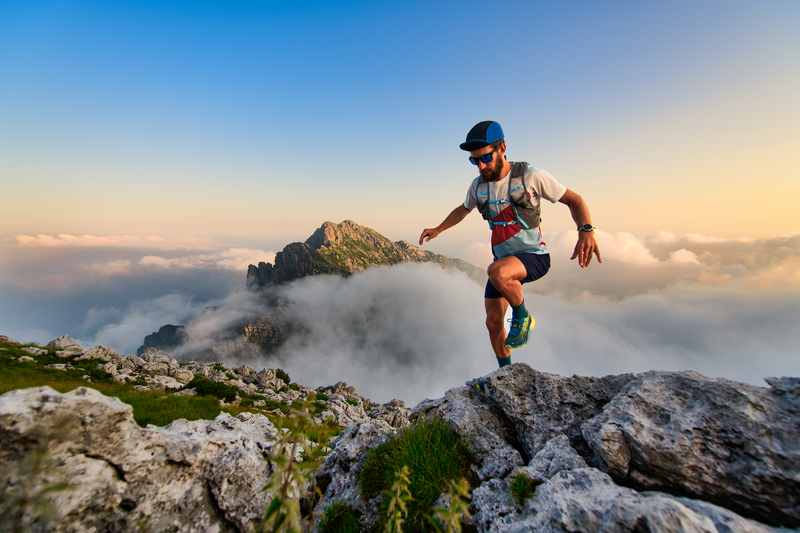
95% of researchers rate our articles as excellent or good
Learn more about the work of our research integrity team to safeguard the quality of each article we publish.
Find out more
REVIEW article
Front. Mater. , 27 June 2022
Sec. Polymeric and Composite Materials
Volume 9 - 2022 | https://doi.org/10.3389/fmats.2022.909534
This article is part of the Research Topic Metal-Organic Framework/Polymer Hybrid Composites: Synthesis Strategies, Characterization, and Applications for Energy and Environment View all 8 articles
Extensive utilization of atrazine (estimated consumption of 70,000–90,000 tons per annum globally) to eliminate undesirable weeds has resulted in the accumulation of atrazine and its metabolites (diaminochlorotriazine, deisopropylatrazine, desethylatrazine, and atrazine mercapturate) in surface and groundwater above maximum permissible limits (drinking water: 3 μg L−1 in the United States, 0.1 μg L−1 in Europe, and 3.0 μg L−1 by the WHO). Atrazine exhibited no to low degradation in aquatic environments; however, poor degradation in soil yields toxic metabolites, which serve as sinks for groundwater resources. Due to mobility, atrazine and its metabolites can persist in various environmental matrices for decades without degradation, posing a serious threat to ecosystem sustainability and, thus, being removed from water resources. Majority of conventional wastewater treatment technologies are either expensive or inefficient. The carbonaceous materials such as activated carbon, biochar, carbon nanotubes, and graphene have been employed as potent adsorbents for the efficient removal of atrazine along with its metabolites from wastewater. Thus, the efficacy of the aforementioned carbonaceous adsorbents for atrazine removal has been discussed in this article by reviewing 161 published articles. The literature survey demonstrated the highest atrazine adsorption capacity of activated carbons (13.95–712.10 mg g−1), followed by biochar (4.55–409.84 mg g−1) and carbon nanotubes (28.21–110.80 mg g−1). Atrazine adsorption onto the carbonaceous adsorbents is a complex process involving single or multiple mechanisms, such as hydrogen bonding, electrostatic interactions, van der Waals forces, hydrophobic interactions, π-π electron donor–acceptor interactions, pore filling, and partitioning. It is recommended that monitoring of atrazine and its metabolites in water resources and their impacts on human and animal lives be explored. Furthermore, modification of carbon-based adsorbents with chemical, mechanical, and thermal means, as well as development of hybrid systems, may completely remove the prevailing atrazine and its metabolites from world water resources.
The intensive utilization of herbicides is contaminating the world's water resources and posing serious health risks for humans along with other lives on the planet Earth. The Europe Union (EU) directive for drinking water permits 0.1 μg L−1–0.5 μg L−1 of a single herbicide (Brown et al., 2004; Chingombe et al., 2006). Atrazine (chemical name: 2-chloro-4-ethylamino-6-isopropylamino-s-triazine) is among the most commonly used herbicides around the globe (Serafin et al., 2022). Atrazine was commercialized in 1959 in the United States and had been widely utilized as a potent herbicide for a long time to control various broadleaf weeds, especially in corn, sorghum, and sugarcane crops (Barr et al., 2007). Shirmardi et al. (2016) reported that atrazine was the most consumable herbicide worldwide with almost 70,000 to 90,000 tons annual consumption. Due to mobility and long-persistency, atrazine has been detected in the fresh, drinking as well as underground water exceeding the maximum permissible limits (drinking water: 3 μg L−1 in the United States, 0.1 μg L−1 in Europe, and 3.0 μg L−1 by WHO), which is deteriorating the health of an ecosystem, including human, animals, plants and marine life (Mahia et al., 2007; Hatfield 2010; Brown et al., 2004). The higher levels of atrazine in water bodies could be the result of 1) volatilization of atrazine into air during spray and introduction into water bodies through precipitation and wind, 2) run-off from surface soil into nearby water resources, 3) leaching from upper soil into groundwater, 4) accidental spill, 5) atmospheric release during manufacturing, and 6) inappropriate disposal of atrazine containing waste (Figure 1) (Pathak and Dikshit, 2011; Dorsey, 2003). It has been estimated that about 14% of atrazine sprayed is volatilized into air and exists as particulate and vapor phases. Likewise, it persists in water bodies via bonding with the sediments. Upon chemical or biological degradation in aquatic systems, it transforms into its metabolites such as diaminochlorotriazine (DACT), deisopropylatrazine (DIA), desethylatrazine (DAE), and atrazine mercapturate (AM). However, in majority of the cases, the atrazine does not degrade and stays on the surface or groundwater for longer periods of time, and consequently enters into the food chain and disturbs the health of the ecosystem including humans and animal lives (Zhang et al., 2021). There are several pathways for the atrazine to enter human beings, such as 1) inhalation, 2) penetration through skin (if contaminated soil or water come in contact), 3) swallowing of atrazine-contaminated food or soil, and 4) drinking atrazine-contaminated water (Figure 1) (Pathak and Dikshit, 2011). Similarly, atrazine can enter into other animals and aquatic lives, subsequently deteriorating their health. The elevated levels of atrazine and its metabolites in water bodies are resulting in various harmful impacts on human beings and animals, such as endocrine disruption, interference with olfactory physiological processes, carcinogenic effects, low sperm count in men, as well as damage to affect human nerves, liver, and heart (Nápoles-Armenta et al., 2021). Various studies demonstrated the acute impacts of atrazine on the central nervous system, reproductive health, immune system, and endocrine system (Bohn et al., 2011). Hence, the exposure of atrazine to human beings and animals must be eliminated by using advanced technologies.
FIGURE 1. The sources and routs for the introduction of atrazine into various environmental matrices, as well as exposure to the human beings.
