- 1Institute of Neuroregeneration and Neurorehabilitation, Qingdao Medical College, Qingdao University, Qingdao, China
- 2Shandong Key Laboratory of Textile Materials for Healthcare, Collaborative Innovation Center for Eco-textiles of Shandong Province and the Ministry of Education, Qingdao, China
- 3Qingdao Stomatological Hospital, Qingdao, China
Three-dimensional (3D) printing technology has emerged as a revolutionary manufacturing strategy that could realize rapid prototyping and customization. It has revolutionized the manufacturing process in the fields of electronics, energy, bioengineering and sensing. Based on digital model files, powdered metal, plastic and other materials were used to construct the required objects by printing layer by layer. In addition, 3D printing possesses remarkable advantages in realizing controllable compositions and complex structures, which could further produce 3D objects with anisotropic functions. In recent years, 3D bioprinting technology has been applied to manufacture functional tissue engineering scaffolds with its ability to assemble complicated construction under precise control, which has attracted great attention. Bioprinting creates 3D scaffolds by depositing and assembling biological and/or non-biological materials with an established tissue. Compared with traditional technology, it can create a structure tailored to the patient according to the medical images. This conception of 3D bioprinting draws on 3D printing technology, which could be utilized to produce personalized implants, thereby opening up a new way for bio-manufacturing methods. As a promising tool, 3D bioprinting can create complex and delicate biomimetic 3D structures, simulating extracellular matrix and preparing high precision multifunctional scaffolds with uniform cell distribution for tissue repair and regeneration. It can also be flexibly combined with other technologies such as electrospinning and thermally induced phase separation, suitable for tissue repair and regeneration. This article reviews the relevant research and progress of 3D bioprinting in tissue repair and regeneration in recent years. Firstly, we will introduce the physical, chemical and biological characteristics of biological scaffolds prepared by 3D bioprinting from several aspects. Secondly, the significant effects of 3D bioprinting on nerves, skin, blood vessels, bones and cartilage injury and regeneration are further expounded. Finally, some views on the clinical challenges and future opportunities of 3D bioprinting are put forward.
1 Introduction
The traditional tissue engineering method is to inoculate cells on the prepared material scaffold after proliferating in vitro to form a complex of “scaffold + cell”. After culturing in vitro for a certain period, the complex is implanted into the patient’s body (Groll et al., 2016). With the gradual degradation and absorption of biomaterials in vivo, the implanted cells continue to proliferate, differentiate and secrete extracellular matrix, and finally achieve the purpose of tissue or organ repair and functional reconstruction (Zhao and Cui, 2020; Abdollahiyan et al., 2021). In the past decades, tissue engineering has gained enormous progress, but traditional methods such as solution casting/particle leaching method, thermally induced phase separation method, foaming and electrospinning, cannot effectively control the pore size, porosity and structure of a sequential network structure, and the repeatability is poor (Zhang et al., 2020; Gao C. et al., 2021; Agarwal et al., 2021; Qu et al., 2021; Zou et al., 2022). However, 3D printing technology can precisely control the appearance and internal structure of printed objects through computer-aided design modeling (Palmara et al., 2021). Its emergence has made the design and construction of porous scaffolds develop by leaps and bounds. 3D printing can efficaciously integrate diverse types of living cells into 3D structures composed of traditional micro-nano biomaterials, thereby creating artificial grafts that can regenerate damaged tissues (Cheng et al., 2021). 3D printing and relevant biological manufacturing methods supply a perfect set of tools for the operation of materials, cells and molecules to construct on-demand customized structures in a reproducible way (Prendergast and Burdick, 2020). Based on the preparation of adjustable 3D tissue structure, it can encapsulate cells and growth factors, which provides a new strategy for designing biomimetic scaffolds for tissue repair and regeneration (Groll et al., 2016).
In addition to engineered tissue scaffolds, this technology is also used for drug delivery (Chia and Wu, 2015; Sandler and Preis, 2016; van der Elst et al., 2021). The current drug delivery system still cannot meet the needs for individualized treatment of patients. However, the advancement in technology has made it possible to realize personalized drug dosage regimens with 3D printing technology, which has the characteristics of flexibility and precise control (Sandler and Preis, 2016). Furthermore, 3D bioprinting has demonstrated its potential to construct transplantable hard organs/tissues in tissue engineering (Ng et al., 2019; Fonseca et al., 2020). However, there are still some uncertainties in the 3D bioprinting methods in the manufacture of 3D hard organs/tissues (Abdollahiyan et al., 2021). Although 3D printing achieves impressive configurable detail, the process depends on whether there is a suitable imaging method, and in some cases, the implantation of printing structures can be challenging (Tamimi et al., 2009; Klammert et al., 2010). The prefabricated structures that obtain the same size and shape of injuries can be applied to treat predictable injuries, under some circumstances, the geometry of the injury lies on the surgical technique and may be changed during the surgical debridement (Ashammakhi et al., 2019). In addition, how to ensure cell viability after printing is one of the core concerns of 3D bioprinting. In traditional extrusion bioprinting, the cell-loaded bioink is extruded through a nozzle, and the shear stress during the extrusion process will inevitably damage cells (Blaeser et al., 2016; Jentsch et al., 2021). The performance and application of the scaffold can be adjusted according to the type of material applied in 3D printing. The microporous structure of traditional biomaterials (ceramics, polymers, metals and their composites) limited their application in 3D bioprinting. Compared with the above traditional biomaterials, nanomaterials have a higher surface area and volume ratio, and their size range is closer to mimicking the structure of natural tissues, which can improve the rate of cell adhesion and proliferation. Therefore, the combination of 3D bioprinting and nanomaterials creates conditions for the preparation of ideal multifunctional scaffolds (Bhatt and Anbarasu, 2017; Rana et al., 2017). One of the most critical obstacles in the clinical application of 3D bioprinting is the selection of an appropriate cell source, which should be safe, minimally invasive, fast and easy to expand (Amler et al., 2021). In addition, 3D scaffold surface functionalization usually requires post-processing treatment, which may lead to inevitable side effects or change the original morphology and shape of the scaffold (Camacho et al., 2021).
In the past decade, 3D printing has become an extremely popular multi-functional technology platform for the rapid manufacture of synthetic substitutes for regenerative medicine (Memarian et al., 2021). In the biomedical field, 3D printing technology is committed to 1) creating personalized prosthetics, anatomical models and implants; 2) manufacturing medical devices; 3) reconstruction of organs and tissues (Kim et al., 2008). At present, the most common 3D printed artificial scaffolds for tissues or organs in the medical field include skin, nerves, blood vessels, cartilage and bones (Lin et al., 2018; Setacci et al., 2018; Yan et al., 2018). In addition, biological scaffolds prepared by 3D printing or their combination with other technologies play an active role in the repair and regeneration of the above organs and tissues.
2 Different Categories of 3D Printing Technology to Construct Scaffolds
Compared with the traditional manufacturing technology, 3D printing has the following three main advantages: unlimited design space, which means that objects with any complex geometric structure can be processed, and designers can design arbitrarily complex geometric shapes; zero-skill manufacturing, which means not requiring higher skills to operate, and can easily realize the personalized design and customization of products; the infinite combination of materials, which refers to the ability to print multiple materials in combination to produce “new products” with different properties. In other words, the three most valued advantages of 3D printing technology are accelerating the product development process, providing personalized and customized products and increasing production flexibility. 3D printing is not a single technology, it integrates cutting-edge technologies in many fields such as digital modeling technology, electromechanical control technology, information technology, materials science, chemistry and biomedicine. At present, the most common 3D printing technologies are mainly divided into three categories: extrusion printing, inkjet printing and laser-assisted bioprinting (LAB) (Figure 1) (Azad et al., 2020). Although no single bioprinting technology can completely replicate the complexity of various tissues, each technology has its advantages and can make up for each other’s shortcomings and limitations (Groll et al., 2016; Mandrycky et al., 2016). The applied bioprinting method depends on the necessary considerations of biological issues and their complexity, resolution and cell structure.
2.1 Extrusion Printing
Extrusion-based 3D printing is the most common method, which has attracted more attention by its usability (Baniasadi et al., 2021; Chen et al., 2021). The concept of extrusion bioprinting is to squeeze bioink from the printer nozzle for printing custom-designed tissue engineering scaffolds and physiologically related organs (Daly et al., 2021). The extrusion bioprinting device consists of two parts: a fluid supply system and an automatic extrusion printing system. The computer-controlled deposition system prints the bioink into shape, and the cells are accurately encapsulated in a 3D structure. Products manufactured by this technology have good structural integrity. The diameter of the extrusion bioprinting nozzle can reach a larger size, the nozzle design is more flexible, and the “ink” can be nearly solid, which makes the printing “ink” diversified.
In addition, this printing method can perform selective material melting (Baniasadi et al., 2021). It allows printing bioinks with high viscosity and high cell density, which is beneficial for controlling the pore structure of the scaffold and is suitable for making structures with appropriate dimensions (Murphy and Atala, 2014). Extrusion bioprinting can be divided into three types: pneumatic drive, mechanical drive (piston or screw) and solenoid drive, among which pneumatic drive and piston drive are more commonly used (Ning and Chen, 2017). This technology can be easily transformed into multi-nozzle technology, which can easily print various materials and cells, and further fabricate different types of biological scaffolds for tissue repair and regeneration. However, due to the mechanical requirements for structural stability after extrusion, the ink is usually quite viscous, about 10–300 Pa s, which will lead to the problems of high shear force and low cell survival rate (Blaeser et al., 2016; Kang et al., 2018). In addition, due to the mechanical limitation of extruding viscous materials through small nozzles, the diameter of the nozzles used is limited, which further limits the resolution of extrusion printing (Bedell et al., 2020). The ideal extrusion bioink should be shear thinner and able to achieve low viscosity in the high shear environment of the printing nozzle, which is awaiting further exploration and development by researchers.
2.2 Inkjet Printing
Inkjet bioprinting originates from traditional 2D inkjet printers and belongs to the category of extrusion bioprinting, which involves the deposition of bioink droplets through nozzles instead of continuous filaments (Gudapati et al., 2016; Li et al., 2020). In theory, inkjet 3D printing is capable of using almost any powder material, such as biopolymer, ceramic and metal powder. Especially in recent years, it has attracted extensive attention in the research fields of polymer molding, nanocomposites and tissue engineering (Farahani et al., 2016; Mandrycky et al., 2016; Ligon et al., 2017). UV curable technology is currently the most environmentally friendly and rapidly growing technology, and its combination with 3D inkjet printing provides high resolution, fast curing, and the ability to change the geometry and material composition in a flexible, adjustable manner. Moreover, the increase in the number of nozzles and/or print heads allows for flexible and controllable commercial production speeds (Clark et al., 2017; Hong et al., 2019). Inkjet printing is one of the pioneers of 3D printing, which can realize cell printing to manufacture tissues or organs. On this basis, inkjet bioprinting was born as a branch of bioprinting (Moroni et al., 2018). Drop-on-demand inkjet printing and continuous inkjet printing are two major types of inkjet technology, among which drop-on-demand inkjet printing has become the main strategy of inkjet bioprinting due to its unique advantages. Drop-on-demand inkjet nozzles only generate droplets while the ejection signal is reached, which makes it possible to obtain higher accuracy and higher ink utilization (Li et al., 2020).
Inkjet droplets are generally 10–150 pL, and their volume change along with pulse frequency, temperature gradient and material viscosity. Therefore, inkjet bioprinting can achieve a resolution of fewer than 30 μm (Angelopoulos et al., 2020). And this printing technology is affected by low cell density, and the viscosity range of the related printing materials applied is extremely limited (Ostrovidov et al., 2019). However, inkjet printing has the preponderances of low technical cost, high printing resolution and the ability to print multiple materials synchronously (Saunders and Derby, 2014).
