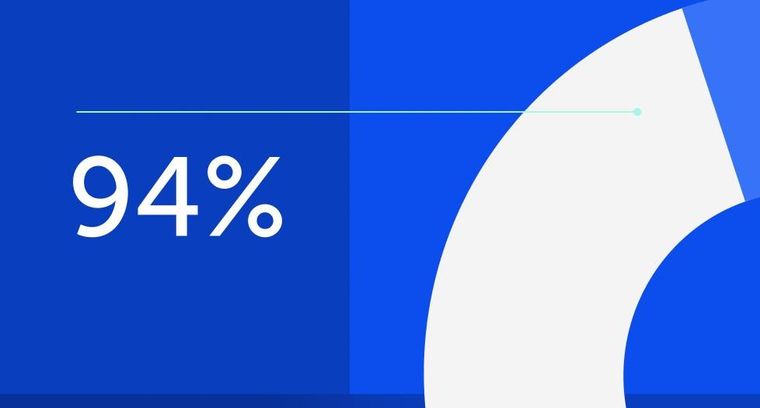
94% of researchers rate our articles as excellent or good
Learn more about the work of our research integrity team to safeguard the quality of each article we publish.
Find out more
ORIGINAL RESEARCH article
Front. Mater., 12 August 2022
Sec. Polymeric and Composite Materials
Volume 9 - 2022 | https://doi.org/10.3389/fmats.2022.912006
To improve the mechanical properties of proton exchange membranes, consequently improving the performance of direct borohydride fuel cells, the present study prepared sulfonated chitosan-g-sulfonated polyvinyl alcohol/polyethylene oxide doped with sulfated zirconia composite (SCS-g-SPVA/PEO/SZrO2) polyelectrolyte membranes. Two fabrication techniques were followed, solution casting and electrospinning, to have the membranes in film and fiber forms and study the effect of the different forms on the membrane’s physicochemical properties. For the casting technique, different concentrations of SZrO2 (1-3 wt%) were used, while the optimum concentration of SZrO2 (3 wt%) was used in the electrospun one (SCS-g-SPVA/PEO/SZrO2-CF). SCS-g-SPVA/PEO/SZrO2-C membranes were prepared in a single step. The grafting and the crosslinking were carried out using glutaraldehyde and sulfosuccinic acid as sulfonating agents for chitosan and PVA and coupling agents simultaneously using click chemistry. On the other hand, SCS-g-SPVA/PEO/SZrO2-CF membranes were prepared in two steps. They were fabricated with electrospinning and then dipped into the coupling and crosslinking solutions. The casting membranes’ physicochemical properties were improved by increasing the SZrO2 content. The experimental results further show that the fabrication procedure significantly influences the physicochemical properties of the membranes. For instance, the composite fiber membrane demonstrated higher selectivity and higher ion exchange capacity (IEC) than the casting membrane. Furthermore, by using the response surface methodology model, the effects of ion exchange capacity, water uptake, and oxidative stability were optimized as three independent variables that affected the ionic conductivity of SCS-g-SPVA/PEO/SZrO2-3C. The optimized ionic conductivity of the SCS-g-SPVA/PEO/SZrO2-3C membrane was 13.6 mS cm−1, achieved at the maximum point of the polynomial model, with an IEC of 0.74 meq g−1, ∼92% water uptake, and about 93% oxidative stability.
A fuel cell is considered one of the most favorable energy resources (Gouda et al., 2020a; Mohy Eldin et al., 2020; Gouda et al., 2021a). It collects the merits of batteries and heat engines and simultaneously avoids their disadvantages. Zero pollution and high efficiency became achievable by a fuel cell, where chemical energy is directly converted to electricity (Gouda et al., 2020b; Gouda et al., 2021b; Gouda et al., 2021c). A fuel cell can provide uninterrupted power as long as reactants are fed to the cell, with both fuel and oxidant being stored externally.
A direct borohydride fuel cell (DBFC) is an electrochemical device that generates electrical energy by the electroreduction of hydrogen peroxide (as the oxidant) at the cathode and the electrooxidation of borohydride (as the fuel) at the anode. Compared with direct alcohol fuel cells or conventional hydrogen-fed polymer electrolyte fuel cells, DBFCs have suitable energy and thermodynamic characteristics (Gouda et al., 2019a; Gouda et al., 2020c; Gouda et al., 2020d).
Proton-exchange membranes (PEM), from which Nafion® is the current benchmark membrane, are an essential component of fuel cells. They work as electron insulators and prevent fuel crossover between electrodes (Gouda et al., 2019b). However, fuel permeability is still an important issue due to the osmotic drag and diffusion, which lower the power density output. Several studies have focused on the transfer of ions or protons via polymeric membranes and new ideas for enhancing its qualities. Still, the high cost of the Nafion® and its fuel permeability has not been solved yet. Therefore, to increase the possibility of DBFCs commercialization, it would be necessary to replace Nafion® membranes with alternative cheap and green membranes. For this purpose, poly (vinyl alcohol) (PVA) membranes could be an excellent choice.