Atrazine can chemically interfere with plant’s photosynthetic process, which may result in blocking the photosynthesis completely and making food unavailable to plants (Barr et al., 2007). Even though atrazine is not expected to bioaccumulate (from available data), the chemical process could be dangerous to vegetable consumers since atrazine would readily metamorphose to hydroxylated metabolites which is insoluble in water and in consequence, requires a more rigorous method for food safety. Therefore, the commercial use of atrazine has been banned in a lot of countries owing to its harmful impacts on humans, animals, and plants; however, some of the metabolites such as DACT, DIA, DAE, and AM have been detected in the environment suggesting the extent of atrazine toxicity and environmental exposure (Barr et al., 2007). Jablonowski et al. (2009) reported 0.1 μg kg−1–3.4 μg kg−1 of atrazine in soils after 22 years of last atrazine spray, suggesting its long-term persistence. In another study, 19.5 g ha−1 of atrazine was found in agricultural soil even after 20 years of application (Jablonowski et al., 2011). About 1–6% of the applied herbicides are being introduced into aquatic environment from agricultural lands and forests as a result of leaching and runoff. The situation gets worst in groundwater as compared to soil owing to low organic carbon and lack of microbes for atrazine degradation (Vonberg et al., 2014).
Despite the aforementioned environmental concerns, utilization of atrazine for weed control is still in practice in many countries around the world. Thus, atrazine is still considered to be a crucial environmental pollutant with potent carcinogenic impacts and practical management of atrazine-contaminated soil and water still remains a challenge for the environmentalists. The complete removal of atrazine from contaminated media is very difficult to achieve, which requires exploring the advanced removal technologies and removal mechanisms to attain complete removal (Mudhoo and Garg, 2011). Therefore, in order to protect the health of human beings, animals, and aquatic lives, as well as of environment, the atrazine utilization must be stopped in the countries around the globe. Additionally, the prevailing atrazine and its metabolites in soil, sediments, and water bodies must be removed using efficient, cost-effect, and eco-friendly technologies. Therefore, the development of useful and efficient advanced removal techniques is required for the complete removal of atrazine from contaminated media.
Therefore, based on the above-mentioned facts, we have analyzed and discussed the published literature about the efficiency of various carbonaceous adsorbents for the removal of atrazine from contaminated water in this review article. The main objectives of this review were to provide an overview of the carbonaceous materials for efficient and eco-friendly removal of atrazine and to discuss the mechanisms involved in atrazine removal from contaminated water with the help of carbon-based adsorbents. Lists of carbonaceous adsorbents along with their main features and atrazine adsorption capacity have been compiled from the published literature. Moreover, future prospective and research needs for the efficient removal of atrazine from waste media using efficient carbonaceous materials have been provided.
Several techniques have been employed for the removal of atrazine from wastewater streams, including incineration, coagulation, biodegradation, catalytic degradation, ozonation, solvent extraction, steam stripping, advanced oxidation processes, chlorination, filtration, and adsorption (Chang et al., 2005; Clausen and Fabricius, 2001; Ghosh and Philip, 2005; Zhu et al., 2005; Elwakeel et al., 2017). The majority of the conventional wastewater treatment techniques can remove a range of environmental contaminants effectively; however, they are inefficient at removing atrazine entirely, owing to its high stability, lower biodegradation, and hydrophilicity (Chingombe et al., 2006). Even the most advanced wastewater treatment technologies such as ozonation and chlorination have been found ineffective in atrazine removal due to high energy requirements, being expensive, and yielding unwanted by-products (Ahmad et al., 2019). The inefficient and poor removal efficiency of various conventional wastewater treatment techniques results in the generation of degradation by-products/metabolites of atrazine even with higher toxicity than atrazine itself, consequently enhancing the levels of secondary pollutants (Gkementzoglou et al., 2016). A selective, reliable, cost-effective, and eco-friendly method is needed for the efficient removal of atrazine along with its metabolites from various water sources for a sustainable environment. Among the aforementioned wastewater treatment techniques, adsorption is widely accepted and efficient for the removal of recalcitrant organic pollutants such as atrazine. Adsorption is characterized by its low capital and operational cost, simplicity, eco-friendliness, higher efficiency, and insensitivity to toxic substances. However, the selection of an appropriate adsorbent is of critical importance for efficient and complete atrazine removal from wastewater.
Various adsorbents, including clays, nanoparticles, nanometals-based adsorbents, MgO particles, nanoparticles, and carbon-based adsorbents, have been employed as good adsorbents for atrazine removal. However, majority of these adsorbents, such as zeolite and bentonite have lower adsorption capacity for atrazine (He et al., 2019). Therefore, various researchers have synthesized engineered adsorbents such as polymer-nanoparticles, magnetic nanoparticles, mesoporous silica-coated Fe3O4 nanoparticles, and magnetic biochars. The application of such engineered adsorbents has demonstrated higher atrazine removal efficiency with good regeneration efficiency (Gkementzoglou et al., 2016; Li et al., 2018). The application of nano-based adsorbents for atrazine removal has gained a huge deal of attention from researchers in recent years (Shirmardi et al., 2016). However, despite their higher atrazine adsorption capacity, nanomaterial-based adsorbents are expensive and their fabrication is complicated (He et al., 2019). Likewise, porous polymeric adsorbents contain both hydrophilic and hydrophobic interactions and thus possess lower selectivity (He et al., 2019).
Though numerous adsorptive materials have been fabricated and employed for atrazine removal from water, there is still a need to develop novel, low-cost, eco-friendly, and efficient adsorbents for the complete removal of atrazine and its metabolites. In this scenario, carbonaceous adsorbents are considered ideal candidates for efficient wastewater treatments to eliminate atrazine and its metabolites due to their cheaper source, large surface areas, higher porosity, as well as unique structural and surface chemistry (Anjum et al., 2019). Carbonaceous adsorbents are biodegradable, involve waste recycling/reusing, have low/no impacts on the ecosystem, and are cheaper (Kudaybergenov et al., 2010).
Carbonaceous adsorbents have been observed to possess higher stability and lower the costs of raw materials (Abd et al., 2020). Additionally, such adsorbents require lower regeneration energy and temperature, while being easier to regenerate. Regeneration is one of the most prominent features of carbonaceous adsorbents for practical application. The regeneration is done by desorbing the adsorbate from the surface of the adsorbent and making the adsorbent ready for the next cycle of adsorption process. Multiple techniques are available to regenerate the adsorbents, including thermal, solvent extraction, biological, gas purging, acid-base treatment, and hybrid methods (Dutta et al., 2019). In this regard, Mohammadi et al. (2019) stated that carbon-based adsorbents such as activated carbon, biochar, and hydrochar can be regenerated easily for proper end-of-life management. Regeneration can substantially improve the surface properties and lifetime of adsorption of the carbonaceous adsorbents; hence, reducing the cost of pollutant removal process (Álvarez et al., 2004). Therefore, carbonaceous adsorbents are often regarded as suitable, sustainable, and cost-effective means of atrazine removal from contaminated media because such adsorbents involve cheaper raw materials and can easily be regenerated multiple times without producing secondary pollutants.