2.3 Laser-Assisted Bioprinting
The LAB with reproducible characteristics could fabricate tissues with similar physiological functions as autologous tissues. Stereolithography and selective laser sintering are both variants of laser-assisted 3D printing technology. Since the nozzle construction of laser-assisted printing belongs to the open type, there is no common nozzle blocking problem with other 3D bioprinting (Luo et al., 2018). The mechanism of this printing method is similar to inkjet printing, but the ink will not pass through the nozzle like inkjet printing, so no wall shear stress acts on the cells. Based on the above situation, LAB usually produces a higher percentage of cell viability (Xiong et al., 2017). Furthermore, the laser and the picoliter-sized droplets ejected from the bioink enable the LAB to possess exceedingly high printing resolution and own ability to print single cells (Keriquel et al., 2017).
The LAB platforms are also increasingly used for cell printing and tissue engineering applications. Relevant studies have shown that this technology can accurately control the density and position of cells on the scaffold, providing a new strategy for the repair and regeneration of various tissues. As a non-contact molding method, this technology can maximize the biological activity of the bioink. However, LAB has ineluctable problems such as low printing efficiency, high price and difficulty in printing large-size tissues and organs.
3 Different Types of Bioinks for 3D Bioprinting
Living tissue is a kind of complex heterostructure, which is composed of building blocks of different sizes arranged in layers to obtain multifunctional characteristics (Levato et al., 2014; Jeon et al., 2019). Since 3D printing can prepare excellent 3D scaffolds to simulate natural tissues, it has been increasingly applied in tissue engineering (Chen et al., 2020). 3D bioprinting ultimately produces artificial organs and biomedical products by assembling a special bioink. The bioink is mainly responsible for the function of constructing 3D structures and reconstructing tissue. Therefore, the selection of bioink is very important, in which the printability, biocompatibility and mechanical properties must be balanced (Saroia et al., 2018; Gu et al., 2020). Materials, cells and bioactive molecules have always been considered as important components of bioinks, which are used to print structures similar to natural tissues (Figure 2) (Yang et al., 2020).
It must be emphasized that the applicability of bioinks will directly determine whether 3D bioprinting technology can be used in clinics. However, bioinks have bottlenecks in solving particular biocompatibility challenges, printability and functional characters (Chen et al., 2021). During 3D bioprinting, high temperatures or organic solvents are incompatible with the deposition of living cells and bioactive molecules. Furthermore, structural integrity and fidelity are difficult to maintain while maintaining high cell viability and phenotype. As a result, the potential to create anatomically accurate multilayered tissues with the ability to naturally replicate human pathophysiological functions is limited (Gold et al., 2021). In the current research, the commonly used materials for constructing bioinks are mainly divided into two categories: synthetic polymers, such as polycaprolactone (PCL), polylactic acid (PLA), polylactic acid-glycolic acid (PLGA), polyethylene oxide terephthalate-co-butylene terephthalate (PEO/PBT), and nature polymers such as alginic acid, hyaluronic acid, decellularized extracellular matrix, gelatin, agarose, and nanocellulose (Agarwal et al., 2021). According to the different types of repaired tissues, related cells and/or biomolecules are added to make the designed and prepared 3D bioprinted scaffolds more versatile (Apelgren et al., 2021). 3D cell printing is the uniform mixing of cells and materials to be printed, hence the printing material must own printability and crosslinkability, as well as good biocompatibility and necessary mechanical properties. With the materials listed above, single and/or combined utilization could achieve these relevant properties and meet the requirements for preparing 3D biological scaffolds. The difference in materials and applied bioprinting technology leads to the difference in mechanical properties of 3D scaffold, which determines the application position of the scaffolds, such as bone, cartilage, skin, nerves and blood vessels. Next, biological materials, cells and bioactive molecules commonly used in current research are summarized.
3.1 Materials
3.1.1 Synthetic Polymers
PCL is a common polymer with high mechanical strength, good biocompatibility, degradability and printability. It has been approved by FDA for medical devices. Because PCL has the characteristics of shape memory, it makes the printed things have “memory”, which can be restored to the original shape under certain conditions. In the medical field, it can be used to print the heart, bones, nerve scaffolds, etc. Because of these excellent properties, PCL has become the most widely used material among multitudinous materials. However, PCL has shortcomings such as hydrophobicity or lack of cell adhesion. To cope with these problems, various methods or technologies have been proposed to modify the printed biological scaffolds to meet the needs of tissue repair and regeneration (Mousavi Nejad et al., 2021).
PLA is a condensation product of α-hydroxy propionic acid, which belongs to a thermoplastic aliphatic polyester. It possesses the advantages of excellent mechanical properties, processability, transparency, dimensional stability and biocompatibility. It is biodegradable thermoplastic, which comes from renewable resources and is recognized as an environmentally friendly material (Sikorska et al., 2018). Secondly, it is the biodegradable material with the largest production capacity, and its dosage ratio is increasing year by year among biodegradable plastics, making it a potential environmentally friendly solution in the field of 3D bioprinting. Because it is degraded in the human body and eventually excreted from the body in the form of CO2 and H2O, thus widely used in medical applications (Musioł et al., 2016; da Silva et al., 2018). Besides, PLA film has excellent antibacterial and antifungal properties, and has a broad market prospect in the preparation of biomedical materials by 3D printers. As a perfect example of “biomaterials”, PLA has innovative multi-dimensional applications. However, pure PLA has some shortcomings of poor toughness, high brittleness and heat resistance (Bardot and Schulz, 2020). At present, there has been a lot of research on the modification of PLA, and the 3D bioprinting consumables of PLA base materials have been updated for a long time (Brézulier et al., 2021).
As a degradable polymer material, PLGA has been certified by FDA as a pharmaceutical excipient and included in the FDA Pharmacopoeia (Danhier et al., 2012). Lactic acid and glycolic acid, the degradation products of PLGA, are also byproducts of the human metabolic pathway, so they have no toxic side effects when used in medicine and biomaterials. At the same time, it has good biocompatibility, will not cause inflammation of surrounding tissues and has no rejection, so it has been widely used in tissue engineering (Du et al., 2020). There are plenty of reports on the preparation and application of various PLGA drug microspheres, among which PLGA microspheres as protein and enzyme drug carriers are the research hotspot (Chereddy et al., 2018). In addition, the reliability and thermal properties of PLGA enable it to be processed by additive manufacturing technology of melt extrusion (Gradwohl et al., 2021).
The uniform properties of synthetic polymers are that they can be manufactured under controlled conditions, so they exhibit generally predictable and reproducible mechanical and physical properties. The more important advantage of synthetic polymers is the ability to control impurities in the material. In biological applications, hydrogels based on synthetic polymers such as PCL, PLA and PLGA can adjust the final mechanical properties by changing the functional groups, molecular weight or polymerization chemistry, thereby customizing performance for specific applications (Yu et al., 2020). However, it is worth noting that the bioprinting of hydrated and ductile 3D hydrogel scaffolds with ideal structural properties has not yet been fully realized (Ning et al., 2021).
3.1.2 Natural Polymers
Collagen is widely found in all tissues from the body surface of lower vertebrates to the body of mammals, namely cartilage, skin, bones and other tissues (Gao J. et al., 2021). As a natural polymer, collagen has been proved in clinical to significantly promote the repair, regeneration and reconstruction of defective tissues. Collagen bioink is one of the most widely used in the field of tissue engineering. Type I collagen widely exists in the human body, accounting for more than 90% of the total mass of collagen (Valot et al., 2019). Collagen, as a natural biological material, has also been applied in the field of 3D bioprinting. However, collagen is temperature-sensitive and prone to degradation during most sterilization processes, so collagen scaffolds often need to be cross-linked or used in combination with other materials. The 3D collagen scaffold with high porosity, permeability and biocompatibility, could facilitate the formation of new tissues (Cheng et al., 2021). Although collagen has good biocompatibility, it is difficult for collagen to form a cell-carrying bioink with appropriate viscosity. The formed scaffold has low strength and is very sensitive to metalloproteinases, and further exploration of composite bioinks with collagen as the core is needed. Moreover, the pH must be adjusted before using collagen as a hydrogel material, because the fibers formed under neutral pH conditions form a physical gel within 30 min at room temperature, and the concentration is as low as 1.5 mg/ml (Mazzocchi et al., 2018).
Gelatin with good biocompatibility, high water absorption and no immunogenicity, belongs to denatured collagen, which is the product of partial hydrolysis of collagen, the main component of the natural extracellular matrix (Liu et al., 2021). Because gelatin has strong maneuverability, like some synthetic polymers, it will be given priority over collagen in some experimental studies (Wu and Ding, 2004). Like collagen, gelatin must be treated with acid or alkali before use. The functionalized form of gelatin methacrylate is compatible with many different types of crosslinking chemistry, photoinitiators and other polymerization systems for long-term cell encapsulation (Yue et al., 2015). Although collagen and gelatin can promote the deposition of extracellular matrix in vitro, the human body may still have an immune response to gelatin or collagen from external sources (Lynn et al., 2004). The shape fixation rate of the gel system is significantly improved after glutaraldehyde cross-linking, and the pores of the scaffold formed by gelatin remain intact, but this approach reduces the biocompatibility of the scaffold. Based on this characteristic of gelatin, pure gelatin is usually used as a sacrificial material in 3D bioprinting, which is conducive to the transmission of oxygen and nutrients. In order to retain the biocompatibility of gelatin and enhance its mechanical strength, the modification of gelatin and the use of UV cross-linking have attracted the attention of a large number of researchers (Billiet et al., 2014).
As a biodegradable, negatively charged and nonimmunogenic polysaccharide, alginate has been widely used in tissue engineering. Its non-toxic and shear thinning are the prerequisites for the application of bioink materials (Bouhadir et al., 2001; Rastogi and Kandasubramanian, 2019). This kind of bioink has been broadly used in the preparation of 3D cell loading structures due to its advantages of low toxicity, good biocompatibility and fast cross-linking. However, the main disadvantage of alginic acid hydrogel is low bioactivity. Therefore, modification of bioactive ingredients is a promising strategy to effectively improve its bioactivity. The existing single-component hydrogels cannot fully satisfy the needs of physical or biological properties. For instance, the biological activity of bioinks may be high, but their printability may be poor and vice versa (Lee et al., 2020). The most commonly used gel-forming method for alginate is to employ calcium chloride (CaCl2) solution as a chelating agent to initiate cross-linking and curing of alginate after 3D printing. At the same time, this method can also better maintain the biological phase of the printed scaffold, so it is widely used in 3D bioprinting. The limitation of alginate is the short cell-binding domain on the polymer chain, and calcium ions may cause inflammation in the body (Chan and Mooney, 2013). Therefore, modifying alginate to improve the adhesion, stretching and proliferation of cells on the alginate scaffold has become the current trend of using alginate for 3D bioprinting.
In biological applications, the mechanical properties of hydrogels based on natural polymers such as collagen, gelatin and hyaluronic acid largely depend on the concentration, cross-linking mechanism and the cross-linking conditions of the materials. In addition to the above factors, combining multiple kinds of natural materials into composite materials can further facilitate mechanical properties. So far, through 3D cell printing, a variety of single-component bioinks, such as alginic acid, hyaluronic acid, silk fibroin and collagen have been taken into account for the development of multifarious tissue structure scaffolds (Chae et al., 2021). However, a large number of experimental studies showed that a single bioink is more restrictive, and the prepared scaffold can not achieve ideal performance. Therefore, to meet more performance requirements, the construction of composite bioinks is one of the current development trends of 3D bioprinting.