PVA is a synthetic polymer, cheap, chemically stable, and nontoxic (Gouda et al., 2021d; Gouda et al., 2021e; Gouda et al., 2021f; Gouda et al., 2021g). In acidic media, the hydroxyl groups of PVA and chitosan (CS) bond with aldehyde groups of glutaraldehyde to form hemiacetal or acetal linkages (Gouda et al., 2021f). Moreover, PVA and CS functional groups (-OH) react with carboxylic groups of sulfosuccinic acid by esterification reaction to crosslink and sulfonate PVA and CS simultaneously. Resultant crosslinked sulfonated PVA (SPVA) and sulfonated CS (SCS) membranes are water-insoluble and suitable for proton conduction and electronic insulation in fuel cells. Blending and crosslinking a synthetic polymer such as PVA with a natural polymer such as CS has a positive effect on enhancing ionic conductivity (Yang et al., 2018). CS is a cationic polysaccharide, nontoxic, inexpensive, natural, and biodegradable polymer (Yang et al., 2004; Divya and Jisha, 2018). It is insoluble in most organic solvents and water. However, it is soluble in some weak dilute organic acids, like acetic acid.
Polyethylene oxide (PEO) is broadly used as a film-forming synthetic polymer with flexibility, good tensile strength, and high PVA compatibility. It can form a hydrogen bond network with the CS and PVA matrix (Rochliadi et al., 2015; Deshmukh et al., 2016). When ceramic inorganic acid like sulfated zirconia (SZrO2) is calcined at 300°C, it enhances ionic conductivity (14.5 mS cm−1), with an ion-exchange capacity of 0.54 meq g−1 and higher water uptake due to sulfate ions, the SZrO2 addition increases the sulfate ions content inside the polymeric membrane (Saccà et al., 2006; Navarra et al., 2008; Tominaka et al., 2010; Giffin et al., 2012). Also, the addition of SZrO2 to the membrane leads to additional ions within the PVA matrix (Gouda et al., 2021a). Gouda et al. (2021f) reported that a PVA blend membrane containing nano SZrO2 exhibited lower fuel permeability, lower swelling, and improved mechanical properties.
Nanofibers with average diameters <1,000 nm are nanomaterials used in the industrial and academic research (Yang et al., 2018). Electrospinning (Ballengee and Pintauro, 2011; Ballengee et al., 2013; Ballengee and Pintauro, 2013; Zizhou et al., 2021) and bubble electrospinning (Liu et al., 2021; He et al., 2022; Qian and He, 2022) are practical and cost-effective approaches for preparing different types of nanofibers. The conductivity improvement in electrospun nanofiber membranes (Box and Behnken, 1960; Dong et al., 2010; Lin et al., 2010; Yao et al., 2011a; Yao et al., 2011b; Li et al., 2014) has been reported. Compared to conventional polymeric membranes, nanofiber membranes appeared to have distinct anion and proton conductive properties. PVA/CS membranes fabricated by casting were compared with those fabricated by electrospinning techniques, and the performance of the two membranes was very close (Yang et al., 2018).
To contribute to the dissemination and future commercialization of borohydride fuel cells, we are developing low-cost and green-prepared membranes with better oxidative stability, tensile strength, and lower borohydride permeability than Nafion. Herein two types of SCS-g-SPVA/PEO/SZrO2 proton exchange membranes have been fabricated. The first one, SCS-g-SPVA/PEO/SZrO2-C, was prepared by casting method, by crosslinking with sulfosuccinic acid and glutaraldehyde, and then doped with different concentrations of SZrO2 (1-3 wt%). The second was fabricated using the electrospinning technique, leading to composite electrospun nanofiber membranes, SCS-g-SPVA/PEO/SZrO2-CF. The characterization of these two membranes was studied with TGA, DSC, and FTIR. Also, water uptake, fuel permeability, ionic conductivity, and selectivity were measured. The aim of blending the three green polymers is to create a network of hydrogen bonds that promotes proton transport across the membrane. Additionally, combining the three polymers leads to a functionalized nanomaterial that enhances the membrane’s mechanical properties.
The fabrication steps of SZrO2 were detailed in our previous studies (Gouda et al., 2021a; Gouda et al., 2021f). The membranes preparation was as follows: 10 wt% PVA solution was prepared by dissolving PVA (99% hydrolysis, medium MW, Sigma-Aldrich, United States) in water at 90°C for 2 h with vigorous stirring to obtain a clear solution. The chitosan clear solution was obtained by stirring 2 wt% CS (Sigma-Aldrich, United States) in 50 ml water with adding 1 ml acetic acid solution. The PEO solution was prepared by dissolving 2 wt% of PEO (average MW:900,000 g mol−1, Acros Organics, United States) in 50 ml of water: ethanol (50:50 vol%) mixture at room temperature. CS and PEO were then added to the PVA solution to form a blend of the PVA/CS/PEO solution by stirring for 2 h. The wt% in the PVA:CS:PEO blend is ca. 70:15:15.
