi:10.3390/ncrna7020027
Gumustas, M., Sengel-Turk, C. T., Gumustas, A., Ozkan, S. A., and Uslu, B. (2017). “Effect of Polymer-Based Nanoparticles on the Assay of Antimicrobial Drug Delivery Systems,” in Multifunctional Systems for Combined Delivery, Biosensing and Diagnostics. Editor A. M. Grumezescu (Elsevier), 67–108. doi:10.1016/b978-0-323-52725-5.00005-8
Han, X., Xu, H., Che, L., Sha, D., Huang, C., Meng, T., et al. (2020). RETRACTED: Application of Inorganic Nanocomposite Hydrogels in Bone Tissue Engineering. iScience 23 (12), 101845. doi:10.1016/j.isci.2020.101845
Hong, L., Sharp, T., Khorsand, B., Fischer, C., Eliason, S., Salem, A., et al. (2016). MicroRNA-200c Represses IL-6, IL-8, and CCL-5 Expression and Enhances Osteogenic Differentiation. Plos One 11 (8), e0160915. doi:10.1371/journal.pone.0160915
Hori, M. (2019). “General Introduction,” in Plasma Medical Science, 1–4. doi:10.1016/b978-0-12-815004-7.00001-9
Hoseinzadeh, S., Atashi, A., Soleimani, M., Alizadeh, E., and Zarghami, N. (2016). MiR-221-inhibited Adipose Tissue-Derived Mesenchymal Stem Cells Bioengineered in a Nano-Hydroxy Apatite Scaffold. Vitro Cell.Dev.Biol.-Animal 52 (4), 479–487. doi:10.1007/s11626-015-9992-x
Hosseinpour, S., Cao, Y., Liu, J., Xu, C., and Walsh, L. J. (2021). Efficient Transfection and Long-Term Stability of Rno-miRNA-26a-5p for Osteogenic Differentiation by Large Pore Sized Mesoporous Silica Nanoparticles. J. Mat. Chem. B 9 (9), 2275–2284. doi:10.1039/d0tb02756a
Hou, Y., Lin, W., Li, Y., Sun, Y., Liu, Y., Chen, C., et al. (2021). De-osteogenic-differentiated Mesenchymal Stem Cells Accelerate Fracture Healing by Mir-92b. J. Orthop. Transl. 27, 25–32. doi:10.1016/j.jot.2020.10.009
Huynh, C. T., Nguyen, M. K., Naris, M., Tonga, G. Y., Rotello, V. M., and Alsberg, E. (2016). Light-triggered RNA Release and Induction of hMSC Osteogenesis via Photodegradable, Dual-Crosslinked Hydrogels. Nanomedicine 11 (12), 1535–1550. doi:10.2217/nnm-2016-0088
Iaquinta, M. R., Mazzoni, E., Bononi, I., Rotondo, J. C., Mazziotta, C., Montesi, M., et al. (2019). Adult Stem Cells for Bone Regeneration and Repair. Front. Cell Dev. Biol. 7, 268. doi:10.3389/fcell.2019.00268
James, E. N., Delany, A. M., and Nair, L. S. (2014). Post-Transcriptional Regulation in Osteoblasts Using Localized Delivery of miR-29a Inhibitor from Nanofibers to Enhance Extracellular Matrix Deposition. Acta Biomater. 10 (8), 3571–3580. doi:10.1016/j.actbio.2014.04.026
James, E. N., Van Doren, E., Li, C., and Kaplan, D. L. (2019). Silk Biomaterials-Mediated miRNA Functionalized Orthopedic Devices. Tissue Eng. Part A 25 (1-2), 12–23. doi:10.1089/ten.TEA.2017.0455
Jiang, F., Yin, F., Lin, Y., Xia, W., Zhou, L., Pan, C., et al. (2020). The Promotion of Bone Regeneration through CS/GP-CTH/antagomir-133a/b Sustained Release System. Nanomedicine Nanotechnol. Biol. Med. 24, 102116. doi:10.1016/j.nano.2019.102116
Jiang, W., Zhu, P., Zhang, T., Liao, F., Yu, Y., Liu, Y., et al. (2021). MicroRNA-205 Mediates Endothelial Progenitor Functions in Distraction Osteogenesis by Targeting the Transcription Regulator NOTCH2. Stem Cell Res. Ther. 12 (1), 101. doi:10.1186/s13287-021-02150-x
Jiao, Y., Xia, Z. L., Ze, L. J., Jing, H., Xin, B., and Fu, S. (2020). Research Progress of Nucleic Acid Delivery Vectors for Gene Therapy. Biomed. Microdevices 22 (1), 16. doi:10.1007/s10544-020-0469-7
Kang, M., Lee, C.-S., and Lee, M. (2021). Bioactive Scaffolds Integrated with Liposomal or Extracellular Vesicles for Bone Regeneration. Bioengineering 8 (10), 137. doi:10.3390/bioengineering8100137
Kashapov, R., Ibragimova, A., Pavlov, R., Gabdrakhmanov, D., Kashapova, N., Burilova, E., et al. (2021). Nanocarriers for Biomedicine: From Lipid Formulations to Inorganic and Hybrid Nanoparticles. Int. J. Mol. Sci. 22 (13), 7055. doi:10.3390/ijms22137055
Kureel, J., John, A. A., Dixit, M., and Singh, D. (2017). MicroRNA-467g Inhibits New Bone Regeneration by Targeting Ihh/Runx-2 Signaling. Int. J. Biochem. Cell Biol. 85, 35–43. doi:10.1016/j.biocel.2017.01.018