3.2 Cells
The selection of cell types is crucial for 3D bioprinting related biological tissues or organs. Different tissues of the human body are composed of unique cells, and different kinds of cells live in different physical and chemical environments. However, not all tissue-specific cells can be isolated and cultured in vitro, and not all cells can maintain their biological activity after undergoing a 3D bioprinted environment. Therefore, how to optimize cell separation, culture and enhancement of proliferation technology have always been the focus of exploration and improvement of 3D bioprinting. At present, although the 3D structures of cell-loaded hydrogels or other biomaterials have been widely established in vitro (Table 1), their effectiveness in vivo still needs to be verified by a large number of long-term clinical trials (Cidonio et al., 2019).
Stem cells have extremely broad application prospects in the fields of cell repair, developmental biology and pharmacology of life sciences. They have been proved to be the key factor in human tissue repair and regeneration for a long time. For example, based on hyaluronic acid hydrogels combined with adipose stem cells, the construction of printable bioinks showed good chondrogenic properties in vitro (Nedunchezian et al., 2021). Pre-vascularized stem cell patch fabricated by 3D printing can facilitate rapid vascularization to improve the therapeutic effect of cardiac repair (Jang et al., 2017). Macrophages, as white blood cells with a wide range of protection, not only exist in the blood, but are also distributed throughout the body. The 3D bioprinted scaffolds containing live macrophages and antibiotics have been demonstrated to promote the clearance of Staphylococcus aureus craniotomy-associated biofilm infections (Aldrich et al., 2019). Because macrophages are easy to obtain, culture and purify, they have great potential for the repair of various tissues in 3D bioprinting. The neuron is the most basic structural and functional unit of the nervous system, and its function is to receive stimulation, generate excitation and conduct excitation. Neuron damage, which often causes irreversible damage to patients, has always been a major clinical difficulty. By formulating specific neural tissue bioinks, printing neuronal structures and precisely controlling the microenvironment in 3D space, it may be a new therapy that can be realized in the future (Knowlton et al., 2018).
Almost all cells in the human body can be used for bioprinting, and other common ones such as muscle cells, T cells, and osteoblasts have been extensively studied. The main difficulty of 3D printing cells is still maintaining cell activity and their original functions, which requires further reform and improvement of 3D printing technology to achieve the desired therapeutic effect.
3.3 Bioactive Molecules
Bioactive molecules, that is, compounds with biological activity, refer to trace or small amounts of substances that have an impact on life phenomena, including polysaccharides, alkaloids, peptides, nucleic acids, proteins, amino acids, vitamins, etc. Natural bioactive molecules have physiological activities such as anti-inflammatory, anti-cancer and antioxidant, and are widely distributed in a variety of animals, plants, marine organisms and microorganisms. At present, the bioactive molecules applied in 3D bioprinting are mainly growth factors, enzymes, antibodies, antigens, plasmids, DNA, etc. (Table 2). These bioactive molecules can be selected according to the type of tissues to be repaired and the functions to be achieved in 3D bioprinting, to achieve the ideal repair effect. The following is a brief introduction to several bioactive molecules commonly used in 3D bioprinting and their roles in printed products.
Enzymes are proteins or RNAs produced by living cells that are highly specific and catalytically efficient for their substrates. Printing enzymes mixed with other polymer materials is a common method to improve their stability, activity and biomedical applications (Steier et al., 2020). A bioink prepared by mixing gelatin methacrylamide, tyrosinase and collagen has been studied for in vivo skin tissue bioprinting. The experimental results show that stable 3D living structures could be printed, and the three main skin cell lines all show high survival rates (Shi et al., 2018). Growth factor, as a class of substances that can regulate the growth and development of cells and the human body, has been widely used in tissue engineering and 3D bioprinting. It plays a very vital role in the treatment of bone, digestion, blood, immunity and respiratory diseases. Researchers have used a custom-made bioprinter to print muscle ink made from gelatin methacrylamide and VEGF-eluting Laponite particles directly onto the site of volumetric muscle loss injury. The muscle ink has been shown to promote muscle function recovery and reduce fibrosis when printed in vivo, paving the way for the treatment of other soft tissue traumas (Quint et al., 2021). DNA is an essential biological macromolecule for the development and normal operation of organisms. It is widely used in modern biology and biochemistry, mainly in the field of genetic engineering. However, it can also be applied in 3D bioprinting to prepare gene-active bioinks and combine with bone marrow mesenchymal stem cells, providing a new idea for the treatment of bone defect regeneration (Cunniffe et al., 2017).
Numerous studies have confirmed that loading cells and/or bioactive molecules during the 3D bioprinting process can improve cell viability and regulate the microenvironment to promote tissue repair and regeneration. All 3D bioprinting manufacturing processes must meet biological standards, ensure cell activity, tissue function and meet medical standards. This requires extensive experimentation to obtain various optimal combinations of biomaterials, cells and growth factors for each organ.
4 Typical Application of 3D Bioprinted Scaffolds for Tissue Repair or Regeneration
4.1 3D Bioprinted Nerve Guidance Conduits
Peripheral nerve injury is a common neurosurgical disease, which is mainly caused by trauma or neurodegenerative diseases (Soman and Vijayavenkataraman, 2020). After peripheral nerve injury, it will lead to movement, sensation and nutrition damage in the innervated area (Wu et al., 2021). At present, the “gold standard of clinical treatment” is autologous nerve transplantation (Paprottka et al., 2013). However, this approach has many limitations, such as nerve torsion, dislocation and loss of donor nerve function. Artificial nerve catheters based on biomedical materials have become a promising alternative method for peripheral nerve regeneration. Among plenty of tissue engineering methods, 3D bioprinting has attracted much attention because it can realize personalized printing and accurate 3D structure, which immensely enhances the possibility of nerve defect repair (Li Q. et al., 2019).
Both biochemistry and topology are the keys to axon guidance and nerve regeneration. However, building a perfect platform that can integrate biochemical gradients and topographic guidance cues is still challenging in the 3D scaffold. Studies have combined improved 3D printing technology with directional freezing technology to construct a 3D scaffold with longitudinally oriented microchannels and continuous biochemical gradients. In vitro experiments and in vivo studies have shown that the scaffold can synergistically facilitate nerve regeneration and directed extension, and further accelerate the recovery of nerve function (Huang et al., 2020). To further functionalize the 3D scaffolds, researchers have used 3D bioprinting technology to construct nerve guidance conduits (NGCs) with bionic natural peripheral nerve structures. NGCs have been proven to provide structural and nutritional support for the nerve endings at both ends, supporting the invasion of surrounding tissues and the regeneration of axons along the conduit (Rodriguez-Sanchez et al., 2021). The sciatic nerve defect was reconstructed using a collagen hydrogel poly(lactide-co-caprolactone) (PLCL) elastic NGC with directional alignment and nanoporous structure developed by 3D printing technology and electrospinning. The elastic NGC has been confirmed to obtain a good effect on axon regeneration and remyelination in a rat sciatic nerve injury model (Figures 3A,B) (Yoo et al., 2020). Currently, some NGCs have been approved by the FDA for clinical applications. Schwann cells are the dominating glial cells in the peripheral nervous system. They could secrete neurotrophic factors, promote the survival of damaged neurons and the regeneration of axons, and participate in the formation of nerve fibers in the peripheral nervous system. Based on these superior properties of Schwann cells, the preparation of 3D scaffolds containing live Schwann cells by 3D bioprinting technology has been widely studied. Relevant experimental evidence proves that tissue scaffolds with Schwann cells can be used as a substitute for autologous transplantation and have great potential in peripheral nerve tissue repair (Ning et al., 2018). These results lay the foundation for the invention of advanced instruments for the treatment of serious system damage.
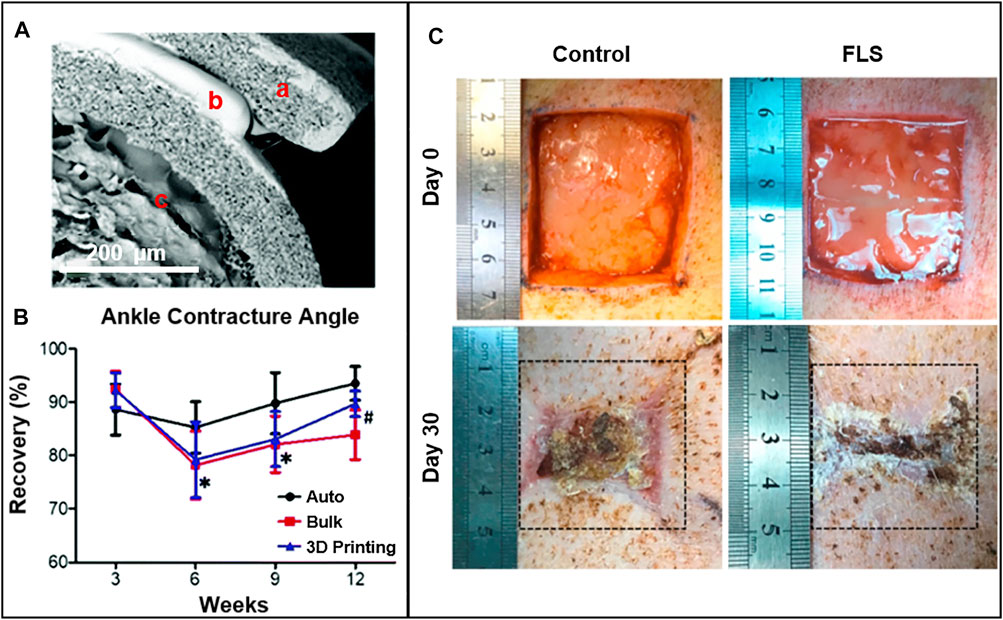
FIGURE 3. (A) The SEM of 3D printing nerve guidance conduit afterfreeze-drying. Reproduced with permission (Yoo et al., 2020). Copyright 2020, Royal Society of Chemistry. (B) Results of ankle contracture angle of autograft, bulk and 3D printing groups measured at 3, 6, 9, and 12 weeks following nerve reconstruction. *p < 0.05 autograft group versus 3D printing group; #p < 0.05 bulk group versus 3D printing group. Reproduced with permission (Yoo et al., 2020). Copyright 2020, Royal Society of Chemistry. (C) Functional living skin (FLS) for full-thickness skin defect repair in a pig model. Gross morphology of the wound healing process within 30 days. Reproduced with permission (Zhou et al., 2020). Copyright 2020, Elsevier.
4.2 3D Bioprinted Wound Dressing
As the largest organ of the human body, the skin is in direct contact with the external environment and has the functions of protecting, excreting, regulating body temperature and feeling external stimuli (Talikowska et al., 2019). However, the skin is vulnerable to external pathogenic microorganisms, accidents or physical injuries, resulting in injuries that cannot heal themselves (Rastin et al., 2021). To deal with this situation, autologous grafts and other skin tissue substitutes are selected clinically, but their availability is limited and may cause secondary injury to the human body; Allogeneic transplantation, use may lead to severe immune rejection (Vig et al., 2017; Hu and Xu, 2020). The appearance of tissue engineered skin substitutes provides new ideas for the repair and regeneration of skin tissues. Among the numerous biomanufacturing methods, 3D bioprinting relies on its strong ability to control complex 3D structures to produce functional 3D tissue scaffolds, which have attracted special attention (Cubo et al., 2016).
3D bioprinting bionic medical dressings are a hot method for wound healing. The role of this dressing is to transport and release diverse types of cells or key biomolecules required in the process of wound healing, so as to accelerate the wound healing process. Some researchers have designed a novel medical grade PCL fiber wound dressing, which is prepared by 3D bioprinting, has anisotropic mechanical properties and is loaded with human gingival mesenchymal stem cells. In the rat injury model, the wound healing rate of this dressing group is significantly higher than other groups, and the scar formation of the full-thickness excision wound is reduced (Shafiee et al., 2021). However, if there is extensive skin damage, simple dressings cannot meet the rehabilitation needs of patients, which leads to the development of skin tissue substitutes. A new method for printing functional living skin (FLS) with new bionic bioinks and 3D bioprinting was developed. FLS can significantly promote the formation of skin appendages (such as hair follicles, sebaceous glands). It is a promising approach to achieve scar-free skin regeneration. FLS with the physiological structure of natural skin, can promote skin and blood vessel regeneration, and because of the mechanical properties and bioadhesive properties, it is convenient to be processed and implanted into the wound surface. This brings new hope for the treatment of large-area skin defects in the future (Figure 3C) (Zhou et al., 2020). Common bioengineered skins have relatively simple tissue structures. Creating porous layered collagen structures and co-printing fibroblasts, melanocytes and keratinocytes can induce precise pigmentation (Ng et al., 2018). In addition, the application of 3D bioprinting dressings is not only limited to the repair and regeneration of skin tissues, but also can be used in other tissues, such as the oral cavity, and nasal mucosa.