For casting membrane preparation, the polymeric blend was crosslinked and sulfonated by adding 10 ml of sulfosuccinic acid (99.9 wt% in H2O, Sigma-Aldrich, United States) and 0.5 ml of glutaraldehyde (GA) (50 wt% in H2O, Alfa Aesar, United States) in 10 ml of acetone and thorough stirring for 4 h at 40°C, followed by adding different concentrations of SZrO2 (1–3 wt% with respect to PVA) in the form of a suspension in few drops of water. The membranes formed were named SCS-g-SPVA/PEO/SZrO2-1C, SCS-g-SPVA/PEO/SZrO2-2C, and SCS-g-SPVA/PEO/SZrO2-3C, relative to the SZrO2 wt%. The mixture solution was poured into Petri dishes and dried overnight at 70°C in a vacuum oven.
The preparation of SCS-g-SPVA/PEO/SZrO2-CF composite nanofiber membranes involved the following steps: 1) the SCS-g-SPVA/PEO/SZrO2-F electrospun nanofiber was first prepared with 3 wt% of SZrO2, then 2) SCS-g-SPVA/PEO/SZrO2-F was crosslinked by the same crosslinkers of glutaraldehyde and sulfosuccinic acid, finally 3) SCS-g-SPVA/PEO/SZrO2-F was dipped into the crosslinked SCS-g-SPVA/PEO/SZrO2-3 solution.
For comparison purposes, the SCS-g-SPVA/PEO/SZrO2-3C membrane was fabricated in nanofiber shape because this casting membrane realized the best physicochemical properties in terms of fuel permeability, water uptake, and ionic conductivity. The SCS-g-SPVA//PEO/SZrO2-3C solution (without crosslinkers) was injected in a 10 ml syringe, and the voltage applied was 20 kV. The distance between the syringe needle and the fixed collector was 15 cm. To fill the macro-voids between fibers and crosslink the nanofiber membrane (to enhance mechanical strength), the prepared SCS-g-SPVA/PEO/SZrO2-3F nanofiber membrane was dipped in the crosslinked SCS-g-SPVA/PEO/SZrO2-3C solution for a few minutes, then dried overnight at 70°C in a vacuum oven to obtain SPVA/PEO/SZrO2-3CF, as shown in Figure 1.
The prepared membranes’ thermal properties and functional groups were evaluated with TGA (Shimadzu TGA-50, Japan) and FTIR (Shimadzu FTIR-8400 S- Japan). The morphologies of electrospun SCS-g-SPVA/PEO/SZrO2-3F membrane and SCS-g-SPVA/PEO/SZrO2-3C were observed with SEM (JEOL Jsm 6360LA-Japan). ImageJ software evaluated the diameter distribution of the SCS-g-SPVA/PEO/SZrO2-3F electrospun nanofibers. The physicochemical and electrochemical tests measured tensile strength, water uptake, ion exchange capacity (IEC), swelling ratio, borohydride permeability, oxidative stability, ionic conductivity, and selectivity. The procedures and devices are detailed in our previous study (Gouda et al., 2019b; Gouda et al., 2020d) and in the Supplementary Material.
Design-Expert, 13.0.9.0 program from STAT-EASE, INC was used for the experimental design, model construction, and data analysis. The Box Behnken design (Elessawy et al., 2020) with three variables were used to determine the response pattern and create a model. Because distinct variables are typically expressed in different units and have varying limitations, the significance of their impacts on response can only be compared once they have been coded. In this study, the effects of three independent variables, A (ion exchange capacity), B (water uptake), and C (oxidative stability), at three levels were chosen as three independent variables that affected the ionic conductivity of SCS-g-SPVA/PEO/SZrO2-3C (Y). The range and values of these three independent variables, presented in Table 1, were based on data from preliminary experiments. Furthermore, the developed polynomial models were statistically validated using analysis of variance (ANOVA), with the F-test used to check their statistical significance. The coefficient of determination R2 was used to check their fitting quality (Ming Yang and Chih Chiu, 2012).
TABLE 1. Level of various independent variables at coded values of response surface methodology experimental design.
As shown in Figure 2A, three stages of weight loss appeared for PVA-based composites with TGA (Gouda et al., 2021f). The first is due to moisture evaporation around (50–150°C), while the second concerns the functional groups’ degradation (ca. 150–250°C), and the last stage is due to polymers backbone degradation (250–450°C). It can also be noted that the thermal degradation temperature shifted to higher temperatures as the SZrO2 content increased. That means the thermal stability is improved in SPVA/PEO/SZrO2-3C and SPVA/PEO/SZrO2-3CF membranes due to the presence of sulfated zirconia with optimum content, which supports the membranes by increasing the hydrogen bond network. As illustrated in Table 2, the melting point of the membranes decreased with the increasing SZrO2 content. The decrease in the melting point of membranes is due to hydrogen bonds formed between sulfated zirconia and polymers’ functional groups, which reduces the crystallinity of the membranes (Gouda et al., 2021f; Gouda et al., 2021g). All functional groups in the membrane matrix can be confirmed from the FTIR spectrum, as shown in Figure 2B. The broad peaks at 3,250–3,500 cm−1 refer to–OH groups of PVA and CS, and those at about 1,415 and 1,085 cm−1 are attributed to–C-O groups (Yang and Su, 2011). The peak at 1,580 cm−1 is due to the NH2 group in pure chitosan (Osman and Arof, 2003; Mauricio-Sánchez et al., 2018). The peaks between 900 and 1,200 cm−1 refer to the sulfate group in the doping agent and crosslinker, while the Zr-O bond was found at 470 cm−1.