Among the most commonly used carbonaceous adsorbents, activated carbon and biochar are of significant importance. The application of carbon-based adsorbents has drawn much attention from the environmentalists for the removal of various organic and inorganic pollutants (Bandura et al., 2016). Furthermore, carbonaceous nano-adsorbents such as graphene and carbon nanotubes have recently been introduced as efficient adsorbents for organic pollutants such as atrazine (Zhang et al., 2010; Ersan et al., 2019). The performance of these nano-adsorbents in aqueous media is better than that of conventional activated carbon due to their higher surface area, complex network of the pore, and higher sorption sites (Ersan et al., 2019). Therefore, the performance and efficiency of carbonaceous adsorbents for atrazine removal from contaminated aqueous media are described in the following sections of this article. Moreover, the major mechanisms controlling atrazine adsorption are discussed in detail. A comprehensive overview of atrazine removal from contaminated water through carbon-based adsorbents is provided, and a summary of the maximum adsorption capacities of these adsorbents is given.
Activated carbon (AC) is considered to be an efficient adsorbent owing to its higher surface area and porosity as well as several other unique characteristics (Sharma et al., 2008; Ahmed et al., 2015). On a commercial scale, agricultural waste, wood, coconut shell, lignite, coal, and peat are used for the preparation of ACs (Baccar et al., 2009; Yacob and Al Swaidan, 2012). Its high porosity makes it an efficient adsorbent for a range of environmental contaminants. Usually, chemical and physical activation methods are used for the ACs fabrication. In general, in physical activation, two pyrolysis processes are used, i.e., inactive atmosphere and solid residue artifact at higher temperatures; whereas, in chemical activation, phosphoric acid is used for the production of commercial activated carbon (Budinova et al., 2006; Baccar et al., 2009). Granular activated carbons (GAC) are mostly used for the removal of atrazine from wastewater streams. The porous structure and higher internal surface area of the AC make it very favorable for the adsorption process. Higher surface area and chemical characteristics of ACs enable them to be used in industrial applications, the environment, and water purification, specifically for industrial waste. Activated carbons are used for the removal of toxic pollutants like phenols, organic compounds, and chromium (Gonzalez-Serrano et al., 2004). The previous techniques for the removal of chloro-s-triazines from water showed that the ozonation efficacy varies with the different sources of water. Sedimentation through alum and iron salts, an abundance of lime ash softening, and disinfection by free chlorine were all ineffective techniques, while GACs removed the chloro-s-triazines very efficiently. GACs are considered to be the most effective adsorbents for the removal of atrazine from wastewater; however, the presence of dissolved organic matter may reduce its efficiency (Zadaka et al., 2009). The characteristics of ACs that affect adsorption include pore texture, surface chemistry, and mineral matter content. The adsorption capacity of the ACs is not simply related to their porosity and surface area, but rather it is about the accessibility of organic pollutants to the inner surface of the adsorbent. The surface chemistry of the activated carbon is dependent on the heteroatom content, specifically the oxygen complex content. The surface charge depends on the pH and surface properties of the carbon. Surface oxygen complexes of acidic nature like carboxyl and phenolic groups produce a negative charge on dissociation (Moreno-Castilla, 2004). Therefore, tuning and optimization of these characteristics may further increase the efficiency of the AC for atrazine removal.
Lupul et al. (2015) synthesized surface activated carbon (SAC) using hemp (Cannabis sativa L.) stems, and modified it with N2, NH3, and HNO3. Adsorption results revealed that the highest atrazine (2-chloro-4-ethylamino six isopropylamino-1,3,5-Triazine) adsorption capacity was exhibited by SACs and N2-treated ACs (227 and 263 mg g−1, respectively), while the mechanisms of atrazine removal were identified as intraparticle diffusion with the combination of film diffusion. However, nitrogen and oxygen present on the surface of carbon were of no concern in the adsorption process (Lupul et al., 2015). In another study, Shirmardi et al. (2016) synthesized nanocomposites of AC with MgO and ZnO and employed them for atrazine removal from contaminated water. It was reported that pristine AC demonstrated the highest atrazine adsorption capacity as compared to nanocomposites of AC-MgO-ZnO, and therefore, they concluded ACs as the most suitable adsorbents than MgO, ZnO nanoparticles, and AC-MgO-ZnO nanocomposites. Contrarily, Shao et al. (2017) fabricated powdered AC and its composites with a gravity-driven membrane and reported 28.5% more atrazine removal by using composite material than pristine ACs. Likewise, Jiang et al. (2021) synthesized CoFe2O4 nanoparticles composited onto ACs and successfully used them for atrazine removal. Results revealed that AC-CoFe2O4 composites were the most efficient materials for the removal of atrazine through photo-Fenton degradation and physisorption. Similarly, Agani et al. (2020) fabricated AC-magnetite-chitosan composites and successfully used them for efficient atrazine removal from aqueous media.
The atrazine adsorption efficiencies of different ACs synthesized from various activation techniques in previous studies have been enlisted in Table 1. Alfa-Aesar-derived GACs exhibited the highest atrazine adsorption capacity (712.1 mg g−1) among all the reported ACs in Table 1 (Rambabu et al., 2012). The ACs derived from rayon fiber, hemp stems (with and without N2 activation), bituminous coal, used coal with KOH activation, walnut shell, oil palm shells, and wood exhibited comparatively higher Langmuir isotherm predicted adsorption efficiencies towards atrazine (212.26–476.1 mg g−1) (Faur et al., 2005; Lladó et al., 2015; Lupul et al., 2015; Rachel et al., 2016; Wei et al., 2017; Wei et al., 2018). Likewise, wood, walnut shell, and apricot shell-derived ACs showed higher adsorption capacities as predicted by Dubinin–Radushkevich isotherm (221.78–255.98 mg g−1) (Wei et al., 2018). Whereas the ACs derived from oak (activated with MgO and ZnO nanoparticles), raw waste, and jack fruit peel showed relatively lower atrazine adsorption capacities as predicted by Langmuir isotherm (13.19–22.9 mg g−1) (Wang, 2003; Ghosh and Philip, 2005; Jain et al., 2009).
TABLE 1. Efficiency of various activated carbons for the removal of atrazine from aqueous media as predicted by Langmuir, Freundlich, and Dubinin–Radushkevich isotherms.