4.3 3D Bioprinted Blood Vessel
The blood vessel is the biological blood transport pipeline, which is responsible for the heartbeat blood to all tissues and organs of the body, to meet the various nutrients required by the body’s activities, and the metabolic end products (or waste) back to the heart, through the lung, kidney and other organs discharged from the body. Vascular proliferation in vivo refers to the proliferation and migration of endothelial cells to generate new microvessels from the existing vascular network, which is critical for tissue regeneration, development and repair (Nulty et al., 2021). Vascularization is an extremely slow process, and the average growth rate of new capillaries is only 5 μm/h (Utzinger et al., 2015). This has prompted tissue engineers to turn their attention to the design and preparation of a capillary-like network scaffold, or engineering tissue that accelerate the process of vascularization through 3D bioprinting (Sharma et al., 2019). In large diameter vessels such as aortic (>8 mm) and carotid arteries (6–8 mm), vascular grafts have satisfactory therapeutic effects. But in small diameter vessels such as coronary artery (<6 mm) are prone to thrombosis and intimal hyperplasia, and printing small-diameter vascular grafts is a priority. Gladys et al. designed PCL/polydioxanone (PDO) small-diameter bilayer nanofibrous vascular structures by 3D printing, which is able to achieve the required printing resolution for blood vessels (Datta et al., 2017; Emechebe et al., 2020).
There is always a certain gap in the complexity and biological function of biomimetic vascular tissue in various existing tissue engineering technologies. The ideal substitute for tissue-engineered blood vessels requires suture in the structure; It is consistent with natural blood vessels in terms of mechanical properties; Functionally, it can withstand the high pressure generated by blood flow, provide sufficient gas and nutrients for cells, and finally recruit endogenous cells to promote angiogenesis. There have been studies using 3D bioprinting to successfully construct an ice scaffold coated with natural or synthetic polymers to create a customizable, independent and biocompatible vascular. The results showed that the optimized vascular had obvious advantages in mechanical properties and functions compared with natural mammalian blood vessels, which is conducive to vascular reconstruction or regeneration (Yeo, 2019). However, due to the size and complexity of functional microvessels, the preparation of their corresponding substitutes has always been very challenging. The generation of blood vessels is often the key to another tissue repair. The dimethyloxallyl glycine (DMOG) scaffold composed of mesoporous bioactive glasses and poly(3-hydroxybutyrate-co-3-hydroxyhexanoate) polymers (MPHS scaffolds), could effectively induce the hypoxic microenvironment of human bone marrow stromal cells (hBMSC). In vivo studies have observed that the scaffold could significantly promote angiogenesis and larger bone formation area in the defect tissue (Figure 4A) (Min et al., 2015). Researchers have used improved thermal inkjet bioprinting to synchronously print human microvascular endothelial cells and fibrin scaffolds to fabricate microvessels. They want to use it in coronary artery bypass grafting, but the idea has not been implemented due to the performance of the blood vessels themselves (Ameri et al., 2017). In addition to the usual 3D printing technology, fresh technologies like co-axial nozzle bioprinting are also being used to print blood vessels. Co-axial nozzle device containing two syringes, the core layer is connected to the bio-ink, and the shell layer is connected to the ionic crosslinking agent, realizing the printing of hollow biological vessels (Jia et al., 2016). Furthermore, 3D bioprinting technology has also been used to build various in vitro tissue or organ models. An independent in vitro vascular model was developed by using coaxial cell printing and specific bioinks. It is conducive to the integration of mechanical and biochemical signals in biomedical applications, and has broad application prospects in future vascular tissue engineering applications (Gao et al., 2018).
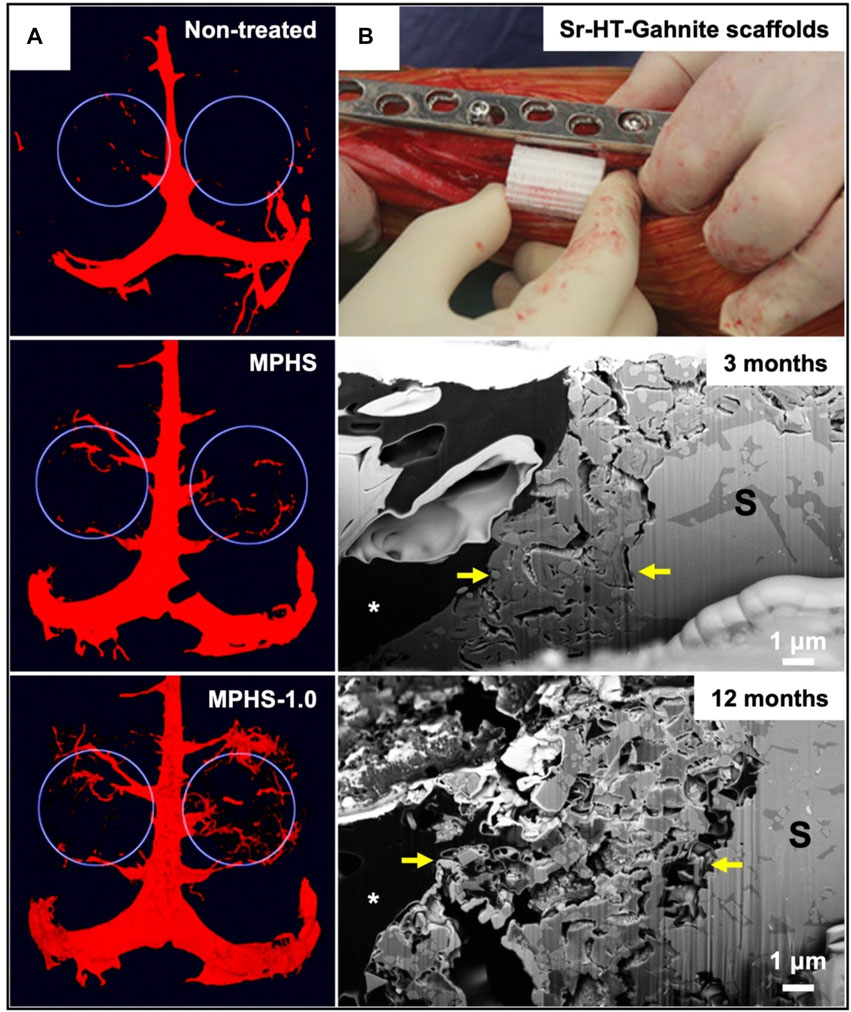
FIGURE 4. (A) Microscopic CT evaluation of vascular reconstruction in the defect area (showing white circles) at 8 weeks after implantation of MPHS and MPHS-DMOG scaffolds. Reproduced with permission (Min et al., 2015). Copyright 2015, Royal Society of Chemistry. (B) The surgical procedure for implanting the 3D printed bioactive ceramic scaffold into the bone defect and FIB-SEM shows the cross section of the implant bone interface. A broken boundary (between the yellow arrows) represents a ceramic disintegration area. At 12 months, the boundary became more prominent and infiltrated by loose connective tissue. Reproduced with permission (Li JJ. et al., 2019). Copyright 2019, Wiley.
4.4 3D Bioprinted Bone/Cartilage
The structure of bone includes: periosteum, bone and marrow. Cartilage consists of cartilage tissue and its surrounding perichondrium. Cartilage tissue is composed of chondrocytes, matrix and collagen fibers (Li N. et al., 2021). There are no blood vessels and lymphatic vessels in cartilage. Nutrients penetrate the intercellular matrix from the blood vessels of the perichondrium and then nourish the osteocytes. This histological feature greatly limits the self-regeneration of damaged cartilage. Therefore, the problem of damage repair has been perplexing clinicians and researchers (Qi et al., 2018). Bone defects are usually caused by trauma and tumor resection. Serious defects exceed the regenerative capacity of the original tissue. The treatment strategy for bone defects is mainly bone grafting to repair bone defects, and bone transplantation has been proved insufficient to reconstruct and regenerate these defects (Mostafavi et al., 2021). In the context of bone tissue engineering, physical and biological needs play a key role in the successful construction and implantation and the formation of bone, cartilage and vascular tissue. However, meeting these requirements to simulate the complexity and function of human tissue remains a major challenge. Recent studies have shown that the combination of 3D bioprinting technology and advanced biomaterials exhibits huge potential in bone tissue engineering (Alcala-Orozco et al., 2021).
Research on substitutes with high strength and high biological activity for repairing large bone/cartilage injuries has been always a severe and realistic difficulty facing the clinic. The emergence of 3D bioprinting has made it possible to print patient-specific bone/cartilage tissue. A 3D printed bioactive ceramic scaffold without cells or growth factors was prepared. The structure and biomechanics of repairing defects of sheep long bone injury were evaluated for a long time. The results show that the scaffold has strong mechanical strength and good bone regeneration ability (Figure 4B) (Li JJ. et al., 2019). In addition, a recent study has prepared a chitosan hydrogel/3D for printing PCL and synovial mesenchymal stem cells (SMSCs) hybrid scaffolds. The scaffold had certain mechanical supportability, can provide an excellent microenvironment for the proliferation and cartilage differentiation of the SMSCs, and is conducive to cartilage regeneration (Li P. et al., 2021). However, bone damage is often accompanied by cartilage injury. So far, there is no clinical alternative to repairing bone and cartilage at the same time. The 3D printed nanoelastomer scaffold with thermo-responsive mechanical propertihasave been reported previously. The chondrogenic differentiation of human bone marrow mesenchymal stem cells on soft scaffolds was enhanced, and the osteogenic differentiation on hard scaffolds was enhanced, which was similar to the relative expression of human femoral head tissue (Wu et al., 2018). Acrylic peptides and PEG hydrogels of human mesenchymal stem cells were successfully printed by reducing the manipulation steps by inkjet bioprinting technology, which can promote the formation of bone and cartilage (Gao et al., 2015). Perhaps, this can open up new ideas for the development and research of totipotency artificial bone/cartilage substitutes.
4.5 3D Bioprinted Heart Valves, Urethra and Esophagus
The application of 3D bioprinting is limited to the repair and regeneration of the issues mentioned above and has been studied in various human tissue structures such as heart valves, urethra, and esophagus. For example, the only available and effective treatment for valvular heart disease is prosthetic valve replacement (Cheung et al., 2015). Tissue engineering and 3D bioprinting technology can design specific functional valves according to patients’ diseased valves, to make up for some shortcomings of prosthetic valve replacement (Vashistha et al., 2019). Urethral stricture caused by urethral injury has always been a difficult clinical problem, and the traditional urethral tissue engineering and treatment methods cannot meet the needs of treatment. Relevant research has shown that the urethra prepared by 3D bioprinting can simulate the mechanical properties and structure of human urethral tissue, which provides a new therapeutic strategy for the field of urology (Zhang et al., 2017; Agung et al., 2021). Various esophageal diseases, such as esophageal stenosis, cancer, atresia, etc., although the clinical treatment has a certain effect, may lead to many complications (Farhat et al., 2021). 3D bioprinting enables the creation of substitutes that mimic the original esophageal structure and intrinsic composition for esophageal defect repair (Takeoka et al., 2019; Nam et al., 2020). There is no doubt that the emergence of 3D bioprinting will provide multiple possibilities for tissue engineering and regenerative medicine. We summarized the applications of 3D printing technology, as shown in Table 3.