Lanzillotti, C., De Mattei, M., Mazziotta, C., Taraballi, F., Rotondo, J. C., Tognon, M., et al. (2021). Long Non-coding RNAs and MicroRNAs Interplay in Osteogenic Differentiation of Mesenchymal Stem Cells. Front. Cell Dev. Biol. 9, 646032. doi:10.3389/fcell.2021.646032
Lee, W. Y., Li, N., Lin, S., Wang, B., Lan, H. Y., and Li, G. (2016). miRNA-29b Improves Bone Healing in Mouse Fracture Model. Mol. Cell. Endocrinol. 430, 97–107. doi:10.1016/j.mce.2016.04.014
Lei, L., Liu, Z., Yuan, P., Jin, R., Wang, X., Jiang, T., et al. (2019). Injectable Colloidal Hydrogel with Mesoporous Silica Nanoparticles for Sustained Co-release of microRNA-222 and Aspirin to Achieve Innervated Bone Regeneration in Rat Mandibular Defects. J. Mat. Chem. B 7 (16), 2722–2735. doi:10.1039/c9tb00025a
Levingstone, T. J., Herbaj, S., Redmond, J., McCarthy, H. O., and Dunne, N. J. (2020). Calcium Phosphate Nanoparticles-Based Systems for RNAi Delivery: Applications in Bone Tissue Regeneration. Nanomaterials 10 (1), 146. doi:10.3390/nano10010146
Li, Y., Fan, L., Liu, S., Liu, W., Zhang, H., Zhou, T., et al. (2013). The Promotion of Bone Regeneration through Positive Regulation of Angiogenic-Osteogenic Coupling Using microRNA-26a. Biomaterials 34 (21), 5048–5058. doi:10.1016/j.biomaterials.2013.03.052
Li, H., Liu, P., Xu, S., Li, Y., Dekker, J. D., Li, B., et al. (2017). FOXP1 Controls Mesenchymal Stem Cell Commitment and Senescence during Skeletal Aging. J. Clin. Invest 127 (4), 1241–1253. doi:10.1172/jci89511
Li, K.-C., Lo, S.-C., Sung, L.-Y., Liao, Y.-H., Chang, Y.-H., and Hu, Y.-C. (2017). Improved Calvarial Bone Repair by hASCs Engineered with Cre/loxP-Based Baculovirus Conferring Prolonged BMP-2 and MiR-148b Co-expression. J. Tissue Eng. Regen. Med. 11 (11), 3068–3077. doi:10.1002/term.2208
Li, X., Liang, Q., Zhang, W., Li, Y., Ye, J., Zhao, F., et al. (2017). Bio-inspired Bioactive Glasses for Efficient microRNA and Drug Delivery. J. Mat. Chem. B 5 (31), 6376–6384. doi:10.1039/c7tb01021d
Li, R., Wang, H., John, J. V., Song, H., Teusink, M. J., and Xie, J. (2020). 3D Hybrid Nanofiber Aerogels Combining with Nanoparticles Made of a Biocleavable and Targeting Polycation and MiR‐26a for Bone Repair. Adv. Funct. Mat. 30 (49), 2005531. doi:10.1002/adfm.202005531
Li, S., Liu, Y., Tian, T., Zhang, T., Lin, S., Zhou, M., et al. (2021). Bioswitchable Delivery of microRNA by Framework Nucleic Acids: Application to Bone Regeneration. Small 17 (47), 2104359. doi:10.1002/smll.202104359
Li, Z., Wang, Y., Li, S., and Li, Y. (2021). Exosomes Derived from M2 Macrophages Facilitate Osteogenesis and Reduce Adipogenesis of BMSCs. Front. Endocrinol. 12, 680328. doi:10.3389/fendo.2021.680328
Li, D., Yang, Z., Luo, Y., Zhao, X., Tian, M., and Kang, P. (2022). Delivery of MiR335‐5p‐Pendant Tetrahedron DNA Nanostructures Using an Injectable Heparin Lithium Hydrogel for Challenging Bone Defects in Steroid‐Associated Osteonecrosis. Adv. Healthc. Mater. 11 (1), 2101412. doi:10.1002/adhm.202101412
Liao, Y.-H., Chang, Y.-H., Sung, L.-Y., Li, K.-C., Yeh, C.-L., Yen, T.-C., et al. (2014). Osteogenic Differentiation of Adipose-Derived Stem Cells and Calvarial Defect Repair Using Baculovirus-Mediated Co-expression of BMP-2 and miR-148b. Biomaterials 35 (18), 4901–4910. doi:10.1016/j.biomaterials.2014.02.055
Lim, S. Y., Shen, W., and Gao, Z. (2015). Carbon Quantum Dots and Their Applications. Chem. Soc. Rev. 44 (1), 362–381. doi:10.1039/c4cs00269e
Liu, N., and Sun, Y. (2021). microRNA-148a-3p-targeting P300 Protects against Osteoblast Differentiation and Osteoporotic Bone Reconstruction. Regen. Med. 16 (5), 435–449. doi:10.2217/rme-2020-0006
Liu, C., Wen, J., Meng, Y., Zhang, K., Zhu, J., Ren, Y., et al. (2015). Efficient Delivery of Therapeutic miRNA Nanocapsules for Tumor Suppression. Adv. Mat. 27 (2), 292–297. doi:10.1002/adma.201403387