TEM micrographs of SZrO2 demonstrate that the sulfated zirconia was fabricated in an irregular shape with a nanosize scale (Figure 3A). The surface of the casting membrane, SCS-g-SPVA/PEO/SZrO2-3C, appeared without agglomeration or pores (Figure 3B). In contrast, SCS-g-SPVA/PEO/SZrO2-3F membranes appeared in the fiber shape (Figure 3C). The average diameter distribution of SPVA/PEO/SZrO2-3F nanofibers is presented in Figure 3D, with the diameters ranging between 20 and 280 nm.
FIGURE 3. TEM images of (A) SZrO2, the SEM image of (B) SCS-g-SPVA/PEO/SZrO2-3C and (C) SCS-g-SPVA/PEO/SZrO2-3F, and (D) average diameter distribution of SPVA/PEO/SZrO2-3F.
It can be noted from Figure 4 and Supplementary Table S6 in Supplementary Materials that all the fabricated membranes have hydrophilic nature because all contact angles were <90° (Gouda et al., 2020d). In addition, the thickness of membranes increased with the doping agent content. Also, the water uptake and the swelling ratio dramatically decreased with increasing SZrO2 because it filled all membrane voids and converted the membranes into compact and denser structures (Gouda et al., 2021f). Fenton’s reagent test evaluated the oxidative stability of the membranes. The SCS-g-SPVA/PEO membrane appeared to have the lowest oxidative stability, with the addition of SZrO2 enhancing the resistance of the membrane to radical attack. SCS-g-SPVA/PEO/SZrO2-3C and SCS-g-SPVA/PEO/SZrO2-3CF membranes appeared the complete stability nearly intact (RW 99 and 98.5%), which demonstrates that SZrO2 increases the chemical stability of the polymeric structure (Gouda et al., 2021a). The tensile strength increased with increasing SZrO2 due to increasing the bonds in the matrix, such as the hydrogen bonds (Gouda et al., 2021a; Gouda et al., 2021f). SCS-g-SPVA/PEO/SZrO2-3C and SCS-g-SPVA/PEO/SZrO2-3CF membranes presented a tensile strength of 27 and 26.5 MPa, respectively, which is higher than that of Nafion®117 (25 MPa).
In Figure 5 and Supplementary Table S7 of Supplementary Material, it can be noted that increasing SZrO2 concentration as a doping agent in the membranes not only decreases the borohydride permeability but also increases the ionic conductivity and selectivity. SCS-g-SPVA/PEO/SZrO2-3C and SCS-g-SPVA/PEO/SZrO2-3CF achieved a BH4− permeability of 3.8 × 10–7 and 2.3 × 10–7 cm2 s−1, respectively, which were lower than Nafion®117 (4 × 10–7 cm2 s−1). That may owe to SZrO2 nanoparticles acting as blocks to the migration of borohydride ions through the membrane (Shaari et al., 2018). Furthermore, when SZrO2 is doped into the polymer matrix, hydrogen bonds are formed between the polymer chains’ oxygenated and OH− groups and SZrO2. Moreover, the membrane matrix is compressed and strengthened due to the increased number of proton delivery sites provided by the hydrogen bonds, which are present in the sulfate radicals from the nanocomposite’s structure. This limits excessive water absorption and swelling and improves the membrane ion-conducting properties (Shaari et al., 2018; Gouda et al., 2021f). SCS-g-SPVA/PEO/SZrO2-3C and SCS-g-SPVA/PEO/SZrO2-3CF attained the same ionic conductivity (16 mS cm−1). On the other hand, SCS-g-SPVA/PEO/SZrO2-3CF achieved the best selectivity (0.69 × 105 S cm−3 s), which means it can be a potential candidate for DBFCs. In addition, it is substantially less expensive than Nafion, which costs around 90 $ m−2, while SCS-g-SPVA/PEO/SZrO2-3CF cost is about 40 $ m−2.
FIGURE 5. Ion exchange capacity, ionic conductivity, borohydride permeability, and selectivity of the prepared membranes and Nafion®117.