5 Conclusion and Outlook
The repair and regeneration of damaged tissue is a major issue in the fields of biology and medicine, involving plenty of basic disciplines such as genetics, development, stem cell tissue engineering, biomaterials and clinical disciplines such as trauma, burns and orthopedics. The results will provide direct services for the treatment and rehabilitation of hundreds of millions of injured patients and improve their quality of life. At present, 3D printing technology has become a promising technology in the field of healthcare, and remarkable progress has been made in printing various complex bionic structures. Meanwhile, emerging strategies such as hybrid bioprinting, which combines biomaterials, cell microenvironments and 3D printing technology, also show wide application possibilities (Moor et al., 2020). 3D bioprinting relies on the complexity of controlling the distribution of cells and biological materials to reconstruct human tissues, which has unparalleled advantages in preparing tissue engineering scaffolds (Kolesky et al., 2016). Although a lot of research results have been achieved in the past 10 years, there is still a very large space for improvement in the construction of bioprinting and bioinks. There is short of bioinks that can meet the requirements of 3D printing and tissue engineering, and the potential applications of bioprinting have been restricted (Chimene et al., 2016). For example, decellularized extracellular matrix (dECM) inks formed by removing cells from tissue and retaining ECM have good biochemical properties, but lack mechanical properties (Abaci and Guvendiren, 2020). Compared with nonbioprinting, 3D bioprinting is more complex and needs to overcome the challenges related to material selection, cell type, growth and differentiation factors, as well as the sensitivity and tissue structure of living cells.
Future bioprinting strategies may pursue the early stages of tissue and organ development. The continuous improvement and integration of cell biology, material science, engineering and many other disciplines will gradually overcome the obstacles encountered by bioprinting in tissue repair and regeneration (Mota et al., 2020). For example, deep learning has the advantages of parameter optimization and image processing, which can simplify the bioprinting process and is expected to promote the progress of 3D printing technology (Ng et al., 2020). Most importantly, 3D bioprinted tissues or organs need to meet a lot of requirements before they can be applied in clinical practice. For example, they need to have biological properties similar to natural organs and have a complete blood vessel network including arteries, veins and capillaries. To achieve a breakthrough in the application of multi-scale and multi-component tissue/organ bioprinting, it is necessary to further develop “intelligent” biomaterials and integrate complementary bioprinting technologies based on existing research.
Author Contributions
TW and YW discussed and developed this review. NL, XZ, and QG wrote the original draft. TW and YW reviewed and edited the manuscript. All authors have contributed to the work and approved the final version of the manuscript.
Funding
The authors thank the support from Qingdao health science and technology plan project (2020-WJZD143), the startup funding of Qingdao stomatological hospital (2020QN01), natural science foundation of Shandong province (ZR2021QC063), Qingdao clinical research center for oral diseases (22-3-7-lczx-7-nsh) and Qingdao key health discipline development fund (2020-2022).
Conflict of Interest
The authors declare that the research was conducted in the absence of any commercial or financial relationships that could be construed as a potential conflict of interest.
Publisher’s Note
All claims expressed in this article are solely those of the authors and do not necessarily represent those of their affiliated organizations, or those of the publisher, the editors and the reviewers. Any product that may be evaluated in this article, or claim that may be made by its manufacturer, is not guaranteed or endorsed by the publisher.
References
Abaci, A., and Guvendiren, M. (2020). Designing Decellularized Extracellular Matrix‐Based Bioinks for 3D Bioprinting. Adv. Healthc. Mat. 9, 2000734. doi:10.1002/adhm.202000734
Abdollahiyan, P., Oroojalian, F., Hejazi, M., De La Guardia, M., and Mokhtarzadeh, A. (2021). Nanotechnology, and Scaffold Implantation for the Effective Repair of Injured Organs: An Overview on Hard Tissue Engineering. J. Control. Release 333, 391–417. doi:10.1016/j.jconrel.2021.04.003
Agarwal, T., Chiesa, I., Presutti, D., Irawan, V., Vajanthri, K. Y., Costantini, M., et al. (2021). Recent Advances in Bioprinting Technologies for Engineering Different Cartilage-Based Tissues. Mater. Sci. Eng. C 123, 112005. doi:10.1016/j.msec.2021.112005
Agung, N. P., Nadhif, M. H., Irdam, G. A., and Mochtar, C. A. (2021). The Role of 3D-Printed Phantoms and Devices for Organ-Specified Appliances in Urology. Int. J. Bioprint 7, 333. doi:10.18063/ijb.v7i2.333
Alcala-Orozco, C. R., Cui, X., Hooper, G. J., Lim, K. S., and Woodfield, T. B. F. (2021). Converging Functionality: Strategies for 3D Hybrid-Construct Biofabrication and the Role of Composite Biomaterials for Skeletal Regeneration. Acta Biomater. 132, 188–216. doi:10.1016/j.actbio.2021.03.008
Aldrich, A., Kuss, M. A., Duan, B., and Kielian, T. (2019). 3D Bioprinted Scaffolds Containing Viable Macrophages and Antibiotics Promote Clearance of Staphylococcus Aureus Craniotomy-Associated Biofilm Infection. ACS Appl. Mat. Interfaces 11, 12298–12307. doi:10.1021/acsami.9b00264
Ameri, K., Samurkashian, R., and Yeghiazarians, Y. (2017). Three-Dimensional Bioprinting. Circulation 135, 1281–1283. doi:10.1161/circulationaha.116.024945
Amler, A. K., Dinkelborg, P. H., Schlauch, D., Spinnen, J., Stich, S., Lauster, R., et al. (2021). Comparison of the Translational Potential of Human Mesenchymal Progenitor Cells from Different Bone Entities for Autologous 3D Bioprinted Bone Grafts. Int. J. Mol. Sci. 22, 796. doi:10.3390/ijms22020796
Angelopoulos, I., Allenby, M. C., Lim, M., and Zamorano, M. (2020). Engineering Inkjet Bioprinting Processes toward Translational Therapies. Biotechnol. Bioeng. 117, 272–284. doi:10.1002/bit.27176
Apelgren, P., Amoroso, M., Säljö, K., Montelius, M., Lindahl, A., Stridh Orrhult, L., et al. (2021). Vascularization of Tissue Engineered Cartilage - Sequential In Vivo MRI Display Functional Blood Circulation. Biomaterials 276, 121002. doi:10.1016/j.biomaterials.2021.121002
Ashammakhi, N., Ahadian, S., Pountos, I., Hu, S.-K., Tellisi, N., Bandaru, P., et al. (2019). In Situ Three-Dimensional Printing for Reparative and Regenerative Therapy. Biomed. Microdevices 21, 42. doi:10.1007/s10544-019-0372-2
Azad, M. A., Olawuni, D., Kimbell, G., Badruddoza, A. Z. M., Hossain, M. S., and Sultana, T. (2020). Polymers for Extrusion-Based 3D Printing of Pharmaceuticals: A Holistic Materials-Process Perspective. Pharmaceutics 12, 124. doi:10.3390/pharmaceutics12020124
Baniasadi, H., Madani, Z., Ajdary, R., Rojas, O. J., and Seppälä, J. (2021). Ascorbic Acid-Loaded Polyvinyl Alcohol/Cellulose Nanofibril Hydrogels as Precursors for 3D Printed Materials. Mater. Sci. Eng. C 130, 112424. doi:10.1016/j.msec.2021.112424
Bardot, M., and Schulz, M. D. (2020). Biodegradable Poly(Lactic Acid) Nanocomposites for Fused Deposition Modeling 3D Printing. Nanomaterials 10, 2567. doi:10.3390/nano10122567
Bedell, M. L., Navara, A. M., Du, Y., Zhang, S., and Mikos, A. G. (2020). Polymeric Systems for Bioprinting. Chem. Rev. 120, 10744–10792. doi:10.1021/acs.chemrev.9b00834
Bhatt, A., and Anbarasu, A. (2017). Nanoscale Biomaterials for 3D Printing. Iosrjpbs 12, 80–86. doi:10.9790/3008-1203068086
Billiet, T., Gevaert, E., De Schryver, T., Cornelissen, M., and Dubruel, P. (2014). The 3D Printing of Gelatin Methacrylamide Cell-Laden Tissue-Engineered Constructs with High Cell Viability. Biomaterials 35, 49–62. doi:10.1016/j.biomaterials.2013.09.078
Blaeser, A., Duarte Campos, D. F., Puster, U., Richtering, W., Stevens, M. M., and Fischer, H. (2016). Controlling Shear Stress in 3D Bioprinting Is a Key Factor to Balance Printing Resolution and Stem Cell Integrity. Adv. Healthc. Mat. 5, 326–333. doi:10.1002/adhm.201500677
Bouhadir, K. H., Lee, K. Y., Alsberg, E., Damm, K. L., Anderson, K. W., and Mooney, D. J. (2001). Degradation of Partially Oxidized Alginate and its Potential Application for Tissue Engineering. Biotechnol. Prog. 17, 945–950. doi:10.1021/bp010070p
Brézulier, D., Chaigneau, L., Jeanne, S., and Lebullenger, R. (2021). The Challenge of 3D Bioprinting of Composite Natural Polymers PLA/Bioglass: Trends and Benefits in Cleft Palate Surgery. Biomedicines 9, 1553. doi:10.3390/biomedicines9111553
Camacho, P., Behre, A., Fainor, M., Seims, K. B., and Chow, L. W. (2021). Spatial Organization of Biochemical Cues in 3D-Printed Scaffolds to Guide Osteochondral Tissue Engineering. Biomater. Sci. 9, 6813–6829. doi:10.1039/d1bm00859e
Chae, S., Lee, S.-S., Choi, Y.-J., Hong, D. H., Gao, G., Wang, J. H., et al. (2021). 3D Cell-Printing of Biocompatible and Functional Meniscus Constructs Using Meniscus‐derived Bioink. Biomaterials 267, 120466. doi:10.1016/j.biomaterials.2020.120466