It has been established that the adsorption of a solute onto porous adsorbents follows three mechanisms of adsorption, i.e., transport of adsorbate from solution to the outer surface of adsorbent (film diffusion), transport of adsorbate inside the particles of adsorbent (particle diffusion), and sorption onto the interior of the porous adsorbent (Chingombe et al., 2006). Atrazine removal through ACs may follow single or multiple mechanisms depending on the type and properties of the ACs as well as sorption conditions. The major mechanisms reported by various researchers for atrazine removal through ACs include electrostatic interactions, cation exchange, van der Waals forces, hydrophobic effects, H-bonding, pore filling, diffusion, partitioning, and π-π interactions (Kovaios et al., 2006; Welhouse and Bleam, 1993; Kulikova and Perminova, 2002; Tang et al., 2012; Salvestrini et al., 2010; Zhao et al., 2013). Various reports have demonstrated that π-stacking interactions are the major mechanism for atrazine removal due to interactions of aromatic π-systems in ACs and atrazine molecules (Lladó et al., 2015). However, Lupul et al. (2015) reported that electrostatic interactions may not pose any influence on atrazine adsorption in aqueous solution in pH range of 5–9, as atrazine is a weak basic herbicide with a pKa value of 12.3. Davies and Jabeen (2003) stated that H-bonding can serve as a sorption mechanism owing to the fact that atrazine has the capability to form H-bond with carboxyl functional groups. In contrast, Lupul et al. (2015) stated that H-bond was not the dominant mechanism for atrazine adsorption onto hemp stem-based ACs, as they obtained an L-shaped curve rather than an S-type curve. Furthermore, they argued that if atrazine adsorption was due to the H-bonding, then hemp stem-based ACs activated with HNO3 and NH3 (possessing ample amount of oxygen and nitrogen) should manifest the highest adsorption capacity, whereas, the results were opposite as can be seen in Table 1. Further, Welhouse and Bleam (1993) stated that the carboxylic groups as well as amide and pyridinic functional groups on the surface of oxidized and aminated ACs, respectively, could serve as potential sites for H-bonding generation. In contrast, Lupul et al. (2015) observed a significant reduction in atrazine adsorption onto hemp stem-based ACs activated with HNO3 and NH3, which could be due to adsorption of water molecules onto the polar functional groups of ACs via H-bonding, consequently creating a hurdle for the further atrazine molecules to be adsorbed onto the adsorbents. Thus, π–π dispersive interactions between the heterocyclic atrazine ring and aromatic carbon atoms of the ACs were found to be the major mechanism controlling atrazine adsorption onto the aforementioned ACs. Chingombe et al. (2006) have also reported similar results stating that H-bonding was not the major mechanism for atrazine adsorption onto coal-based GACs. Therefore, they concluded that film diffusion was the operating mechanism for adsorbing atrazine onto GACs. Moreover, the polar oxygen and nitrogen functional groups on the surface of GACs have the capability to adsorb water molecules, subsequently reducing the atrazine adsorption due to the hydrophobic nature of atrazine (Chingombe et al., 2006). Moreover, pore size distribution and pore volume have been recognized as important factors for adsorption in carbon-based adsorbents. Mamchenko et al. (1982) reported that pore filling into the micropore was the main type of physical adsorption mechanism for the adsorption of organic pollutants onto ACs.
Biochar is a black, solid, stable, and porous carbonaceous substance obtained by the pyrolysis of different types of biomasses such as manure, wood, grass, manure, sludge, etc. under limited or zero oxygen supply (Sohi, 2012; Ahmad et al., 2018). Due to distinctive features including surface functional groups, porous structure, and large surface area, biochar has attained a range of environmental, engineering, agricultural, and industrial applications. Biochar has been identified as a potential candidate to mitigate climate change through carbon sequestration and improve soil fertility simultaneously. Besides other applications, biochar has widely been used as a low-cost and effective adsorbent for the removal of a range of environmental contaminants, including organic and inorganic substances. Biochar has properties identical to the activated carbon, which is efficiently being used as a sorbent for the removal of pollutants from wastewater treatment plants; however, it requires lower temperatures and energy compared to activated carbon (Chen et al., 2007a; Karakoyun et al., 2011; Lu et al., 2012). Biochar has exhibited excellent removal efficiencies for a range of contaminants from polluted aqueous solutions (Xue et al., 2012; Yang et al., 2014).
Biochar has attained a great deal of attention from researchers as an effective adsorbent for organic pollutants such as atrazine. However, the sorption capacities of biochar for atrazine largely depend on feedstock sources, pyrolysis temperature, rate of pyrolysis, and resident time. Moreover, the type and number of surface functional groups (Penn et al., 2018), surface area, and strong binding affinity may also influence the sorption potential of biochar for atrazine (Zhao et al., 2013; Mandal et al., 2017). Biochar's sorption capacities for atrazine vary largely with the type of feedstock used for biochar production. For instance, Table 2 represents that corn straw-derived biochar composite with NH₄H₂PO₄ showed higher atrazine adsorption capacity compared with other biochars. Additionally, it was reported that the adsorption capacities of biochar residues and colloids increased with increasing pyrolysis temperatures, and high adsorption capacities were recorded in biochar colloids due to high mineral substances and more oxygen functional groups compared to biochar residues. The maximum atrazine adsorption capacity (139.33 mg g−1) was attained in biochar colloids pyrolyzed at 700°C, signifying the important role of pyrolysis temperature in atrazine removal (Yang et al., 2018).
TABLE 2. Efficiency of various biochars for the removal of atrazine from aqueous media as predicted by Langmuir, Freundlich, and Dubinin–Radushkevich isotherms.
Various studies have demonstrated higher atrazine removal efficiencies through biochars as compared to soil organic matter and activated carbon (Table 2) (Deng et al., 2014). However, the time and capacity of biochar for sorption of atrazine may follow different mechanisms depending upon the type and characteristics of the biochar applied. Liu et al. (2015) studied atrazine adsorption characteristics of rice stalks, corn stalks, soybeans, pig manure, poultry manure, and cattle manure-derived biochars and suggested that the highest atrazine sorption occurred at 24 h by soybean-derived biochar, which was reduced subsequently. The decrease in the rate of atrazine adsorption with time could be explained by a gradual decrease in the availability of adsorption sites, resulting in the slow atrazine diffusion into the micro and mesopores in the biochar (Weng et al., 2009; Liu et al., 2015). Zhao et al. (2013) reported that the atrazine sorption capacity of corn straw biochar treated with NH₄H₂PO₄ was significantly higher than the pristine biochar (corn straw).
The physicochemical properties of the biochar, especially surface area, pore size, and composition of the functional groups, play a critical role in atrazine adsorption dynamics (Liu et al., 2015). A higher surface area usually results in higher atrazine adsorption capacities of biochar (Liu et al., 2015). It was reported that lower pH favored atrazine sorption in an aqueous medium no matter what type of biochar was used (Zhao et al., 2013). Lower pH might be an advantageous factor for the sorption of ionizable substances (Oh and Seo, 2016; Mandal et al., 2017), which proposes the role of negative surface charges in the biochar adsorption capacity. In a study by Penn et al. (2018), it was reported that pH did not affect the sorption process and heat release. The atrazine-biochar solution with a neutral pH does not alter the state of atrazine, thus reducing the possibility of cationic exchange in the sorption process (Weber, 1993). However, biochar with a higher pH may result in higher atrazine sorption. For instance, Mandal and Singh (2017) reported that rice straw-derived biochar with a higher pH adsorbed larger amounts of atrazine, compared to similar biochar with a lower pH.