In this study, we conduct an optimization step to ensure the optimum effect of the IEC, water uptake, and oxidative stability on the ionic conductivity of the SCS-g-SPVA/PEO/SZrO2-3C membrane, which in turn increases the overall DBFC performance. To the best of our knowledge, only a few publications have addressed the optimization of PEMs in fuel cells using RSM. For instance, Shaari and Kamarudin studied the optimal content of SGO and glycerol as an additive on the selectivity of sodium alginate-based biomembrane (Shaari and Kamarudin, 2018). The actual design of this study is presented in Table 3. 3D and 2D contour plots, as shown in Figure 6, illustrate the interactive effects of the three independent variables most affecting the ionic conductivity of the SCS-g-SPVA/PEO/SZrO2-3C membrane. The response variable was fitted with a quadratic equation that describes the process:
TABLE 3. The Box-Behnken design matrix and results for the three variables that influence the ionic conductivity of the SCS-g-SPVA/PEO/SZrO2-3C membrane.
FIGURE 6. (A,C,E) 3D surface plots and (B,D,F) 2D surface plots of the interactive effects of IEC, oxidative stability, and water uptake on the ionic conductivity of the SCS-g-SPVA/PEO/SZrO2-3C membrane.
Y (ionic conductivity) = 13.86 + 1.18A −1.41B+ 0.1875C −0.2AB+ 0.1AC −0.125BC −0.0925A2 −0.3175B2 + 0.4325C2
The ANOVA analysis of variance is well-known for determining the statistical significance of the quadratic response surface model. As shown in Table 4, the quadratic model is suitable for attaining a high coefficient of determination R2 (0.9586). The F-value of 18.03 implies that the model is significant (Shaari and Kamarudin, 2018; Elessawy et al., 2022). There is only a 0.05% chance that an F-value this large could occur due to noise. p-values less than 0.0500 indicate model terms are significant. There is only a 0.62% chance that a Lack of Fit F-value this large could occur due to noise. A deq precision measures the signal-to-noise ratio. A ratio greater than 4 is desirable. The ratio of 16.035 indicates an adequate signal. This model can be used to navigate the design space. The optimal levels of the three components at the maximum point of the polynomial model were 0.74 meq g−1 for IEC, ∼92% water uptake, and about 93% oxidative stability. This leads to about 13.6 mS cm−1 for the optimized ionic conductivity of the SCS-gSPVA/PEO/SZrO2-3C membrane, with good mechanical properties compared to Nafion®117.
This study prepared SCS-g-SPVA/PEO/SZrO2 membranes by casting and electrospinning methods. The electrospun membrane was dipped in crosslinked polymeric casting solution to form a composite nanofiber membrane. By comparing the physicochemical properties of the two types of membranes, it was found that the BH4− permeability of the composite fiber membrane (2.3 × 10–7 cm2 s−1) was lower than the casting one (3.8 × 10–7 cm2 s−1). The ionic conductivity was the same for the two membrane types (16 mS cm−1). Both the composite fiber and the casting membranes achieved lower fuel permeability, higher tensile strength, and better oxidative stability than Nafion®117. The composite fiber membrane demonstrated higher selectivity than the casting membrane, meaning that the fabrication method has a significant effect on the physicochemical properties of the membranes. Using RSM, the SCS-g-SPVA/PEO/SZrO2-3C membrane’s optimal ionic conductivity was 13.6 mS cm−1, which was attained at the polynomial model’s maximum point of 0.74 meq g−1 for IEC, 92% water absorption, and almost 93% oxidative stability. The cost estimation for the prepared membranes is much lower than Nafion, which costs about 90 $ m−2, whereas the cost of the prepared membranes would not exceed 40 $ m−2.
The original contributions presented in the study are included in the article/Supplementary Material; further inquiries can be directed to the corresponding authors.
MHG proposed the project, wrote the manuscript with input from the other coauthors, and produced the membranes. NE performed the experiments, analyzed the data, and wrote the manuscript with input from the other coauthors. ME worked with MG in membrane fabrication. MAG, IR, and AH carried out different characterization tests. MY and DS supervised the work and revised the manuscript.
The authors acknowledge the support from the Academy of Scientific Research and Technology and Bibliotheca Alexandria (ASRT-BA) for this research work through post-doctoral research grant project No. 1436. Fundação para a Ciência e a Tecnologia (FCT, Portugal) is acknowledged for funding a research contract in the scope of programmatic funding UIDP/04540/2020 (DS).
The authors declare that the research was conducted in the absence of any commercial or financial relationships that could be construed as a potential conflict of interest.
All claims expressed in this article are solely those of the authors and do not necessarily represent those of their affiliated organizations, or those of the publisher, the editors, and the reviewers. Any product that may be evaluated in this article, or claim that may be made by its manufacturer, is not guaranteed or endorsed by the publisher.