Liu, J., Meng, T., Ming, Y., Wen, L., Cheng, B., Liu, N., et al. (2016). MicroRNA-200c Delivered by Solid Lipid Nanoparticles Enhances the Effect of Paclitaxel on Breast Cancer Stem Cell. Int. J. Nanomedicine Vol. 11, 6713–6725. doi:10.2147/ijn.S111647
Liu, Z., Chang, H., Hou, Y., Wang, Y., Zhou, Z., Wang, M., et al. (2018a). Lentivirus-mediated microRNA-26a Overexpression in Bone Mesenchymal Stem Cells Facilitates Bone Regeneration in Bone Defects of Calvaria in Mice. Mol. Med. Rep. 18 (6), 5317–5326. doi:10.3892/mmr.2018.9596
Liu, Z., Chen, X., Zhang, Z., Zhang, X., Saunders, L., Zhou, Y., et al. (2018b). Nanofibrous Spongy Microspheres to Distinctly Release miRNA and Growth Factors to Enrich Regulatory T Cells and Rescue Periodontal Bone Loss. ACS Nano 12 (10), 9785–9799. doi:10.1021/acsnano.7b08976
Liu, H., Dong, Y., Feng, X., Li, L., Jiao, Y., Bai, S., et al. (2019). miR-34a Promotes Bone Regeneration in Irradiated Bone Defects by Enhancing Osteoblastic Differentiation of Mesenchymal Stromal Cells in Rats. Stem Cell Res. Ther. 10 (1), 180. doi:10.1186/s13287-019-1285-y
Liu, J., Dang, L., Wu, X., Li, D., Ren, Q., Lu, A., et al. (2019). microRNA-Mediated Regulation of Bone Remodeling: A Brief Review. JBMR Plus 3 (9), e10213. doi:10.1002/jbm4.10213
Liu, Q., Lin, Z., Liu, Y., Du, J., Lin, H., and Wang, J. (2019). Delivery of miRNA-29b Using R9-LK15, a Novel Cell-Penetrating Peptide, Promotes Osteogenic Differentiation of Bone Mesenchymal Stem Cells. BioMed Res. Int. 2019, 12. doi:10.1155/2019/3032158
Liu, J., Cui, Y., Kuang, Y., Xu, S., Lu, Q., Diao, J., et al. (2021). Hierarchically Porous Calcium-Silicon Nanosphere-Enabled Co-delivery of microRNA-210 and Simvastatin for Bone Regeneration. J. Mat. Chem. B 9 (16), 3573–3583. doi:10.1039/d1tb00063b
Lu, G. W., and Gao, P. (2010). “Emulsions and Microemulsions for Topical and Transdermal Drug Delivery,” in Handbook of Non-invasive Drug Delivery Systems. Editor V. S. Kulkarni (Boston: William Andrew Publishing), 59–94. doi:10.1016/b978-0-8155-2025-2.10003-4
Marew, T., and Birhanu, G. (2021). Three Dimensional Printed Nanostructure Biomaterials for Bone Tissue Engineering. Regen. Ther. 18, 102–111. doi:10.1016/j.reth.2021.05.001
Masotti, A., Miller, M. R., Celluzzi, A., Rose, L., Micciulla, F., Hadoke, P. W. F., et al. (2016). Regulation of Angiogenesis through the Efficient Delivery of microRNAs into Endothelial Cells Using Polyamine-Coated Carbon Nanotubes. Nanomedicine Nanotechnol. Biol. Med. 12 (6), 1511–1522. doi:10.1016/j.nano.2016.02.017
Mazziotta, C., Lanzillotti, C., Iaquinta, M. R., Taraballi, F., Torreggiani, E., Rotondo, J. C., et al. (2021). MicroRNAs Modulate Signaling Pathways in Osteogenic Differentiation of Mesenchymal Stem Cells. Int. J. Mol. Sci. 22 (5), 2362. doi:10.3390/ijms22052362
McCully, M., Conde, J., Baptista, P., Dalby, M. J., and Berry, C. C. (2018). Nanoparticle-antagomiR Based Targeting of miR-31 to Induce Osterix and Osteocalcin Expression in Mesenchymal Stem Cells. Plos One 13 (2), e0192562. doi:10.1371/journal.pone.0192562
Md, S., Alhakamy, N. A., Karim, S., Gabr, G. A., Iqubal, M. K., and Murshid, S. S. A. (2021). Signaling Pathway Inhibitors, miRNA, and Nanocarrier-Based Pharmacotherapeutics for the Treatment of Lung Cancer: A Review. Pharmaceutics 13 (12), 2120. doi:10.3390/pharmaceutics13122120
Meech, R., Edelman, D. B., Jones, F. S., and Makarenkova, H. P. (2005). The Homeobox Transcription Factor Barx2 Regulates Chondrogenesis during Limb Development. Development 132 (9), 2135–2146. doi:10.1242/dev.01811
Mencía Castaño, I., Curtin, C. M., Duffy, G. P., and O’Brien, F. J. (2016). Next Generation Bone Tissue Engineering: Non-viral miR-133a Inhibition Using Collagen-Nanohydroxyapatite Scaffolds Rapidly Enhances Osteogenesis. Sci. Rep. 6, 27941. doi:10.1038/srep27941
Mencía Castaño, I., Curtin, C. M., Duffy, G. P., and O'Brien, F. J. (2019). Harnessing an Inhibitory Role of miR-16 in Osteogenesis by Human Mesenchymal Stem Cells for Advanced Scaffold-Based Bone Tissue Engineering. Tissue Eng. Part A 25 (1-2), 24–33. doi:10.1089/ten.TEA.2017.0460