Besides pH, the temperature during the sorption plays a vital role in atrazine adsorption onto biochars. Generally, the atrazine sorption increases as the temperature increases. It was observed that the Langmuir predicted atrazine sorption capacity of pristine and modified corn straw biochars increased two-folds with increasing the temperature from 283.15 to 313.15 K (Table 2) (Zhao et al., 2013). Thus, it was suggested that higher temperature was a beneficial aspect of atrazine sorption. However, the biochars still hold the optimum capability of sorption at low temperatures (Zhao et al., 2013). Likewise, the initial atrazine concentration plays a significant role in the rate of adsorption. Generally, a higher rate of adsorption can be achieved with lower initial atrazine concentrations and vice versa. Similarly, the dose of the biochar substantially influences the rate of atrazine adsorption. Mandal and Singh (2017) reported that the amount of biochar required for atrazine removal increased linearly with increasing removal percent of atrazine needed in all sorption stages.
Contaminant removal mainly depends on the characteristics of the adsorbent and adsorbate, as well as the sorption conditions. Due to the heterogeneous characteristics of various biochars, their atrazine removal process is of complex nature, involving multiple mechanisms depending upon initial adsorbate concentration, solution pH, temperature, and biochar type. It has been reported that the adsorption of atrazine onto biochar may follow H-bonding, hydrophobic interactions, pore diffusion, electrostatic interaction (attraction or repulsion), π-π–electron-donor-acceptor (EDA) interactions, electrophilic interaction, ion complexation, and bonding with the surface functional groups (–OH, R–OH, and–COOH) (Figure 2). For instance, Laird and Koskinen (2008) reported that interactions between organic functional groups of biochar and atrazine were involved in atrazine removal from aqueous solutions. Ma and Selim (1996) reported that covalent bonding, H-bonding, physical fixation, ionic bonding, hydrophobic interactions, and van der Waals forces were responsible for atrazine removal through biochar. Likewise, the H-bonding was responsible for the removal of atrazine through sludge-derived biochar, as reported by Zhang et al. (2015).
The electrostatic interactions have been reported as the dominant mechanism, along with other contributing mechanisms, for the adsorption of pesticides onto the biochars. In this mechanism, anionic adsorbates are attracted to cationic sorption sites, whereas cationic adsorbates are attracted towards the anionic sorption sites (Zheng et al., 2013). The ionic strength and pH are the main factors that can affect electrostatic forces (attractive or repulsive) between organic adsorbates and biochar. At pH higher than pHPZC (pH at point of zero charges) of the biochar, the surface is net negatively charged, while at lower pH, the total surface charge is net positive (Mukherjee et al., 2011). Likewise, at attractive electrostatic interactions, a decrease in atrazine sorption has been noticed with increasing the ionic strength of organic pollutants (Inyang et al., 2014). Moreover, the surface characteristics of biochar play a very crucial role in the adsorption of atrazine via electrostatic interactions. Furthermore, the biochars pyrolyzed at low temperatures possess phenol and carboxyl moieties in their functional groups, which have the ability to bind cationic organic species (Ahmad et al., 2015).
Specific surface areas and active sorption sites present on the surface of biochar significantly affect the adsorbent's efficiency towards atrazine removal in the batch systems (Qu et al., 2013). The surface area plays a crucial role and is directly linked to the adsorption capacity (Han et al., 2013). A higher surface area usually provides more sites for adsorption, consequently resulting in higher atrazine removal through the pore-filling mechanism (Chen et al., 2012). Biochar surface is usually comprised of micro-, meso-, and macropores; however, most of the surface area of biochar is covered with micro-, and mesopores, helping in removing higher amounts of atrazine (Nguyen et al., 2007; Han et al., 2013). The pore-filling process has been seen as the dominant sorption mechanism for atrazine under lower volatile matter content or lower solute concentration (Kasozi et al., 2010). In various studies, an increased adsorption capability of carbonaceous material was reported due to the prominent effect of pore filling in an aqueous medium owing to higher pore volumes and higher surface areas (Han et al., 2013; Zhu et al., 2014). However, slightly higher atrazine adsorption capacities due to pore filling were recorded on biochars compared to other pesticides at low solute concentrations owing to the smaller size of atrazine molecules (Zhang et al., 2013).
The removal of organic compounds through hydrophobic interactions involves both hydrophobic adsorption and partitioning (Murphy et al., 1994). Freshly pyrolyzed biochars are hydrophobic with lower surface oxidation and can adsorb neutral organic compounds as well as hydrophobic organic compounds (HOC) through hydrophobic adsorption and partitioning (Chen et al., 2011). In hydrophobic adsorption, direct competition between water molecules and adsorbed polar molecules occurs on adsorbent (Zhu et al., 2005). Zhang et al. (2013) stated that aromatic carbon in the adsorbent with the hydrophobic surface can offer more adsorption sites for the pore-filling process as well as a hydrophobic effect. Many studies have suggested a positive correlation between aromatic carbon within the adsorbents and the partition coefficient (Ahmed et al., 2001; Yang et al., 2011). The aromatic carbon content of biochar is correlated positively with the carboxyl carbon content and O-alkyl groups due to the higher ash contents. Both functional groups would have decreased the availability of aromatic domains for pesticides. Therefore, many interactions other than hydrophobic partitioning might be involved in pesticide adsorption onto biochar surfaces (Yang et al., 2011; Zhang et al., 2011).
Hydrogen bonding is a conceivable mechanism for the sorption of various organic compounds by the application of biochars (Sun et al., 2011; Chen et al., 2015). The presence of a range of surface functional groups on biochar helps in atrazine removal by developing H-bonds between atrazine (electronegative elements) and biochars (electropositive elements) (Sun et al., 2012). For instance, Wang et al. (2020) stated that atrazine molecules may serve either hydrogen donors or accepters, subsequently developing H-bonds with H, O, or N-containing functional groups of biochar. Similarly, Zhang et al. (2015) reported that the carboxylic and phenolic functional groups of biochar generated H-bonds with azo- or amino-N groups of the atrazine molecule, suggesting H-bonding as the major mechanism controlling atrazine adsorption onto biochar. Zhao et al. (2017) suggested that the release of free H+ ions in the aqueous media may generate H-bonds with the O-containing functional groups on biochar surface, resulting in atrazine adsorption onto biochar.
Strong non-covalent EDA interactions in graphene-like biochar surfaces may act as an operating sorption mechanism for atrazine removal. Complete graphitization of biochar occurs at higher temperatures (>1,100°C); however, an irregular distribution of charges in the aromatic rings of biochars takes place at medium temperatures (>500°C) (Keiluweit et al., 2010; Spokas, 2010). This aromatic π-system, developed in biochars pyrolyzed under low to medium temperature, may act as electron acceptors owing to their electron-removal units (Sun et al., 2012). It can affect electron density by either increasing or decreasing it, consequently generating EDA systems (Zhu and Pignatello, 2005). Electron-rich graphitic carbon sheets or poly-condensed aromatic rings of biochar produced at a higher temperature may also act as π-electron donors owing to lower carboxyl functional groups (Zheng et al., 2013). However, the biochar produced at lower temperature serves as π-electron acceptor due to the presence of aromatic carbon in biochar; whereas, the heterocyclic ringed structure of atrazine serves as π-electron donor, subsequently resulting in stronger EDA interactions (Zhang et al., 2011). Furthermore, the chlorine substituent in atrazine makes the associated aromatic structures electron acceptors because of its electron retreating nature. This could interrelate EDA interactions by aromatic carbon on the biochar surface. Wang et al. (2020) reported the similar finding stating that the heterocyclic rings of atrazine molecule serve as π-electron donors, subsequently generating π-π EDA interactions with biochars. These interactions are suggested to be much stronger than Van der Waals forces (Keiluweit and Kleber, 2009). Therefore, the high adsorption affinity between biochars and atrazine might be the result of specific interactions between the surfaces of the biochar and pesticide molecules (Zhang et al., 2013).