The Supplementary Material for this article can be found online at: https://www.frontiersin.org/articles/10.3389/fmats.2022.912006/full#supplementary-material
Ballengee, J. B., Haugen, G. M., Hamrock, S. J., and Pintauro, P. N. (2013). Properties and fuel cell performance of a nanofiber composite membrane with 660 equivalent weight perfluorosulfonic acid. J. Electrochem. Soc. 160, F429–F435. doi:10.1149/2.088304jes
Ballengee, J. B., and Pintauro, P. N. (2011). Composite fuel cell membranes from dual-nanofiber electrospun mats. Macromolecules 44, 7307–7314. doi:10.1021/ma201684j
Ballengee, J. B., and Pintauro, P. N. (2013). Preparation of nanofiber composite proton-exchange membranes from dual fiber electrospun mats. J. Membr. Sci. 442, 187–195. doi:10.1016/j.memsci.2013.04.023
Box, G., and Behnken, D. W. (1960). Some new three level designs for the study of quantitative variables. Technometrics 2, 455–475. doi:10.1080/00401706.1960.10489912
Deshmukh, K., Ahamed, M. B., Sadasivuni, K. K., Ponnamma, D., Deshmukh, R. R., Pasha, S. K. K., et al. (2016). Graphene oxide reinforced polyvinyl alcohol/polyethylene glycol blend composites as high-performance dielectric material. J. Polym. Res. 23, 159. doi:10.1007/s10965-016-1056-8
Divya, K., and Jisha, M. S. (2018). Chitosan nanoparticles preparation and applications. Environ. Chem. Lett. 16, 101–112. doi:10.1007/s10311-017-0670-y
Dong, B., Gwee, L., Salas-de la Cruz, D., Winey, K. I., and Elabd, Y. A. (2010). Super proton conductive high-purity Nafion nanofibers. Nano Lett. 10, 3785–3790. doi:10.1021/nl102581w
Elessawy, N. A., Gouda, M. H., Elkady, M. F., Ali, S. M., Gouda, M., Eldin, M. S. M., et al. (2020). Ultra-fast removal of cadmium and lead from wastewater using high-efficient adsorbent derived from plastic waste: Statistical modeling, kinetic and isotherm studies. Dwt 173, 394–408. doi:10.5004/dwt.2020.24809
Elessawy, N. A., Gouda, M. H., Elnouby, M., Taha, N. A., Youssef, M. E., Santos, D. M. F., et al. (2022). Polyvinyl alcohol/polyaniline/carboxylated graphene oxide nanocomposites for coating protection of cast iron in simulated seawater. Polymers 14, 1791. doi:10.3390/polym14091791
Giffin, G. A., Piga, M., Lavina, S., Navarra, M. A., D’Epifanio, A., Scrosati, B., et al. (2012). Characterization of sulfated-zirconia/Nafion composite membranes for proton exchange membrane fuel cells. J. Power Sources 198, 66–75. doi:10.1016/j.jpowsour.2011.09.093
Gouda, M. H., Ali, S. M., Othman, S. S., A. Abd Al-Aziz, S. A., Abu-Serie, M., Elsokary, N. A., et al. (2021e). Novel scaffold based graphene oxide doped electrospun iota carrageenan/polyvinyl alcohol for wound healing and pathogen reduction: In-vitro and in-vivo study. Sci. Rep. 11, 20456. doi:10.1038/s41598-021-00069-0
Gouda, M. H., Elessawy, N. A., Al-Hussain, S. A., and Toghan, A. (2021f). Design of promising green cation-exchange-membranes-based sulfonated PVA and doped with nano sulfated zirconia for direct borohydride fuel cells. Polymers 13, 4205. doi:10.3390/polym13234205
Gouda, M. H., Elessawy, N. A., and Santos, D. M. F. (2020c). Synthesis and characterization of novel green hybrid nanocomposites for application as proton exchange membranes in direct borohydride fuel cells. Energies 13, 1180. doi:10.3390/en13051180
Gouda, M. H., Elessawy, N. A., and Toghan, A. (2021g). Development of effectively costed and performant novel cation exchange ceramic nanocomposite membrane based sulfonated PVA for direct borohydride fuel cells. J. Industrial Eng. Chem. 100, 212–219. doi:10.1016/j.jiec.2021.05.021
Gouda, M. H., Elessawy, N. A., and Toghan, A. (2021d). Novel crosslinked sulfonated PVA/PEO doped with phosphated titanium oxide nanotubes as effective green cation exchange membrane for direct borohydride fuel cells. Polymers 13, 2050. doi:10.3390/polym13132050
Gouda, M. H., Elnouby, M., Aziz, A. N., Youssef, M. E., Santos, D. M. F., Elessawy, N. A., et al. (2020b). Green and low-cost membrane electrode assembly for proton exchange membrane fuel cells: Effect of double-layer electrodes and gas diffusion layer. Front. Mat. 6, 337. doi:10.3389/fmats.2019.00337
Gouda, M. H., Gouveia, W., Afonso, M. L., Šljukić, B., El Essawy, N. A., Nassr, A. B. A. A., et al. (2019b). Poly(vinyl alcohol)-based crosslinked ternary polymer blend doped with sulfonated graphene oxide as a sustainable composite membrane for direct borohydride fuel cells. J. Power Sources 432, 92–101. doi:10.1016/j.jpowsour.2019.05.078