Mencía Castaño, I., Curtin, C. M., Shaw, G., Mary Murphy, J., Duffy, G. P., and O'Brien, F. J. (2015). A Novel Collagen-Nanohydroxyapatite microRNA-Activated Scaffold for Tissue Engineering Applications Capable of Efficient Delivery of Both miR-Mimics and antagomiRs to Human Mesenchymal Stem Cells. J. Control. Release 200, 42–51. doi:10.1016/j.jconrel.2014.12.034
Meng, Y.-B., Li, X., Li, Z.-Y., Zhao, J., Yuan, X.-B., Ren, Y., et al. (2015). microRNA-21 Promotes Osteogenic Differentiation of Mesenchymal Stem Cells by the PI3K/β-Catenin Pathway. J. Orthop. Res. 33 (7), 957–964. doi:10.1002/jor.22884
Meng, Y., Li, X., Li, Z., Liu, C., Zhao, J., Wang, J., et al. (2016a). Surface Functionalization of Titanium Alloy with miR-29b Nanocapsules to Enhance Bone Regeneration. ACS Appl. Mat. Interfaces 8 (9), 5783–5793. doi:10.1021/acsami.5b10650
Meng, Y., Liu, C., Zhao, J., Li, X., Li, Z., Wang, J., et al. (2016b). An Injectable miRNA-Activated Matrix for Effective Bone Regeneration In Vivo. J. Mat. Chem. B 4 (43), 6942–6954. doi:10.1039/c6tb01790h
Mercer, J., Lee, J. E., Saphire, E. O., and Freeman, S. A. (2020). SnapShot: Enveloped Virus Entry. Cell 182 (3), 786. e781. doi:10.1016/j.cell.2020.06.033
Mishra, B., and Singh, J. (2020). “Novel Drug Delivery Systems and Significance in Respiratory Diseases,” in Targeting Chronic Inflammatory Lung Diseases Using Advanced Drug Delivery Systems, 57–95. doi:10.1016/b978-0-12-820658-4.00004-2
Moncal, K. K., Aydin, R. S. T., Abu-Laban, M., Heo, D. N., Rizk, E., Tucker, S. M., et al. (2019). Collagen-infilled 3D Printed Scaffolds Loaded with miR-148b-Transfected Bone Marrow Stem Cells Improve Calvarial Bone Regeneration in Rats. Mater. Sci. Eng. C 105, 110128. doi:10.1016/j.msec.2019.110128
Nan, K., Zhang, Y., Zhang, X., Li, D., Zhao, Y., Jing, Z., et al. (2021). Exosomes from miRNA-378-Modified Adipose-Derived Stem Cells Prevent Glucocorticoid-Induced Osteonecrosis of the Femoral Head by Enhancing Angiogenesis and Osteogenesis via Targeting miR-378 Negatively Regulated Suppressor of Fused (Sufu). Stem Cell Res. Ther. 12 (1), 331. doi:10.1186/s13287-021-02390-x
Nayerossadat, N., Ali, P., and Maedeh, T. (2012). Viral and Nonviral Delivery Systems for Gene Delivery. Adv. Biomed. Res. 1, 27. doi:10.4103/2277-9175.98152
Nguyen, M. K., Jeon, O., Krebs, M. D., Schapira, D., and Alsberg, E. (2014). Sustained Localized Presentation of RNA Interfering Molecules from In Situ Forming Hydrogels to Guide Stem Cell Osteogenic Differentiation. Biomaterials 35 (24), 6278–6286. doi:10.1016/j.biomaterials.2014.04.048
Nguyen, M. K., Jeon, O., Dang, P. N., Huynh, C. T., Varghai, D., Riazi, H., et al. (2018). RNA Interfering Molecule Delivery from In Situ Forming Biodegradable Hydrogels for Enhancement of Bone Formation in Rat Calvarial Bone Defects. Acta Biomater. 75, 105–114. doi:10.1016/j.actbio.2018.06.007
Omote, H., and Moriyama, Y. (2013). Vesicular Neurotransmitter Transporters: an Approach for Studying Transporters with Purified Proteins. Physiology 28 (1), 39–50. doi:10.1152/physiol.00033.2012
Ou, L., Lan, Y., Feng, Z., Feng, L., Yang, J., Liu, Y., et al. (2019). Functionalization of SF/HAP Scaffold with GO-PEI-miRNA Inhibitor Complexes to Enhance Bone Regeneration through Activating Transcription Factor 4. Theranostics 9 (15), 4525–4541. doi:10.7150/thno.34676
Pan, T., Song, W., Gao, H., Li, T., Cao, X., Zhong, S., et al. (2016). miR-29b-Loaded Gold Nanoparticles Targeting to the Endoplasmic Reticulum for Synergistic Promotion of Osteogenic Differentiation. ACS Appl. Mat. Interfaces 8 (30), 19217–19227. doi:10.1021/acsami.6b02969
Peng, H., Lu, S. L., Bai, Y., Fang, X., Huang, H., and Zhuang, X. Q. (2018). MiR-133a Inhibits Fracture Healing via Targeting RUNX2/BMP2. Eur. Rev. Med. Pharmacol. Sci. 22 (9), 2519–2526. doi:10.26355/eurrev_201805_14914