Hence, the removal of atrazine through biochar is a complex process involving various mechanisms individually and simultaneously. Due to the heterogeneous properties of various biochars based on feedstock types and pyrolysis conditions, the performance of biochar is not consistent. Researchers around the globe are trying to develop biochar with super-adsorption efficiencies by optimizing their properties. Several pathways including physical, chemical (polymers, acids, and bases), biological, and/or mechanical means have been employed to modify the physicochemical, surface, and structural characteristics of the biochars to enhance their atrazine adsorption capacities.
Carbon nanotubes (CNTs) are made up of graphitic as well as several concentric tubules and are considered a new class of nanomaterial. The CNTs could either be single-walled (SWCNTs) or multi-walled (MWCNTs). Both of these types are sole macromolecules with one-dimensional structure, and thermally stable with certain chemical properties (Ren et al., 2011; Taghizade Firozjaee et al., 2018; Ahmad et al., 2019). CNTs are characterized with large surface area, tunable physical, chemical, and electrical characteristics, as well as small, hollow, and layered structures (Ahmad et al., 2019). The adsorption capacity of the CNTs towards pesticides is influenced by pore structure and functional groups that can be acquired by thermal and chemical modifications to gain the optimum performance for a targeted purpose (Yunus et al., 2012). Functional groups have the ability to alter the moisture contents on the surface of CNTs, which may help in the sorption of low molecular weight and polar compounds (Cho et al., 2008). Due to their unique characteristics, CNTs have come up recently as potential adsorbents for the removal of organic, inorganic, and biological contaminants from wastewater streams (Upadhyayula et al., 2009). However, the wastewater treatment efficiencies of CNTs mainly rely on the characteristics of the CNTs such as purity, surface area, and functional groups, as well as the adsorption conditions such as pH, temperature, ionic strength, contact time, competitive adsorption, dissolved organic matter, and initial contaminant concentration.
There is extensive information available on the application of CNTs for removal of various environmental pollutants from wastewater streams; however, the studies regarding atrazine adsorption onto CNTs are limited (Chen et al., 2009). Due to the presence of sp2 hybridized carbon atoms, CNTs can potentially attach to aromatic compounds such as atrazine via π-π EDA interactions (Chen et al., 2009). Furthermore, dispersion, hydrophobic effect, weak dipolar forces, and diffusion could also help in the adsorption of atrazine onto CNTs (Yang et al., 2006). The adsorption of atrazine onto various CNTs has been enlisted in Table 3. It is obvious from Table 3, that r-MWNTs exhibited the highest atrazine adsorption capacity as predicted by Langmuir isotherm (110.8, 100.42, and 97.05 mg g−1 at 18, 25, and 35°C, respectively). The highest adsorption capacities of the r-MWNTs could be attributed to the highest surface area of the said adsorbent (299.63 cm2 g−1) as compared to the other CNTs adsorbents mentioned (Yan et al., 2008). Yan et al. (2008) further stated that due to the higher isoelectric point, the r-MWNTs contained a higher number of O-containing functional groups, which subsequently aided the atrazine adsorption onto it. Further, it was also stated that the adsorption process of atrazine onto r-MWNTs was spontaneous and exothermic (Yan et al., 2008). A study conducted by Rambabu et al. (2012) demonstrated a relatively higher adsorption capacity (66.5, 63.7, 60.8 mg g−1 at 18, 23, and 31°C, respectively) of CNTs as predicted by the Langmuir isotherm, which could also be attributed to the higher surface area (189 cm2 g−1) of the said CNTs. They also explained that the adsorption of atrazine onto CNTs was an exothermic and spontaneous process. All the other types of CNTs reported in Table 3 represented relatively lower atrazine adsorption capacities, which could be designated to their comparatively lower surface areas and other physio-chemical and structural characteristics.
TABLE 3. Efficiency of various carbon nanotubes (CNTs), single-walled carbon nanotubes (SWCNTs), and multi-walled carbon nanotubes (MWCNTs) for the removal of atrazine from aqueous media as predicted by Langmuir and Freundlich isotherms.
The performance of CNTs is not always uniform due to their agglomeration in the aqueous solution and deficiency of polar functional groups (Ahmad et al., 2019). Therefore, a lot of researchers are focusing on modifying the CNTs for improved adsorption capacity for a range of contaminants removal from wastewater streams. However, again, the modified CNTs do not always show an improvement in their adsorption capacity compared with the pristine ones. For instance, Shi et al. (2010) modified the CNTs with various surfactants i.e., cetyltrimethylammonium bromide, sodium dodecylbenzene sulfonate, and humic acid, and reported that the presence of the surfactants inhibited the adsorption of atrazine onto both SWCNTs and MWCNTs (Table 3). The hydrophilic fraction of the aforementioned surfactant micelles faced in water converts the modified CNTs into more hydrophilic adsorbents, consequently reducing the adsorption of atrazine onto the modified CNTs. The inhibitory effects of anionic (sodium dodecylbenzene sulfonate) surfactants were higher than that of cationic (cetyltrimethylammonium bromide) surfactants. Due to lower purity and more oxygen contents, the presence of O-containing functional groups on pristine and modified SWCNTs enhances the adsorption of atrazine, thereby reducing the inhibitory effects, as compared to pristine and modified MWCNTs adsorption (Shi et al., 2010).