Gouda, M. H., Gouveia, W., Elessawy, N. A., Šljukić, B., Nassr, A. B. A. A., Santos, D. M. F., et al. (2020d). Simple design of PVA-based blend doped with SO4(PO4)-functionalised TiO2 as an effective membrane for direct borohydride fuel cells. Int. J. Hydrogen Energy 45, 15226–15238. doi:10.1016/j.ijhydene.2020.04.013
Gouda, M. H., Gouveia, W., Afonso, M. L., Šljukić, B., and Elessawy, N. A. (2019a). “Santos DMF novel ternary polymer blend membranes doped with SO4/PO4-TiO2 for low temperature fuel cells,” in Proceedings of the 5th World Congress on Mechanical, Chemical, and Material Engineering (MCM’19), August 15 – 17, 2019 (Lisbon, Portugal: Paper No. ICCPE 106). doi:10.11159/iccpe19.106
Gouda, M. H., Konsowa, A. H., Farag, H. A., Elessawy, N. A., Tamer, T. M., Eldin, M. S. M., et al. (2021a). Development novel eco-friendly proton exchange membranes doped with nano sulfated zirconia for direct methanol fuel cells. J. Polym. Res. 28, 263. doi:10.1007/s10965-021-02628-5
Gouda, M. H., Konsowa, A. H., Farag, H. A., Elessawy, N. A., Tamer, T. M., Mohy Eldin, M. S., et al. (2020a). Novel nanocomposite membranes based on cross-linked eco-friendly polymers doped with sulfated titania nanotubes for direct methanol fuel cell application. Nanomater. Nanotechnol. 10, 184798042096436. doi:10.1177/1847980420964368
Gouda, M. H., Tamer, T. M., Konsowa, A. H., Farag, H. A., and Mohy Eldin, M. S. (2021b). Organic-inorganic novel green cation exchange membranes for direct methanol fuel cells. Energies 14, 4686. doi:10.3390/en14154686
Gouda, M. H., Tamer, T. M., and Mohy Eldin, M. S. (2021c). A highly selective novel green cation exchange membrane doped with ceramic nanotubes material for direct methanol fuel cells. Energies 14, 5664. doi:10.3390/en14185664
He, J. H., Qian, M. Y., and Li, Y. (2022). The maximal wrinkle angle during the bubble collapse and its application to the bubble electrospinning. Front. Mat. 8, 800567. doi:10.3389/fmats.2021.800567
Li, H. Y., Lee, Y. Y., Lai, J. Y., and Liu, Y. L. (2014). Composite membranes of Nafion and poly(styrene sulfonic acid)-grafted poly(vinylidene fluoride) electrospun nanofiber mats for fuel cells. J. Membr. Sci. 466, 238–245. doi:10.1016/j.memsci.2014.04.057
Lin, H. L., Wang, S. H., Chiu, C. K., Yu, T. L., Chen, L. C., Huang, C. C., et al. (2010). Preparation of Nafion/poly(vinyl alcohol) electro-spun fiber composite membranes for direct methanol fuel cells. J. Membr. Sci. 365, 114–122. doi:10.1016/j.memsci.2010.08.045
Liu, L., Liu, Y. Q., Li, Y. Y., Shen, Y., and He, J. H. (2021). Dropping in electrospinning process: A general strategy for fabrication of microspheres. Therm. Sci. 25, 1295–1303. doi:10.2298/tsci191228025l
Mauricio-Sánchez, R. A., Salazar, R., Luna-Bárcenas, J., and Mendoza-Galván, A. (2018). FTIR spectroscopy studies on the spontaneous neutralization of chitosan acetate films by moisture conditioning. Vib. Spectrosc. 94, 1–6. doi:10.1016/j.vibspec.2017.10.005
Ming Yang, J. M., and Chih Chiu, H. C. (2012). Preparation and characterization of polyvinyl alcohol/chitosan blended membrane for alkaline direct methanol fuel cells. J. Membr. Sci. 419-420, 65–71. doi:10.1016/j.memsci.2012.06.051
Mohy Eldin, M. S., Farag, H. A., Tamer, T. M., Konsowa, A. H., and Gouda, M. H. (2020). Development of novel iota carrageenan-g-polyvinyl alcohol polyelectrolyte membranes for direct methanol fuel cell application. Polym. Bull. 77, 4895–4916. doi:10.1007/s00289-019-02995-6
Navarra, M., Abbati, C., and Scrosati, B. (2008). Properties and fuel cell performance of a Nafion-based, sulfated zirconia-added, composite membrane. J. Power Sources 183, 109–113. doi:10.1016/j.jpowsour.2008.04.033
Osman, Z., and Arof, A. K. (2003). FTIR studies of chitosan acetate based polymer electrolytes. Electrochimica Acta 48, 993–999. doi:10.1016/s0013-4686(02)00812-5
Qian, M. Y., and He, J. H. (2022). Collection of polymer bubble as a nanoscale membrane. Surfaces Interfaces 28, 101665. doi:10.1016/j.surfin.2021.101665
Rochliadi, A., Bundjali, B., and Arcana, I. M. (2015). “Polymer electrolyte membranes prepared by blending of poly(vinyl alcohol)- poly(ethylene oxide) for lithium battery application,” in Proceedings of the joint international conference on electric vehicular technology and industrial, mechanical, electrical and chemical engineering (ICEVT & IMECE), 04-05 November 2015 (Surakarta, Indonesia: IEEE), 370–373. doi:10.1109/ICEVTIMECE.2015.7496690