Peng, Z., Lu, S., Lou, Z., Li, Z., Li, S., Yang, K., et al. (2021). Exosomes from Bone Marrow Mesenchymal Stem Cells Promoted Osteogenic Differentiation by Delivering miR-196a that Targeted Dickkopf-1 to Activate Wnt/β-Catenin Pathway. Bioengineered Epub ahead of print. doi:10.1080/21655979.2021.1996015
Qadir, A. S., Lee, J., Lee, Y. S., Woo, K. M., Ryoo, H. M., and Baek, J. H. (2018). Distal‐less Homeobox 3, a Negative Regulator of Myogenesis, Is Downregulated by microRNA‐133. J. Cell Biochem. 120, 2226–2235. doi:10.1002/jcb.27533
Qin, Y., Wang, L., Gao, Z., Chen, G., and Zhang, C. (2016). Bone Marrow Stromal/stem Cell-Derived Extracellular Vesicles Regulate Osteoblast Activity and Differentiation In Vitro and Promote Bone Regeneration In Vivo. Sci. Rep. 6, 21961. doi:10.1038/srep21961
Qu, H., Fu, H., Han, Z., and Sun, Y. (2019). Biomaterials for Bone Tissue Engineering Scaffolds: a Review. RSC Adv. 9 (45), 26252–26262. doi:10.1039/c9ra05214c
Qureshi, A. T., Monroe, W. T., Dasa, V., Gimble, J. M., and Hayes, D. J. (2013). miR-148b-nanoparticle Conjugates for Light Mediated Osteogenesis of Human Adipose Stromal/stem Cells. Biomaterials 34 (31), 7799–7810. doi:10.1016/j.biomaterials.2013.07.004
Rahim, M., Rizvi, S. M. D., Iram, S., Khan, S., Bagga, P. S., and Khan, M. S. (2018). “Interaction of Green Nanoparticles with Cells and Organs,” in Inorganic Frameworks as Smart Nanomedicines, 185–237. doi:10.1016/b978-0-12-813661-4.00005-5
Raj Preeth, D., Saravanan, S., Shairam, M., Selvakumar, N., Selestin Raja, I., Dhanasekaran, A., et al. (2021). Bioactive Zinc(II) Complex Incorporated PCL/gelatin Electrospun Nanofiber Enhanced Bone Tissue Regeneration. Eur. J. Pharm. Sci. 160, 105768. doi:10.1016/j.ejps.2021.105768
Reda El Sayed, S., Cristante, J., Guyon, L., Denis, J., Chabre, O., and Cherradi, N. (2021). MicroRNA Therapeutics in Cancer: Current Advances and Challenges. Cancers 13 (11), 2680. doi:10.3390/cancers13112680
Remy, M. T., Akkouch, A., He, L., Eliason, S., Sweat, M. E., Krongbaramee, T., et al. (2021). Rat Calvarial Bone Regeneration by 3D-Printed β-Tricalcium Phosphate Incorporating MicroRNA-200c. ACS Biomater. Sci. Eng. 7 (9), 4521–4534. doi:10.1021/acsbiomaterials.0c01756
Rossi, M., Pitari, M. R., Amodio, N., Di Martino, M. T., Conforti, F., Leone, E., et al. (2013). miR-29b Negatively Regulates Human Osteoclastic Cell Differentiation and Function: Implications for the Treatment of Multiple Myeloma-Related Bone Disease. J. Cell. Physiol. 228 (7), 1506–1515. doi:10.1002/jcp.24306
Ruedel, A., and Bosserhoff, A. K. (2012). “Transfection Methods,” in Laboratory Methods in Cell Biology, 163–182. doi:10.1016/b978-0-12-405914-6.00008-1
Samorezov, J. E., and Alsberg, E. (2015). Spatial Regulation of Controlled Bioactive Factor Delivery for Bone Tissue Engineering. Adv. Drug Deliv. Rev. 84, 45–67. doi:10.1016/j.addr.2014.11.018
Schade, A., Müller, P., Delyagina, E., Voronina, N., Skorska, A., Lux, C., et al. (2014). Magnetic Nanoparticle Based Nonviral MicroRNA Delivery into Freshly Isolated CD105(+) hMSCs. Stem Cells Int. 2014, 11. doi:10.1155/2014/197154
Scheideler, M., Vidakovic, I., and Prassl, R. (2020). Lipid Nanocarriers for microRNA Delivery. Chem. Phys. Lipids 226, 104837. doi:10.1016/j.chemphyslip.2019.104837
Shen, W., Sun, B., Zhou, C., Ming, W., Zhang, S., and Wu, X. (2020). CircFOXP1/FOXP1 Promotes Osteogenic Differentiation in Adipose‐derived Mesenchymal Stem Cells and Bone Regeneration in Osteoporosis via miR‐33a‐5p. J. Cell. Mol. Med. 24 (21), 12513–12524. doi:10.1111/jcmm.15792
Sriram, M., Sainitya, R., Kalyanaraman, V., Dhivya, S., and Selvamurugan, N. (2015). Biomaterials Mediated microRNA Delivery for Bone Tissue Engineering. Int. J. Biol. Macromol. 74, 404–412. doi:10.1016/j.ijbiomac.2014.12.034
Su, X., Liao, L., Shuai, Y., Jing, H., Liu, S., Zhou, H., et al. (2015). MiR-26a Functions Oppositely in Osteogenic Differentiation of BMSCs and ADSCs Depending on Distinct Activation and Roles of Wnt and BMP Signaling Pathway. Cell Death Dis. 6 (8), e1851. doi:10.1038/cddis.2015.221