It has been established that CNTs possess higher adsorption potentials for both organic and inorganic contaminants. The major mechanisms controlling atrazine adsorption onto CNTs could be either micropore diffusion or surface adsorption (Chen et al., 2008). Therefore, the adsorption of atrazine onto CNTs may follow either a single or more than one mechanisms such as hydrophobic effect, π-π EDA interactions, covalent bonding, electrostatic interactions, and H-bonding (Yang et al., 2008; Pyrzynska, 2011). Generally, the organic molecules with C=C bonds and aromatic rings are adsorbed onto the CNTs through π-π EDA interactions, H-bonding with the surface functional groups, and electrostatic interactions (Chen et al., 2007b; Yang et al., 2008; Ji et al., 2009; Ghaedi and Kokhdan, 2012; Smith and Rodrigues 2015). However, numerous studies have demonstrated that higher π-π EDA interactions are one of the most significant mechanisms for the adsorption of an organic compound with aromatic rings onto CNTs (Hyung and Kim, 2008; Lin and Xing, 2008). Besides, Shi et al. (2010) reported that surface area and pore volume (both micropore and mesopores) distribution significantly affect the atrazine adsorption onto CNTs. On the other hand, Yan et al. (2008) reported that surface area and O-containing functional groups are the major factors controlling the adsorption of atrazine onto r-MWNTs. Likewise, Ellerie et al. (2013) demonstrated that the surface area of the CNTs was the major factor controlling its adsorption efficiency towards atrazine. Contrarily, Chingombe et al. (2006) demonstrated that higher O-containing functional groups may adsorb more H2O molecules and resulting in the generation of clusters, subsequently reducing the adoption of atrazine. The reduction in atrazine adsorption with increased O-containing function groups (carboxylic groups) could also be attributed to the deprotonation property of the aforementioned functional groups (Chen et al., 2009). Additionally, the pore filling is also considered an important mechanism for the adsorption of atrazine onto CNTs (Chen et al., 2009). In the aqueous media, the CNTs combine to create bundles, and the tiny empty spaces between the tubes in these bundles behaving like pores are filled with atrazine molecules. Therefore, the adsorption of atrazine onto CNTs is a complex process and depends on multiple factors including the characteristics of adsorbent as well as the adsorption conditions. Hence, detailed information about the characteristics of CNTs must be known before its implications for the removal of atrazine from wastewater streams.
Wastewater effluent from domestic households and industries containing huge amounts of organic and inorganic toxins, such as atrazine, must be treated before discharging into the ecosystem in order to reduce the environmental and health risks (Rashed, 2013; Roccaro et al., 2013; Zheng et al., 2013). Among various wastewater treatment technologies, adsorption through graphene has attracted a significant deal of attention from the researchers owing to its cost-effectiveness, easy operation, and higher efficiencies (Ali et al., 2017; Ali et al., 2018). Graphene is a well-known adsorbent for adsorption of a range of environmental pollutants including both organic and inorganic compounds. In recent years, graphene has attracted great consideration due to its physical, chemical, and mechanical characteristics. An enormous system of delocalized π-electrons, higher surface area, thermal and chemical stability, and abundance of adsorption sites, make it a potential candidate for wastewater treatment processes (Gopalakrishnan et al., 2015). Graphene is found in various forms including subtracted bounded monolayer graphene, graphene oxide (GO), reduced graphene oxide (rGO), and graphene nanosheets (GNS) (Sanchez et al., 2012). Graphene oxide is an enormously oxidative graphene form, developed by the chemical exfoliation of graphite (Ku and Park 2013). These are comprised of epoxy and hydroxyl groups at the base plane, carboxyl and carbonyl groups at the ends, and oxygen functional groups at their graphitic back (Kim et al., 2010). Analogous to graphene, GOs may also act as effective adsorbents owing to their distinct features including higher water solubility, better functionalities of O-containing surface, and theoretically big surface area (Sitko et al., 2013). On the other hand, rGOs are very imperfect forms of graphene and are produced by the chemical reduction of GOs, as well as hydrothermal and thermal annealing (Zhao et al., 2015).
Graphene-based adsorbents are efficient for the removal of organic pollutants owing to their light weight, mechanically strong, and higher surface area as well as abundant functional groups (Zhang et al., 2018). O-containing functional groups and π-π EDA of graphene-based adsorbents serve as binding sites for the adsorption of atrazine. However, a decline in atrazine adsorption is expected with an increase in reduced GOs owing to lower O-containing functional groups, consequently resulting in weaker surface complexations (Zhang et al., 2018). Therefore, much attention has been given to developing nano-graphene composites with supreme properties in recent times, because it has been established that an ideal adsorbent should remove multiple pollutants simultaneously.
Several graphene-based nanomaterials have been developed with targeted applicability so far. Nanocomposite of rGOs has successfully been used for adsorption of harmful pesticides, such as atrazine from aqueous media (Boruah et al., 2017). The rGO sorption features are considered to be really efficient for wastewater treatment (Ramesha et al., 2011; Maliyekkal et al., 2013; Choudhury and Balasubramanian, 2014). Novel rGO composites with corn straws-derived biochar (rGO-BC) were synthesized and their atrazine adsorption behavior has been investigated by Zhang et al. (2018). They reported that the surface of BC coated with rGO nanosheets by EDA interactions retained the original morphology even after forging. The synthesized nanocomposites rGO-BC thus exhibited an atrazine adsorption capacity of 67.55 mg g−1 with regeneration of 81% after four times (Zhang et al., 2018). Likewise, Murkherjee et al. (2011) fabricated the composite ultrafiltration (UF) membranes with GO as the base on macroporous ceramic support tubes by a new methodology and reported >95% removal of atrazine.
Lee et al. (2018) synthesized a nanocomposite of rGOs incorporated porous agarose (Ar-rGO) via the sol-gel method. Due to the synergism between the rGO-sheets and the agarose bundles, the resultant Ar-rGO nanocomposites exhibited excellent adsorption characteristics towards organic contaminants. The agarose bundles in the composite served as a water binding site, whereas, the rGOs acted as an active binding site for the organic pollutant, consequently removing higher amounts of the organic contaminants (Lee et al., 2018). The degradation of atrazine through reduced graphene (GR) composites is influenced by various factors such as temperature, pH, organic matters, and dosages of incorporated materials. Wu et al. (2018) observed an increase in atrazine removal efficiencies at high temperatures, as well as in acidic pH levels, whereas, the contents of organic matter did not affect the degradation of atrazine at lower concentrations. Therefore, the atrazine removal process using graphene as adsorbents must be optimized for the aforementioned conditions for higher and more efficient atrazine removal.
The removal of atrazine from wastewater through graphene may follow an individual or a combination of various processes, e.g., H-bonding, electrostatic interactions, pore diffusion, and π-π EDA interactions depending upon the type of adsorptive material and adsorption conditions (Tripathi and Ranjan, 2015; Singh and Gupta, 2016). Among all the aforementioned processes, π-π EDA interactions are the most dominant mechanism for adsorption of atrazine onto graphene.
The adsorption of atrazine onto graphene is largely dependent on the type and characteristics of graphene used, as well as on the adsorbent conditions. For instance, a higher number of O-containing functional groups in GOs enhances its binding capability for positively charged atrazine molecules through electrostatic interaction, consequently removing a higher amount of atrazine (Chen et al., 2013). Further, the O-containing functional groups present on graphene and N–H groups of atrazine could attach via H-bonding, resulting in atrazine adsorption. Besides, the higher surface area and pore volumes of the graphene play a major role in the adsorption of atrazine via pore-filling mechanism (Zhang et al., 2018). Contrarily, the abundance of O-containing functional groups may reduce the adsorption of atrazine onto graphene via generating hydrophilic surfaces. However, the O-containing functional groups of graphene may remove after its annealing reduction, consequently increasing the π-π EDA interactions (Zhang et al., 2018). In a study conducted by Li et al. (2013), it was reported that the atrazine adsorption capacity of graphene was attributed to surface area and π-π EDA interactions. Moreover, besides adsorption, the atrazine can also be degraded with the help of graphene. For instance, Wu et al. (2018) studied the degradation potential of graphene composites with nanoscale zero-valent iron and persulfate for atrazine removal. The results suggested that atrazine degradation followed multiple mechanisms including dealkylation, dechlorination-hydroxylation, allylic oxidation, and allylic hydroxylation. Therefore, it can be stated that the removal of atrazine from contaminated wastewater through graphene follows multiple mechanisms including adsorption and degradation.