Chan, G., and Mooney, D. J. (2013). Ca2+ Released from Calcium Alginate Gels Can Promote Inflammatory Responses In Vitro and In Vivo. Acta Biomater. 9, 9281–9291. doi:10.1016/j.actbio.2013.08.002
Chen, J., Huang, D., Wang, L., Hou, J., Zhang, H., Li, Y., et al. (2020). 3D Bioprinted Multiscale Composite Scaffolds Based on Gelatin Methacryloyl (Gelma)/Chitosan Microspheres as a Modular Bioink for Enhancing 3D Neurite Outgrowth and Elongation. J. Colloid Interface Sci. 574, 162–173. doi:10.1016/j.jcis.2020.04.040
Chen, X., Yue, Z., Winberg, P. C., Lou, Y.-R., Beirne, S., and Wallace, G. G. (2021). 3D Bioprinting Dermal-like Structures Using Species-specific Ulvan. Biomater. Sci. 9, 2424–2438. doi:10.1039/d0bm01784a
Cheng, L., Suresh K, S., He, H., Rajput, R. S., Feng, Q., Ramesh, S., et al. (2021). 3D Printing of Micro- and Nanoscale Bone Substitutes: A Review on Technical and Translational Perspectives. Ijn Vol. 16, 4289–4319. doi:10.2147/ijn.s311001
Chereddy, K. K., Payen, V. L., and Préat, V. (2018). PLGA: From a Classic Drug Carrier to a Novel Therapeutic Activity Contributor. J. Control. Release 289, 10–13. doi:10.1016/j.jconrel.2018.09.017
Cheung, D. Y., Duan, B., and Butcher, J. T. (2015). Current Progress in Tissue Engineering of Heart Valves: Multiscale Problems, Multiscale Solutions. Expert Opin. Biol. Ther. 15, 1155–1172. doi:10.1517/14712598.2015.1051527
Chia, H. N., and Wu, B. M. (2015). Recent Advances in 3D Printing of Biomaterials. J. Biol. Eng. 9, 4. doi:10.1186/s13036-015-0001-4
Chimene, D., Lennox, K. K., Kaunas, R. R., and Gaharwar, A. K. (2016). Advanced Bioinks for 3D Printing: A Materials Science Perspective. Ann. Biomed. Eng. 44, 2090–2102. doi:10.1007/s10439-016-1638-y
Cidonio, G., Glinka, M., Dawson, J. I., and Oreffo, R. O. C. (2019). The Cell in the Ink: Improving Biofabrication by Printing Stem Cells for Skeletal Regenerative Medicine. Biomaterials 209, 10–24. doi:10.1016/j.biomaterials.2019.04.009
Clark, E. A., Alexander, M. R., Irvine, D. J., Roberts, C. J., Wallace, M. J., Sharpe, S., et al. (2017). 3D Printing of Tablets Using Inkjet with UV Photoinitiation. Int. J. Pharm. 529, 523–530. doi:10.1016/j.ijpharm.2017.06.085
Cubo, N., Garcia, M., del Cañizo, J. F., Velasco, D., and Jorcano, J. L. (2016). 3D Bioprinting of Functional Human Skin: Production and In Vivo Analysis. Biofabrication 9, 015006. doi:10.1088/1758-5090/9/1/015006
Cunniffe, G. M., Gonzalez-Fernandez, T., Daly, A., Sathy, B. N., Jeon, O., Alsberg, E., et al. (2017). Three-Dimensional Bioprinting of Polycaprolactone Reinforced Gene Activated Bioinks for Bone Tissue Engineering. Tissue Eng. Part A 23, 891–900. doi:10.1089/ten.tea.2016.0498
da Silva, D., Kaduri, M., Poley, M., Adir, O., Krinsky, N., Shainsky-Roitman, J., et al. (2018). Biocompatibility, Biodegradation and Excretion of Polylactic Acid (PLA) in Medical Implants and Theranostic Systems. Chem. Eng. J. 340, 9–14. doi:10.1016/j.cej.2018.01.010
Daly, A. C., Prendergast, M. E., Hughes, A. J., and Burdick, J. A. (2021). Bioprinting for the Biologist. Cell. 184, 18–32. doi:10.1016/j.cell.2020.12.002
Danhier, F., Ansorena, E., Silva, J. M., Coco, R., Le Breton, A., and Préat, V. (2012). PLGA-based Nanoparticles: An Overview of Biomedical Applications. J. Control. Release 161, 505–522. doi:10.1016/j.jconrel.2012.01.043
Datta, P., Ayan, B., and Ozbolat, I. T. (2017). Bioprinting for Vascular and Vascularized Tissue Biofabrication. Acta Biomater. 51, 1–20. doi:10.1016/j.actbio.2017.01.035
Du, B., Yin, H., Chen, Y., Lin, W., Wang, Y., Zhao, D., et al. (2020). A Waterborne Polyurethane 3D Scaffold Containing PLGA with a Controllable Degradation Rate and an Anti-inflammatory Effect for Potential Applications in Neural Tissue Repair. J. Mat. Chem. B 8, 4434–4446. doi:10.1039/d0tb00656d
Emechebe, G. A., Obiweluozor, F. O., Jeong, I. S., Park, J.-K., Park, C. H., and Kim, C. S. (2020). Merging 3D Printing with Electrospun Biodegradable Small-Caliber Vascular Grafts Immobilized with VEGF. Nanomedicine Nanotechnol. Biol. Med. 30, 102306. doi:10.1016/j.nano.2020.102306
Farahani, R. D., Dubé, M., and Therriault, D. (2016). Three-Dimensional Printing of Multifunctional Nanocomposites: Manufacturing Techniques and Applications. Adv. Mat. 28, 5794–5821. doi:10.1002/adma.201506215
Farhat, W., Chatelain, F., Marret, A., Faivre, L., Arakelian, L., Cattan, P., et al. (2021). Trends in 3D Bioprinting for Esophageal Tissue Repair and Reconstruction. Biomaterials 267, 120465. doi:10.1016/j.biomaterials.2020.120465
Fonseca, A. C., Melchels, F. P. W., Ferreira, M. J. S., Moxon, S. R., Potjewyd, G., Dargaville, T. R., et al. (2020). Emulating Human Tissues and Organs: A Bioprinting Perspective toward Personalized Medicine. Chem. Rev. 120, 11093–11139. doi:10.1021/acs.chemrev.0c00342
Gao, C., Lu, C., Jian, Z., Zhang, T., Chen, Z., Zhu, Q., et al. (2021). 3D Bioprinting for Fabricating Artificial Skin Tissue. Colloids Surfaces B Biointerfaces 208, 112041. doi:10.1016/j.colsurfb.2021.112041
Gao, G., Park, J. Y., Kim, B. S., Jang, J., and Cho, D. W. (2018). Coaxial Cell Printing of Freestanding, Perfusable, and Functional In Vitro Vascular Models for Recapitulation of Native Vascular Endothelium Pathophysiology. Adv. Healthc. Mater 7, e1801102. doi:10.1002/adhm.201801102
Gao, G., Yonezawa, T., Hubbell, K., Dai, G., and Cui, X. (2015). Inkjet-Bioprinted Acrylated Peptides and PEG Hydrogel with Human Mesenchymal Stem Cells Promote Robust Bone and Cartilage Formation with Minimal Printhead Clogging. Biotechnol. J. 10, 1568–1577. doi:10.1002/biot.201400635
Gao, J., Ding, X., Yu, X., Chen, X., Zhang, X., Cui, S., et al. (2021). Cell-Free Bilayered Porous Scaffolds for Osteochondral Regeneration Fabricated by Continuous 3D-Printing Using Nascent Physical Hydrogel as Ink. Adv. Healthc. Mater 10, e2001404. doi:10.1002/adhm.202001404
Gold, K. A., Saha, B., Rajeeva Pandian, N. K., Walther, B. K., Palma, J. A., Jo, J., et al. (2021). 3D Bioprinted Multicellular Vascular Models. Adv. Healthc. Mater 10, e2101141. doi:10.1002/adhm.202101141
Gradwohl, M., Chai, F., Payen, J., Guerreschi, P., Marchetti, P., and Blanchemain, N. (2021). Effects of Two Melt Extrusion Based Additive Manufacturing Technologies and Common Sterilization Methods on the Properties of a Medical Grade PLGA Copolymer. Polym. (Basel) 13, 572. doi:10.3390/polym13040572
Groll, J., Boland, T., Blunk, T., Burdick, J. A., Cho, D.-W., Dalton, P. D., et al. (2016). Biofabrication: Reappraising the Definition of an Evolving Field. Biofabrication 8, 013001. doi:10.1088/1758-5090/8/1/013001
Gu, Z., Fu, J., Lin, H., and He, Y. (2020). Development of 3D Bioprinting: From Printing Methods to Biomedical Applications. Asian J. Pharm. Sci. 15, 529–557. doi:10.1016/j.ajps.2019.11.003
Gudapati, H., Dey, M., and Ozbolat, I. (2016). A Comprehensive Review on Droplet-Based Bioprinting: Past, Present and Future. Biomaterials 102, 20–42. doi:10.1016/j.biomaterials.2016.06.012
Hong, H., Hu, J., and Yan, X. (2019). UV Curable Conductive Ink for the Fabrication of Textile-Based Conductive Circuits and Wearable UHF RFID Tags. ACS Appl. Mat. Interfaces 11, 27318–27326. doi:10.1021/acsami.9b06432
Hu, H., and Xu, F.-J. (2020). Rational Design and Latest Advances of Polysaccharide-Based Hydrogels for Wound Healing. Biomater. Sci. 8, 2084–2101. doi:10.1039/d0bm00055h
Huang, L., Gao, J., Wang, H., Xia, B., Yang, Y., Xu, F., et al. (2020). Fabrication of 3D Scaffolds Displaying Biochemical Gradients along Longitudinally Oriented Microchannels for Neural Tissue Engineering. ACS Appl. Mat. Interfaces 12, 48380–48394. doi:10.1021/acsami.0c15185
Jang, J., Park, H.-J., Kim, S.-W., Kim, H., Park, J. Y., Na, S. J., et al. (2017). 3D Printed Complex Tissue Construct Using Stem Cell-Laden Decellularized Extracellular Matrix Bioinks for Cardiac Repair. Biomaterials 112, 264–274. doi:10.1016/j.biomaterials.2016.10.026
Jentsch, S., Nasehi, R., Kuckelkorn, C., Gundert, B., Aveic, S., and Fischer, H. (2021). Multiscale 3D Bioprinting by Nozzle‐Free Acoustic Droplet Ejection. Small Methods 5, 2000971. doi:10.1002/smtd.202000971
Jeon, O., Lee, Y. B., Hinton, T. J., Feinberg, A. W., and Alsberg, E. (2019). Cryopreserved Cell-Laden Alginate Microgel Bioink for 3D Bioprinting of Living Tissues. Mater. Today Chem. 12, 61–70. doi:10.1016/j.mtchem.2018.11.009
Jia, W., Gungor-Ozkerim, P. S., Zhang, Y. S., Yue, K., Zhu, K., Liu, W., et al. (2016). Direct 3D Bioprinting of Perfusable Vascular Constructs Using a Blend Bioink. Biomaterials 106, 58–68. doi:10.1016/j.biomaterials.2016.07.038
Kang, D., Ahn, G., Kim, D., Kang, H.-W., Yun, S., Yun, W.-S., et al. (2018). Pre-Set Extrusion Bioprinting for Multiscale Heterogeneous Tissue Structure Fabrication. Biofabrication 10, 035008. doi:10.1088/1758-5090/aac70b
Keriquel, V., Oliveira, H., Rémy, M., Ziane, S., Delmond, S., Rousseau, B., et al. (2017). In Situ Printing of Mesenchymal Stromal Cells, by Laser-Assisted Bioprinting, for In Vivo Bone Regeneration Applications. Sci. Rep. 7, 1778. doi:10.1038/s41598-017-01914-x
Kim, H. J., Kim, U.-J., Kim, H. S., Li, C., Wada, M., Leisk, G. G., et al. (2008). Bone Tissue Engineering with Premineralized Silk Scaffolds. Bone 42, 1226–1234. doi:10.1016/j.bone.2008.02.007
Klammert, U., Gbureck, U., Vorndran, E., Rödiger, J., Meyer-Marcotty, P., and Kübler, A. C. (2010). 3D Powder Printed Calcium Phosphate Implants for Reconstruction of Cranial and Maxillofacial Defects. J. Cranio-Maxillofacial Surg. 38, 565–570. doi:10.1016/j.jcms.2010.01.009