Suh, J. S., Lee, J. Y., Choi, Y. S., Chong, P. C., and Park, Y. J. (2013). Peptide-mediated Intracellular Delivery of miRNA-29b for Osteogenic Stem Cell Differentiation. Biomaterials 34 (17), 4347–4359. doi:10.1016/j.biomaterials.2013.02.039
Sui, L., Wang, M., Han, Q., Yu, L., Zhang, L., Zheng, L., et al. (2018). A Novel Lipidoid-MicroRNA Formulation Promotes Calvarial Bone Regeneration. Biomaterials 177, 88–97. doi:10.1016/j.biomaterials.2018.05.038
Sun, M. H., Wang, W. J., Li, Q., Yuan, T., and Weng, W. J. (2018). Autologous Oxygen Release Nano Bionic Scaffold Composite miR-106a Induced BMSCs Enhances Osteoblast Conversion and Promotes Bone Repair through Regulating BMP-2. Eur. Rev. Med. Pharmacol. Sci. 22 (21), 7148–7155. doi:10.26355/eurrev_201811_16246
Sun, X., Li, X., Qi, H., Hou, X., Zhao, J., Yuan, X., et al. (2020). MiR-21 Nanocapsules Promote Early Bone Repair of Osteoporotic Fractures by Stimulating the Osteogenic Differentiation of Bone Marrow Mesenchymal Stem Cells. J. Orthop. Transl. 24, 76–87. doi:10.1016/j.jot.2020.04.007
Tahmasebi, A., Enderami, S. E., Saburi, E., Islami, M., Yaslianifard, S., Mahabadi, J. A., et al. (2020). Micro‐RNA‐incorporated Electrospun Nanofibers Improve Osteogenic Differentiation of Human‐induced Pluripotent Stem Cells. J. Biomed. Mater. Res. 108 (2), 377–386. doi:10.1002/jbm.a.36824
Tarach, P., and Janaszewska, A. (2021). Recent Advances in Preclinical Research Using PAMAM Dendrimers for Cancer Gene Therapy. Int. J. Mol. Sci. 22 (6), 2912. doi:10.3390/ijms22062912
Tian, T., Xiao, D., Zhang, T., Li, Y., Shi, S., Zhong, W., et al. (2020). A Framework Nucleic Acid Based Robotic Nanobee for Active Targeting Therapy. Adv. Funct. Mat. 31 (5), 2007342. doi:10.1002/adfm.202007342
Tu, M., Tang, J., He, H., Cheng, P., and Chen, C. (2017). MiR-142-5p Promotes Bone Repair by Maintaining Osteoblast Activity. J. Bone Min. Metab. 35 (3), 255–264. doi:10.1007/s00774-016-0757-8
Vimalraj, S., Saravanan, S., Vairamani, M., Gopalakrishnan, C., Sastry, T. P., and Selvamurugan, N. (2016). A Combinatorial Effect of Carboxymethyl Cellulose Based Scaffold and microRNA-15b on Osteoblast Differentiation. Int. J. Biol. Macromol. 93 (Pt B), 1457–1464. doi:10.1016/j.ijbiomac.2015.12.083
Vishal, M., Vimalraj, S., Ajeetha, R., Gokulnath, M., Keerthana, R., He, Z., et al. (2017). MicroRNA-590-5p Stabilizes Runx2 by Targeting Smad7 During Osteoblast Differentiation. J. Cell. Physiol. 232 (2), 371–380. doi:10.1002/jcp.25434
Wang, W., and Yeung, K. W. K. (2017). Bone Grafts and Biomaterials Substitutes for Bone Defect Repair: A Review. Bioact. Mater. 2 (4), 224–247. doi:10.1016/j.bioactmat.2017.05.007
Wang, X., Guo, B., Li, Q., Peng, J., Yang, Z., Wang, A., et al. (2013). miR-214 Targets ATF4 to Inhibit Bone Formation. Nat. Med. 19 (1), 93–100. doi:10.1038/nm.3026
Wang, Z., Wu, G., Wei, M., Liu, Q., Zhou, J., et al. (2016). Improving the Osteogenesis of Human Bone Marrow Mesenchymal Stem Cell Sheets by microRNA-21-Loaded Chitosan/hyaluronic Acid Nanoparticles via Reverse Transfection. Int. J. Nanomedicine 11, 2091–2105. doi:10.2147/ijn.S104851
Wang, Q., Shen, X., Chen, Y., Chen, J., and Li, Y. (2021). Osteoblasts-derived Exosomes Regulate Osteoclast Differentiation through miR-503-3p/Hpse axis. Acta Histochem. 123 (7), 151790. doi:10.1016/j.acthis.2021.151790
Wang, Y., He, R., Yang, A., Guo, R., Liu, J., Liang, G., et al. (2021). Role of miR-214 in Biomaterial Transplantation Therapy for Osteonecrosis. Bme, 1–14. doi:10.3233/bme-211296
Wang, Q.-y., Yali-Xiang, X., Hu, Q.-h., Huang, S.-h., Lin, J., and Zhou, Q.-h. (2022). Surface Charge Switchable Nano-Micelle for pH/redox-Triggered and Endosomal Escape Mediated Co-delivery of Doxorubicin and Paclitaxel in Treatment of Lung Adenocarcinoma. Colloids Surfaces B Biointerfaces 216, 112588. doi:10.1016/j.colsurfb.2022.112588