Saccà, A., Gatto, I., Carbone, A., Pedicini, R., and Passalacqua, E. (2006). ZrO2-Nafion composite membranes for polymer electrolyte fuel cells (PEFCs) at intermediate temperature. J. Power Sources 163, 47–51. doi:10.1016/j.jpowsour.2005.12.062
Shaari, N., Kamarudin, S. K., Basri, S., Shyuan, L. K., Masdar, M. S., Nordin, D., et al. (2018). Enhanced proton conductivity and methanol permeability reduction via sodium alginate electrolyte-sulfonated graphene oxide bio-membrane. Nanoscale Res. Lett. 13, 82. doi:10.1186/s11671-018-2493-6
Shaari, N., and Kamarudin, S. K. (2018). Performance of crosslinked sodium alginate/sulfonated graphene oxide as polymer electrolyte membrane in DMFC application: RSM optimization approach. Int. J. Hydrogen Energy 43, 22986–23003. doi:10.1016/j.ijhydene.2018.10.098
Tominaka, S., Momma, T., Scrosati, B., and Osaka, T. (2010). Sulfated zirconia as a proton conductor for fuel cells: Stability to hydrolysis and influence on catalysts. J. Power Sources 195, 4065–4071. doi:10.1016/j.jpowsour.2010.01.053
Yang, J. M., Fan, C. S., Wang, N. C., and Chang, Y. H. (2018). Evaluation of membrane preparation method on the performance of alkaline polymer electrolyte: Comparison between poly(vinyl alcohol)/chitosan blended membrane and poly(vinyl alcohol)/chitosan electrospun nanofiber composite membranes. Electrochimica Acta 266, 332–340. doi:10.1016/j.electacta.2018.02.043
Yang, J. M., Su, W. Y., Leu, T. L., and Yang, M. C. (2004). Evaluation of chitosan/PVA blended hydrogel membranes. J. Membr. Sci. 236, 39–51. doi:10.1016/j.memsci.2004.02.005
Yang, J. M., and Su, W. Y. (2011). Preparation and characterization of chitosan hydrogel membrane for the permeation of 5-Fluorouracil. Mater. Sci. Eng. C 31, 1002–1009. doi:10.1016/j.msec.2011.02.025
Yao, Y., Guo, B., Ji, L., Jung, K. H., Lin, Z., Alcoutlabi, M., et al. (2011). Highly proton conductive electrolyte membranes: Fiber-induced long-range ionic channels. Electrochem. Commun. 13, 1005–1008. doi:10.1016/j.elecom.2011.06.028
Yao, Y., Ji, L., Lin, Z., Li, Y., Alcoutlabi, M., Hamouda, H., et al. (2011). Sulfonated polystyrene fiber network-induced hybrid proton exchange membranes. ACS Appl. Mat. Interfaces 3, 3732–3737. doi:10.1021/am2009184
Keywords: proton exchange membranes, polyvinyl alcohol, polyethylene oxide, chitosan, electrospun, direct borohydride fuel cell
Citation: Gouda MH, Elessawy NA, Elnouby M, Ghorab MA, Radwan IO, Hashim A, Youssef ME and Santos DMF (2022) Evaluation of sulfonated chitosan-g-sulfonated polyvinyl alcohol/polyethylene oxide/sulfated zirconia composite polyelectrolyte membranes for direct borohydride fuel cells: Solution casting against the electrospun membrane fabrication technique. Front. Mater. 9:912006. doi: 10.3389/fmats.2022.912006
Received: 03 April 2022; Accepted: 06 July 2022;
Published: 12 August 2022.
Edited by:
Mazeyar Parvinzadeh Gashti, PRE Labs Inc., CanadaReviewed by:
Ji-Huan He, Soochow University, ChinaCopyright © 2022 Gouda, Elessawy, Elnouby, Ghorab, Radwan, Hashim, Youssef and Santos. This is an open-access article distributed under the terms of the Creative Commons Attribution License (CC BY). The use, distribution or reproduction in other forums is permitted, provided the original author(s) and the copyright owner(s) are credited and that the original publication in this journal is cited, in accordance with accepted academic practice. No use, distribution or reproduction is permitted which does not comply with these terms.
*Correspondence: Noha A. Elessawy, bm9ueV9lc3Nhd3lAeWFob28uY29t; Diogo M. F. Santos, ZGlvZ29zYW50b3NAdGVjbmljby51bGlzYm9hLnB0
Disclaimer: All claims expressed in this article are solely those of the authors and do not necessarily represent those of their affiliated organizations, or those of the publisher, the editors and the reviewers. Any product that may be evaluated in this article or claim that may be made by its manufacturer is not guaranteed or endorsed by the publisher.
Research integrity at Frontiers
Learn more about the work of our research integrity team to safeguard the quality of each article we publish.