Knowlton, S., Anand, S., Shah, T., and Tasoglu, S. (2018). Bioprinting for Neural Tissue Engineering. Trends Neurosci. 41, 31–46. doi:10.1016/j.tins.2017.11.001
Kolesky, D. B., Homan, K. A., Skylar-Scott, M. A., and Lewis, J. A. (2016). Three-Dimensional Bioprinting of Thick Vascularized Tissues. Proc. Natl. Acad. Sci. U.S.A. 113, 3179–3184. doi:10.1073/pnas.1521342113
Lee, J., Hong, J., Kim, W., and Kim, G. H. (2020). Bone-Derived Decm/Alginate Bioink for Fabricating a 3D Cell-Laden Mesh Structure for Bone Tissue Engineering. Carbohydr. Polym. 250, 116914. doi:10.1016/j.carbpol.2020.116914
Levato, R., Visser, J., Planell, J. A., Engel, E., Malda, J., and Mateos-Timoneda, M. A. (2014). Biofabrication of Tissue Constructs by 3D Bioprinting of Cell-Laden Microcarriers. Biofabrication 6, 035020. doi:10.1088/1758-5082/6/3/035020
Li, J. J., Dunstan, C. R., Entezari, A., Li, Q., Steck, R., Saifzadeh, S., et al. (2019). A Novel Bone Substitute with High Bioactivity, Strength, and Porosity for Repairing Large and Load-Bearing Bone Defects. Adv. Healthc. Mater 8, e1801298. doi:10.1002/adhm.201801298
Li, N., Guo, R., and Zhang, Z. J. (2021). Bioink Formulations for Bone Tissue Regeneration. Front. Bioeng. Biotechnol. 9, 630488. doi:10.3389/fbioe.2021.630488
Li, P., Fu, L., Liao, Z., Peng, Y., Ning, C., Gao, C., et al. (2021). Chitosan hydrogel/3D-Printed Poly(ε‐caprolactone) Hybrid Scaffold Containing Synovial Mesenchymal Stem Cells for Cartilage Regeneration Based on Tetrahedral Framework Nucleic Acid Recruitment. Biomaterials 278, 121131. doi:10.1016/j.biomaterials.2021.121131
Li, Q., Lei, X., Wang, X., Cai, Z., Lyu, P., and Zhang, G. (2019). Hydroxyapatite/Collagen Three-Dimensional Printed Scaffolds and Their Osteogenic Effects on Human Bone Marrow-Derived Mesenchymal Stem Cells. Tissue Eng. Part A 25, 1261–1271. doi:10.1089/ten.tea.2018.0201
Li, X., Liu, B., Pei, B., Chen, J., Zhou, D., Peng, J., et al. (2020). Inkjet Bioprinting of Biomaterials. Chem. Rev. 120, 10793–10833. doi:10.1021/acs.chemrev.0c00008
Ligon, S. C., Liska, R., Stampfl, J., Gurr, M., and Mülhaupt, R. (2017). Polymers for 3D Printing and Customized Additive Manufacturing. Chem. Rev. 117, 10212–10290. doi:10.1021/acs.chemrev.7b00074
Lin, J., Zhou, Z., Guan, J., Zhu, Y., Liu, Y., Yang, Z., et al. (2018). Using Three-Dimensional Printing to Create Individualized Cranial Nerve Models for Skull Base Tumor Surgery. World Neurosurg. 120, e142–e152. doi:10.1016/j.wneu.2018.07.236
Liu, S., Sun, L., Zhang, H., Hu, Q., Wang, Y., and Ramalingam, M. (2021). High-Resolution Combinatorial 3D Printing of Gelatin-Based Biomimetic Triple-Layered Conduits for Nerve Tissue Engineering. Int. J. Biol. Macromol. 166, 1280–1291. doi:10.1016/j.ijbiomac.2020.11.010
Luo, Y., Lin, X., and Huang, P. (2018). 3D Bioprinting of Artificial Tissues: Construction of Biomimetic Microstructures. Macromol. Biosci. 18, e1800034. doi:10.1002/mabi.201800034
Lynn, A. K., Yannas, I. V., and Bonfield, W. (2004). Antigenicity and Immunogenicity of Collagen. J. Biomed. Mat. Res. 71B, 343–354. doi:10.1002/jbm.b.30096
Moor, L. D., Fernandez, S., Vercruysse, C., Tytgat, L., Asadian, M., De Geyter, N., et al. (2020). Hybrid Bioprinting of Chondrogenically Induced Human Mesenchymal Stem Cell Spheroids. Front. Bioeng. Biotechnol. 8, 484. doi:10.3389/fbioe.2020.00484
Mandrycky, C., Wang, Z., Kim, K., and Kim, D.-H. (2016). 3D Bioprinting for Engineering Complex Tissues. Biotechnol. Adv. 34, 422–434. doi:10.1016/j.biotechadv.2015.12.011
Mazzocchi, A., Devarasetty, M., Huntwork, R., Soker, S., and Skardal, A. (2018). Optimization of Collagen Type I-Hyaluronan Hybrid Bioink for 3D Bioprinted Liver Microenvironments. Biofabrication 11, 015003. doi:10.1088/1758-5090/aae543
Memarian, P., Sartor, F., Bernardo, E., Elsayed, H., Ercan, B., Delogu, L. G., et al. (2021). Osteogenic Properties of 3D-Printed Silica-Carbon-Calcite Composite Scaffolds: Novel Approach for Personalized Bone Tissue Regeneration. Int. J. Mol. Sci. 22, 475. doi:10.3390/ijms22020475
Min, Z., Shichang, Z., Chen, X., Yufang, Z., and Changqing, Z. (2015). 3D-Printed Dimethyloxallyl Glycine Delivery Scaffolds to Improve Angiogenesis and Osteogenesis. Biomater. Sci. 3, 1236–1244. doi:10.1039/c5bm00132c
Moroni, L., Boland, T., Burdick, J. A., De Maria, C., Derby, B., Forgacs, G., et al. (2018). Biofabrication: A Guide to Technology and Terminology. Trends Biotechnol. 36, 384–402. doi:10.1016/j.tibtech.2017.10.015
Mostafavi, A., Abudula, T., Russell, C. S., Mostafavi, E., Williams, T. J., Salah, N., et al. (2021). In Situ Printing of Scaffolds for Reconstruction of Bone Defects. Acta Biomater. 127, 313–326. doi:10.1016/j.actbio.2021.03.009
Mota, C., Camarero-Espinosa, S., Baker, M. B., Wieringa, P., and Moroni, L. (2020). Bioprinting: From Tissue and Organ Development to In Vitro Models. Chem. Rev. 120, 10547–10607. doi:10.1021/acs.chemrev.9b00789
Mousavi Nejad, Z., Zamanian, A., Saeidifar, M., Vanaei, H. R., and Salar Amoli, M. (2021). 3D Bioprinting of Polycaprolactone-Based Scaffolds for Pulp-Dentin Regeneration: Investigation of Physicochemical and Biological Behavior. Polym. (Basel) 13, 4442. doi:10.3390/polym13244442
Murphy, S. V., and Atala, A. (2014). 3D Bioprinting of Tissues and Organs. Nat. Biotechnol. 32, 773–785. doi:10.1038/nbt.2958
Musioł, M., Sikorska, W., Adamus, G., Janeczek, H., Richert, J., Malinowski, R., et al. (2016). Forensic Engineering of Advanced Polymeric Materials. Part III - Biodegradation of Thermoformed Rigid PLA Packaging under Industrial Composting Conditions. Waste Manag. 52, 69–76. doi:10.1016/j.wasman.2016.04.016
Nam, H., Jeong, H.-J., Jo, Y., Lee, J. Y., Ha, D.-H., Kim, J. H., et al. (2020). Multi-layered Free-form 3D Cell-Printed Tubular Construct with Decellularized Inner and Outer Esophageal Tissue-Derived Bioinks. Sci. Rep. 10, 7255. doi:10.1038/s41598-020-64049-6
Nedunchezian, S., Banerjee, P., Lee, C.-Y., Lee, S.-S., Lin, C.-W., Wu, C.-W., et al. (2021). Generating Adipose Stem Cell-Laden Hyaluronic Acid-Based Scaffolds Using 3D Bioprinting via the Double Crosslinked Strategy for Chondrogenesis. Mater. Sci. Eng. C 124, 112072. doi:10.1016/j.msec.2021.112072
Ng, W. L., Chan, A., Ong, Y. S., and Chua, C. K. (2020). Deep Learning for Fabrication and Maturation of 3D Bioprinted Tissues and Organs. Virtual Phys. Prototyp. 15, 340–358. doi:10.1080/17452759.2020.1771741
Ng, W. L., Chua, C. K., and Shen, Y.-F. (2019). Print Me an Organ! Why We Are Not There yet. Prog. Polym. Sci. 97, 101145. doi:10.1016/j.progpolymsci.2019.101145
Ning, L., and Chen, X. (2017). A Brief Review of Extrusion-Based Tissue Scaffold Bio-Printing. Biotechnol. J. 12, 1600671. doi:10.1002/biot.201600671
Ning, L., Sun, H., Lelong, T., Guilloteau, R., Zhu, N., Schreyer, D. J., et al. (2018). 3D Bioprinting of Scaffolds with Living Schwann Cells for Potential Nerve Tissue Engineering Applications. Biofabrication 10, 035014. doi:10.1088/1758-5090/aacd30
Ning, L., Zhu, N., Smith, A., Rajaram, A., Hou, H., Srinivasan, S., et al. (2021). Noninvasive Three-Dimensional In Situ and In Vivo Characterization of Bioprinted Hydrogel Scaffolds Using the X-Ray Propagation-Based Imaging Technique. ACS Appl. Mat. Interfaces 13, 25611–25623. doi:10.1021/acsami.1c02297
Nulty, J., Freeman, F. E., Browe, D. C., Burdis, R., Ahern, D. P., Pitacco, P., et al. (2021). 3D Bioprinting of Prevascularised Implants for the Repair of Critically-Sized Bone Defects. Acta Biomater. 126, 154–169. doi:10.1016/j.actbio.2021.03.003
Ostrovidov, S., Salehi, S., Costantini, M., Suthiwanich, K., Ebrahimi, M., Sadeghian, R. B., et al. (2019). 3D Bioprinting in Skeletal Muscle Tissue Engineering. Small 15, e1805530. doi:10.1002/smll.201805530
Palmara, G., Frascella, F., Roppolo, I., Chiappone, A., and Chiadò, A. (2021). Functional 3D Printing: Approaches and Bioapplications. Biosens. Bioelectron. 175, 112849. doi:10.1016/j.bios.2020.112849
Paprottka, F. J., Wolf, P., Harder, Y., Kern, Y., Paprottka, P. M., Machens, H. G., et al. (2013). Sensory Recovery Outcome after Digital Nerve Repair in Relation to Different Reconstructive Techniques: Meta-Analysis and Systematic Review. Plast. Surg. Int. 2013, 704589. doi:10.1155/2013/704589
Prendergast, M. E., and Burdick, J. A. (2020). Recent Advances in Enabling Technologies in 3D Printing for Precision Medicine. Adv. Mater 32, e1902516. doi:10.1002/adma.201902516
Qi, C., Liu, J., Jin, Y., Xu, L., Wang, G., Wang, Z., et al. (2018). Photo-Crosslinkable, Injectable Sericin Hydrogel as 3D Biomimetic Extracellular Matrix for Minimally Invasive Repairing Cartilage. Biomaterials 163, 89–104. doi:10.1016/j.biomaterials.2018.02.016
Qu, M., Wang, C., Zhou, X., Libanori, A., Jiang, X., Xu, W., et al. (2021). Multi-Dimensional Printing for Bone Tissue Engineering. Adv. Healthc. Mater 10, e2001986. doi:10.1002/adhm.202001986
Quint, J. P., Mostafavi, A., Endo, Y., Panayi, A., Russell, C. S., Nourmahnad, A., et al. (2021). In Vivo Printing of Nanoenabled Scaffolds for the Treatment of Skeletal Muscle Injuries. Adv. Healthc. Mater 10, e2002152. doi:10.1002/adhm.202002152
Rana, D., Kumar, T. S. S., and Ramalingam, M. (2017). Impact of Nanotechnology on 3D Bioprinting. J. bionanosci 11, 1–6. doi:10.1166/jbns.2017.1417