Wei, J., Li, H., Wang, S., Li, T., Fan, J., Liang, X., et al. (2014). let-7Enhances Osteogenesis and Bone Formation While Repressing Adipogenesis of Human Stromal/Mesenchymal Stem Cells by Regulating HMGA2. Stem Cells Dev. 23 (13), 1452–1463. doi:10.1089/scd.2013.0600
Wu, G., Feng, C., Hui, G., Wang, Z., Tan, J., Luo, L., et al. (2016). Improving the Osteogenesis of Rat Mesenchymal Stem Cells by Chitosan-Based-microRNA Nanoparticles. Carbohydr. Polym. 138, 49–58. doi:10.1016/j.carbpol.2015.11.044
Wu, S., Liu, W., and Zhou, L. (2016). MiR-590-3p Regulates Osteogenic Differentiation of Human Mesenchymal Stem Cells by Regulating APC Gene. Biochem. Biophysical Res. Commun. 478 (4), 1582–1587. doi:10.1016/j.bbrc.2016.08.160
Wu, G., Feng, C., Quan, J., Wang, Z., Wei, W., Zang, S., et al. (2018). In Situ controlled Release of Stromal Cell-Derived Factor-1α and antimiR-138 for On-Demand Cranial Bone Regeneration. Carbohydr. Polym. 182, 215–224. doi:10.1016/j.carbpol.2017.10.090
Xie, Q., Wang, Z., Zhou, H., Yu, Z., Huang, Y., Sun, H., et al. (2016). The Role of miR-135-Modified Adipose-Derived Mesenchymal Stem Cells in Bone Regeneration. Biomaterials 75, 279–294. doi:10.1016/j.biomaterials.2015.10.042
Xie, Q., Wei, W., Ruan, J., Ding, Y., Zhuang, A., Bi, X., et al. (2017). Effects of miR-146a on the Osteogenesis of Adipose-Derived Mesenchymal Stem Cells and Bone Regeneration. Sci. Rep. 7, 42840. doi:10.1038/srep42840
Xiong, A., He, Y., Gao, L., Li, G., Weng, J., Kang, B., et al. (2020). Smurf1-targeting miR-19b-3p-Modified BMSCs Combined PLLA Composite Scaffold to Enhance Osteogenic Activity and Treat Critical-Sized Bone Defects. Biomater. Sci. 8 (21), 6069–6081. doi:10.1039/d0bm01251c
Xu, G., Ding, Z., and Shi, H.-f. (2019). The Mechanism of miR-889 Regulates Osteogenesis in Human Bone Marrow Mesenchymal Stem Cells. J. Orthop. Surg. Res. 14 (1), 366. doi:10.1186/s13018-019-1399-z
Xue, Y., Guo, Y., Yu, M., Wang, M., Ma, P. X., and Lei, B. (2017). Monodispersed Bioactive Glass Nanoclusters with Ultralarge Pores and Intrinsic Exceptionally High miRNA Loading for Efficiently Enhancing Bone Regeneration. Adv. Healthc. Mat. 6 (20), 1700630. doi:10.1002/adhm.201700630
Yan, S. G., Zhang, J., Tu, Q. S., Ye, J. H., Luo, E., Schuler, M., et al. (2011). Enhanced Osseointegration of Titanium Implant through the Local Delivery of Transcription Factor SATB2. Biomaterials 32 (33), 8676–8683. doi:10.1016/j.biomaterials.2011.07.072
Yan, J., Zhang, C., Zhao, Y., Cao, C., Wu, K., Zhao, L., et al. (2014). Non-viral Oligonucleotide antimiR-138 Delivery to Mesenchymal Stem Cell Sheets and the Effect on Osteogenesis. Biomaterials 35 (27), 7734–7749. doi:10.1016/j.biomaterials.2014.05.089
Yan, J., Lu, X., Zhu, X., Hu, X., Wang, L., Qian, J., et al. (2020). Effects of miR-26a on Osteogenic Differentiation of Bone Marrow Mesenchymal Stem Cells by a Mesoporous Silica Nanoparticle - PEI - Peptide System. Int. J. Nanomedicine 15, 497–511. doi:10.2147/ijn.S228797
Yang, C., Liu, X., Zhao, K., Zhu, Y., Hu, B., Zhou, Y., et al. (2019). miRNA-21 Promotes Osteogenesis via the PTEN/PI3K/Akt/HIF-1α Pathway and Enhances Bone Regeneration in Critical Size Defects. Stem Cell Res. Ther. 10 (1), 65. doi:10.1186/s13287-019-1168-2
Yang, K., Zhang, S., He, J., and Nie, Z. (2021). Polymers and Inorganic Nanoparticles: A Winning Combination towards Assembled Nanostructures for Cancer Imaging and Therapy. Nano Today 36, 101046. doi:10.1016/j.nantod.2020.101046
Yang, L., He, X., Jing, G., Wang, H., Niu, J., Qian, Y., et al. (2021). Layered Double Hydroxide Nanoparticles with Osteogenic Effects as miRNA Carriers to Synergistically Promote Osteogenesis of MSCs. ACS Appl. Mat. Interfaces 13 (41), 48386–48402. doi:10.1021/acsami.1c14382
Yoshizuka, M., Nakasa, T., Kawanishi, Y., Hachisuka, S., Furuta, T., Miyaki, S., et al. (2016). Inhibition of microRNA-222 Expression Accelerates Bone Healing with Enhancement of Osteogenesis, Chondrogenesis, and Angiogenesis in a Rat Refractory Fracture Model. J. Orthop. Sci. 21 (6), 852–858. doi:10.1016/j.jos.2016.07.021
Yu, M., Xue, Y., Ma, P. X., Mao, C., and Lei, B. (2017). Intrinsic Ultrahigh Drug/miRNA Loading Capacity of Biodegradable Bioactive Glass Nanoparticles toward Highly Efficient Pharmaceutical Delivery. ACS Appl. Mat. Interfaces 9 (10), 8460–8470. doi:10.1021/acsami.6b13874
Yuan, X., Han, L., Lin, H., Guo, Z., Huang, Y., Li, S., et al. (2019). The Role of antimiR-26a-5p/biphasic Calcium Phosphate in Repairing Rat Femoral Defects. Int. J. Mol. Med. 44 (3), 857–870. doi:10.3892/ijmm.2019.4249
Zare, H., Ahmadi, S., Ghasemi, A., Ghanbari, M., Rabiee, N., Bagherzadeh, M., et al. (2021). Carbon Nanotubes: Smart Drug/Gene Delivery Carriers. Int. J. Nanomedicine 16, 1681–1706. doi:10.2147/ijn.S299448
Zhang, X., Li, Y., Chen, Y. E., Chen, J., and Ma, P. X. (2016). Cell-free 3D Scaffold with Two-Stage Delivery of miRNA-26a to Regenerate Critical-Sized Bone Defects. Nat. Commun. 7, 10376. doi:10.1038/ncomms10376
Zhang, Q., Lin, S., Shi, S., Zhang, T., Ma, Q., Tian, T., et al. (2018). Anti-inflammatory and Antioxidative Effects of Tetrahedral DNA Nanostructures via the Modulation of Macrophage Responses. ACS Appl. Mat. Interfaces 10 (4), 3421–3430. doi:10.1021/acsami.7b17928
Zhang, L., Yang, G., Johnson, B. N., and Jia, X. (2019). Three-dimensional (3D) Printed Scaffold and Material Selection for Bone Repair. Acta Biomater. 84, 16–33. doi:10.1016/j.actbio.2018.11.039
Zhang, J., Zhang, T., Tang, B., Li, J., and Zha, Z. (2021). The miR-187 Induced Bone Reconstruction and Healing in a Mouse Model of Osteoporosis, and Accelerated Osteoblastic Differentiation of Human Multipotent Stromal Cells by Targeting BARX2. Pathology - Res. Pract. 219, 153340. doi:10.1016/j.prp.2021.153340
Zhang, Y., Zhuang, Z., Wei, Q., Li, P., Li, J., Fan, Y., et al. (2021). Inhibition of miR‐93‐5p Promotes Osteogenic Differentiation in a Rabbit Model of Trauma‐induced Osteonecrosis of the Femoral Head. FEBS Open Bio 11, 2152–2165. doi:10.1002/2211-5463.13218
Zhang, Z., Jiang, W., Hu, M., Gao, R., and Zhou, X. (2021). MiR-486-3p Promotes Osteogenic Differentiation of BMSC by Targeting CTNNBIP1 and Activating the Wnt/β-Catenin Pathway. Biochem. Biophysical Res. Commun. 566, 59–66. doi:10.1016/j.bbrc.2021.05.098
Zhao, C., Sun, W., Zhang, P., Ling, S., Li, Y., Zhao, D., et al. (2015). miR-214 Promotes Osteoclastogenesis by Targeting Pten/PI3k/Akt Pathway. RNA Biol. 12 (3), 343–353. doi:10.1080/15476286.2015.1017205
Zhao, C., Gu, Y., Wang, Y., Qin, Q., Wang, T., Huang, M., et al. (2021). miR-129-5p Promotes Osteogenic Differentiation of BMSCs and Bone Regeneration via Repressing Dkk3. Stem Cells Int. 2021, 18. doi:10.1155/2021/7435605
Keywords: bone tissue engineering, microRNA, calcium phosphates, nanoparticles, transfection, nonviral vectors, osteogenesis
Citation: Zhu M, Gu Y, Bian C, Xie X, Bai Y and Zhang N (2022) Applications of Nonviral Biomaterials for microRNA Transfection in Bone Tissue Engineering. Front. Mater. 9:932157. doi: 10.3389/fmats.2022.932157
Received: 29 April 2022; Accepted: 09 June 2022;
Published: 22 July 2022.
Edited by:
Anuj Kumar, Yeungnam University, South KoreaReviewed by:
Xiaoyu Du, ETH Zürich, SwitzerlandSangram Keshari Samal, Regional Medical Research Center (ICMR), India
Ganesh Ingavle, Symbiosis International University, India
Copyright © 2022 Zhu, Gu, Bian, Xie, Bai and Zhang. This is an open-access article distributed under the terms of the Creative Commons Attribution License (CC BY). The use, distribution or reproduction in other forums is permitted, provided the original author(s) and the copyright owner(s) are credited and that the original publication in this journal is cited, in accordance with accepted academic practice. No use, distribution or reproduction is permitted which does not comply with these terms.
*Correspondence: Ning Zhang, ZGVudGlzdHpoYW5nMTEyQDE2My5jb20=
†These authors have contributed equally to this work