Rastin, H., Ramezanpour, M., Hassan, K., Mazinani, A., Tung, T. T., Vreugde, S., et al. (2021). 3D Bioprinting of a Cell-Laden Antibacterial Polysaccharide Hydrogel Composite. Carbohydr. Polym. 264, 117989. doi:10.1016/j.carbpol.2021.117989
Rastogi, P., and Kandasubramanian, B. (2019). Review of Alginate-Based Hydrogel Bioprinting for Application in Tissue Engineering. Biofabrication 11, 042001. doi:10.1088/1758-5090/ab331e
Rodríguez-Sánchez, D. N., Pinto, G. B. A., Cartarozzi, L. P., De Oliveira, A. L. R., Bovolato, A. L. C., De Carvalho, M., et al. (2021). 3D-Printed Nerve Guidance Conduits Multi-Functionalized with Canine Multipotent Mesenchymal Stromal Cells Promote Neuroregeneration after Sciatic Nerve Injury in Rats. Stem Cell. Res. Ther. 12, 303. doi:10.1186/s13287-021-02315-8
Sandler, N., and Preis, M. (2016). Printed Drug-Delivery Systems for Improved Patient Treatment. Trends Pharmacol. Sci. 37, 1070–1080. doi:10.1016/j.tips.2016.10.002
Saroia, J., Yanen, W., Wei, Q., Zhang, K., Lu, T., and Zhang, B. (2018). A Review on Biocompatibility Nature of Hydrogels with 3D Printing Techniques, Tissue Engineering Application and its Future Prospective. Bio-des. Manuf. 1, 265–279. doi:10.1007/s42242-018-0029-7
Saunders, R. E., and Derby, B. (2014). Inkjet Printing Biomaterials for Tissue Engineering: Bioprinting. Int. Mater. Rev. 59, 430–448. doi:10.1179/1743280414y.0000000040
Setacci, C., Mele, M., Benevento, D., Ruzzi, U., Giannace, G., De Donato, G., et al. (2018). EVAR Follow-Up: Traumas Could Facilitate Late High Flow Endoleaks. J. Cardiovasc Surg. (Torino) 59, 486–488. doi:10.23736/S0021-9509.18.10133-9
Shafiee, A., Cavalcanti, A. S., Saidy, N. T., Schneidereit, D., Friedrich, O., Ravichandran, A., et al. (2021). Convergence of 3D Printed Biomimetic Wound Dressings and Adult Stem Cell Therapy. Biomaterials 268, 120558. doi:10.1016/j.biomaterials.2020.120558
Sharma, D., Ross, D., Wang, G., Jia, W., Kirkpatrick, S. J., and Zhao, F. (2019). Upgrading Prevascularization in Tissue Engineering: A Review of Strategies for Promoting Highly Organized Microvascular Network Formation. Acta Biomater. 95, 112–130. doi:10.1016/j.actbio.2019.03.016
Shi, Y., Xing, T. L., Zhang, H. B., Yin, R. X., Yang, S. M., Wei, J., et al. (2018). Tyrosinase-Doped Bioink for 3D Bioprinting of Living Skin Constructs. Biomed. Mat. 13, 035008. doi:10.1088/1748-605x/aaa5b6
Sikorska, W., Musioł, M., Rydz, J., Zięba, M., Rychter, P., Lewicka, K., et al. (2018). Prediction Studies of Environment-Friendly Biodegradable Polymeric Packaging Based on PLA. Influence of Specimens' Thickness on the Hydrolytic Degradation Profile. Waste Manag. 78, 938–947. doi:10.1016/j.wasman.2018.07.014
Soman, S. S., and Vijayavenkataraman, S. (2020). Perspectives on 3D Bioprinting of Peripheral Nerve Conduits. Int. J. Mol. Sci. 21, 5792. doi:10.3390/ijms21165792
Steier, A., Schmieg, B., Irtel Von Brenndorff, Y., Meier, M., Nirschl, H., Franzreb, M., et al. (2020). Enzyme Scaffolds with Hierarchically Defined Properties via 3D Jet Writing. Macromol. Biosci. 20, e2000154. doi:10.1002/mabi.202000154
Takeoka, Y., Matsumoto, K., Taniguchi, D., Tsuchiya, T., Machino, R., Moriyama, M., et al. (2019). Regeneration of Esophagus Using a Scaffold-free Biomimetic Structure Created with Bio-Three-Dimensional Printing. PLoS One 14, e0211339. doi:10.1371/journal.pone.0211339
Talikowska, M., Fu, X., and Lisak, G. (2019). Application of Conducting Polymers to Wound Care and Skin Tissue Engineering: A Review. Biosens. Bioelectron. 135, 50–63. doi:10.1016/j.bios.2019.04.001
Tamimi, F., Torres, J., Gbureck, U., Lopez-Cabarcos, E., Bassett, D. C., Alkhraisat, M. H., et al. (2009). Craniofacial Vertical Bone Augmentation: A Comparison between 3D Printed Monolithic Monetite Blocks and Autologous Onlay Grafts in the Rabbit. Biomaterials 30, 6318–6326. doi:10.1016/j.biomaterials.2009.07.049
Utzinger, U., Baggett, B., Weiss, J. A., Hoying, J. B., and Edgar, L. T. (2015). Large-Scale Time Series Microscopy of Neovessel Growth during Angiogenesis. Angiogenesis 18, 219–232. doi:10.1007/s10456-015-9461-x
Valot, L., Martinez, J., Mehdi, A., and Subra, G. (2019). Chemical Insights into Bioinks for 3D Printing. Chem. Soc. Rev. 48, 4049–4086. doi:10.1039/c7cs00718c
van der Elst, L., Faccini De Lima, C., Gokce Kurtoglu, M., Koraganji, V. N., Zheng, M., and Gumennik, A. (2021). 3D Printing in Fiber-Device Technology. Adv. Fiber Mat. 3, 59–75. doi:10.1007/s42765-020-00056-6
Vashistha, R., Kumar, P., Dangi, A. K., Sharma, N., Chhabra, D., and Shukla, P. (2019). Quest for Cardiovascular Interventions: Precise Modeling and 3D Printing of Heart Valves. J. Biol. Eng. 13, 12. doi:10.1186/s13036-018-0132-5
Vig, K., Chaudhari, A., Tripathi, S., Dixit, S., Sahu, R., Pillai, S., et al. (2017). Advances in Skin Regeneration Using Tissue Engineering. Int. J. Mol. Sci. 18, 789. doi:10.3390/ijms18040789
Wu, L., and Ding, J. (2004). In Vitro degradation of Three-Dimensional Porous Poly(d,l-Lactide-Co-Glycolide) Scaffolds for Tissue Engineering. Biomaterials 25, 5821–5830. doi:10.1016/j.biomaterials.2004.01.038
Wu, L., Magaz, A., Wang, T., Liu, C., Darbyshire, A., Loizidou, M., et al. (2018). Stiffness Memory of Indirectly 3D-Printed Elastomer Nanohybrid Regulates Chondrogenesis and Osteogenesis of Human Mesenchymal Stem Cells. Biomaterials 186, 64–79. doi:10.1016/j.biomaterials.2018.09.013
Wu, Z., Xie, S., Kang, Y., Shan, X., Li, Q., and Cai, Z. (2021). Biocompatibility Evaluation of a 3D-Bioprinted Alginate-Gelma-Bacteria Nanocellulose (BNC) Scaffold Laden with Oriented-Growth RSC96 Cells. Mater. Sci. Eng. C 129, 112393. doi:10.1016/j.msec.2021.112393
Xiong, R., Zhang, Z., Chai, W., Chrisey, D. B., and Huang, Y. (2017). Study of Gelatin as an Effective Energy Absorbing Layer for Laser Bioprinting. Biofabrication 9, 024103. doi:10.1088/1758-5090/aa74f2
Yan, W.-C., Davoodi, P., Vijayavenkataraman, S., Tian, Y., Ng, W. C., Fuh, J. Y. H., et al. (2018). 3D Bioprinting of Skin Tissue: From Pre-processing to Final Product Evaluation. Adv. Drug Deliv. Rev. 132, 270–295. doi:10.1016/j.addr.2018.07.016
Yang, Y., Zhang, Q., Xu, T., Zhang, H., Zhang, M., Lu, L., et al. (2020). Photocrosslinkable Nanocomposite Ink for Printing Strong, Biodegradable and Bioactive Bone Graft. Biomaterials 263, 120378. doi:10.1016/j.biomaterials.2020.120378
Yeo, G. C. (2019). A New Vascular Engineering Strategy Using 3D Printed Ice. Trends Biotechnol. 37, 451–453. doi:10.1016/j.tibtech.2019.01.007
Yoo, J., Park, J. H., Kwon, Y. W., Chung, J. J., Choi, I. C., Nam, J. J., et al. (2020). Augmented Peripheral Nerve Regeneration through Elastic Nerve Guidance Conduits Prepared Using a Porous PLCL Membrane with a 3D Printed Collagen Hydrogel. Biomater. Sci. 8, 6261–6271. doi:10.1039/d0bm00847h
Yu, C., Schimelman, J., Wang, P., Miller, K. L., Ma, X., You, S., et al. (2020). Photopolymerizable Biomaterials and Light-Based 3D Printing Strategies for Biomedical Applications. Chem. Rev. 120, 10695–10743. doi:10.1021/acs.chemrev.9b00810
Yue, K., Trujillo-De Santiago, G., Alvarez, M. M., Tamayol, A., Annabi, N., and Khademhosseini, A. (2015). Synthesis, Properties, and Biomedical Applications of Gelatin Methacryloyl (Gelma) Hydrogels. Biomaterials 73, 254–271. doi:10.1016/j.biomaterials.2015.08.045
Zhang, J., Wehrle, E., Adamek, P., Paul, G. R., Qin, X.-H., Rubert, M., et al. (2020). Optimization of Mechanical Stiffness and Cell Density of 3D Bioprinted Cell-Laden Scaffolds Improves Extracellular Matrix Mineralization and Cellular Organization for Bone Tissue Engineering. Acta Biomater. 114, 307–322. doi:10.1016/j.actbio.2020.07.016
Zhang, K., Fu, Q., Yoo, J., Chen, X., Chandra, P., Mo, X., et al. (2017). 3D Bioprinting of Urethra with PCL/PLCL Blend and Dual Autologous Cells in Fibrin Hydrogel: An In Vitro Evaluation of Biomimetic Mechanical Property and Cell Growth Environment. Acta Biomater. 50, 154–164. doi:10.1016/j.actbio.2016.12.008
Zhao, J., and Cui, W. (2020). Functional Electrospun Fibers for Local Therapy of Cancer. Adv. Fiber Mat. 2, 229–245. doi:10.1007/s42765-020-00053-9
Zhou, F., Hong, Y., Liang, R., Zhang, X., Liao, Y., Jiang, D., et al. (2020). Rapid Printing of Bio-Inspired 3D Tissue Constructs for Skin Regeneration. Biomaterials 258, 120287. doi:10.1016/j.biomaterials.2020.120287
Keywords: 3D bioprinting, bioinks, scaffolds, biological materials, tissue repair and regeneration
Citation: Liu N, Zhang X, Guo Q, Wu T and Wang Y (2022) 3D Bioprinted Scaffolds for Tissue Repair and Regeneration. Front. Mater. 9:925321. doi: 10.3389/fmats.2022.925321
Received: 21 April 2022; Accepted: 17 June 2022;
Published: 07 July 2022.
Edited by:
May Griffith, Université de Montréal, CanadaReviewed by:
Hongxu Lu, University of Technology Sydney, AustraliaLaura Zorzetto, Max Planck Institute of Colloids and Interfaces, Germany
Wei Long Ng, Nanyang Technological University, Singapore
Copyright © 2022 Liu, Zhang, Guo, Wu and Wang. This is an open-access article distributed under the terms of the Creative Commons Attribution License (CC BY). The use, distribution or reproduction in other forums is permitted, provided the original author(s) and the copyright owner(s) are credited and that the original publication in this journal is cited, in accordance with accepted academic practice. No use, distribution or reproduction is permitted which does not comply with these terms.
*Correspondence: Yuanfei Wang, emhpenVuYmFvMTlAMTYzLmNvbQ==
†These authors have contributed equally to this work