Atrazine and its metabolites have been detected in the atmosphere, water bodies, soil, and sediments all around the world. In the majority of cases, the concentrations of atrazine in wastewater exceed the maximum permissible levels for drinking water i.e., 3 μg L−1 in the United States, 0.1 μg L−1 in Europe, and 3.0 μg L−1 by WHO. The particulate, as well as vapor phases of atrazine, have been found even 100–300 km from the application site (Dorsey 2003). Likewise, it is mobile in soil and aquatic environment and can potentially migrate from one place to another. There is a limited degradation of atrazine in soil via chemical and biological means, however, no biodegradation of atrazine in groundwater has been reported. Moreover, the degradation of atrazine in the surface water is very low and has not been demonstrated well (Dorsey, 2003). Hence, all the available data indicated that atrazine is high recalcitrance in nature and may persist in water bodies for decades without degradation. However, if degraded, its metabolites could be even more toxic than the pristine atrazine. Therefore, the focus should be drawn to removing the recalcitrant atrazine and its metabolites from world water resources on urgent bases. The following directions could be followed to cope with this situation:
• Atrazine application should be prohibited in all the countries where atrazine utilization is still in practice.
• Monitoring/surveillance of atrazine and its metabolites in surface and ground water resources surrounding 100–300 km should be performed. Moreover, levels of atrazine and its by-products in soils and sediments should be studied, as these could serve as a sink for atrazine introduction into nearby water bodies.
• Behavior/fate of atrazine, as well as its metabolites in various environments, should be investigated.
• A comprehensive study to explore the potential impacts of atrazine on humans, amphibians, and other animals should be conducted.
• Efficient technologies should be identified for the complete removal of atrazine and its metabolites from water resources. The existing carbonaceous adsorbent should be modified via chemical, mechanical, thermal, ozone, plasma, and/or electro activities to improve their performance and efficiency.
• Carbonaceous nano-adsorbents should be fabricated and modified with metal oxide nanoparticles and applied for atrazine removal from wastewater.
• Novel carbonaceous adsorbents with higher regeneration/reusability should be developed for more sustainable management of atrazine-contaminated media.
MA conceptualized and designed the study, performed the literature review, drafted the manuscript, and approved the final manuscript as submitted. UR and SI reviewed identified articles to determine if they met the defined study inclusion and exclusion criteria, generated tables, and critically discussed the extracted data. JA and HR performed the screening of obtained articles based on title, abstract, and full text, interpreted extracted data, and edited the manuscript draft. AA-F reviewed and edited the manuscript draft. MA-W critically reviewed the manuscript, edited the final manuscript, and approved the final manuscript as submitted. All of the authors approved the final manuscript as submitted and agree to be accountable for all aspects of the work.
The authors declare that the research was conducted in the absence of any commercial or financial relationships that could be construed as a potential conflict of interest.
All claims expressed in this article are solely those of the authors and do not necessarily represent those of their affiliated organizations, or those of the publisher, the editors, and the reviewers. Any product that may be evaluated in this article, or claim that may be made by its manufacturer, is not guaranteed or endorsed by the publisher.
The authors extend their appreciation to the Deanship of Scientific Research, King Saud University, for funding this work through the international research group project RG-1439-043.
Abd, A. A., Naji, S. Z., Hashim, A. S., and Othman, M. R. (2020). Carbon Dioxide Removal through Physical Adsorption Using Carbonaceous and Non-carbonaceous Adsorbents: a Review. J. Environ. Chem. Eng. 8 (5), 104142. doi:10.1016/j.jece.2020.104142
Adams, C. D., and Watson, T. L. (1996). Treatability Ofs-Triazine Herbicide Metabolites Using Powdered Activated Carbon. J. Environ. Eng. 122 (4), 327–330. doi:10.1061/(asce)0733-9372(1996)122:4(327)
Agani, I., Fatombi, J. K., Osseni, S. A., Idohou, E. A., Neumeyer, D., Verelst, M., et al. (2020). Removal of Atrazine from Aqueous Solutions onto a Magnetite/chitosan/activated Carbon Composite in a Fixed-Bed Column System: Optimization Using Response Surface Methodology. RSC Adv. 10 (68), 41588–41599. doi:10.1039/d0ra07873e
Ahmad, J., Naeem, S., Ahmad, M., Usman, A. R. A., and Al-Wabel, M. I. (2019). A Critical Review on Organic Micropollutants Contamination in Wastewater and Removal through Carbon Nanotubes. J. Environ. Manag. 246, 214–228. doi:10.1016/j.jenvman.2019.05.152
Ahmad, M., Usman, A. R. A., Al-Faraj, A. S., Ahmad, M., Sallam, A., and Al-Wabel, M. I. (2018). Phosphorus-loaded Biochar Changes Soil Heavy Metals Availability and Uptake Potential of Maize (Zea mays L.) Plants. Chemosphere 194, 327–339. doi:10.1016/j.chemosphere.2017.11.156
Ahmad, R., Kookana, R. S., Alston, A. M., and Skjemstad, J. O. (2001). The Nature of Soil Organic Matter Affects Sorption of Pesticides. 1. Relationships with Carbon Chemistry as Determined by 13C CPMAS NMR Spectroscopy. Environ. Sci. Technol. 35 (5), 878–884. doi:10.1021/es001446i
Ahmed, M. B., Zhou, J. L., Ngo, H. H., and Guo, W. (2015). Adsorptive Removal of Antibiotics from Water and Wastewater: Progress and Challenges. Sci. Total Environ. 532, 112–126. doi:10.1016/j.scitotenv.2015.05.130
Ali, I., Alharbi, O. M. L., Alothman, Z. A., Badjah, A. Y., Alwarthan, A., and Basheer, A. A. (2018). Artificial Neural Network Modelling of Amido Black Dye Sorption on Iron Composite Nano Material: Kinetics and Thermodynamics Studies. J. Mol. Liq. 250, 1–8. doi:10.1016/j.molliq.2017.11.163
Ali, I., Alothman, Z. A., and Alwarthan, A. (2017). Uptake of Propranolol on Ionic Liquid Iron Nanocomposite Adsorbent: Kinetic, Thermodynamics and Mechanism of Adsorption. J. Mol. Liq. 236, 205–213. doi:10.1016/j.molliq.2017.04.028
Álvarez, P. M., Beltrán, F. J., Gómez-Serrano, V., Jaramillo, J., and Rodríguez, E. M. (2004). Comparison between Thermal and Ozone Regenerations of Spent Activated Carbon Exhausted with Phenol. Water Res. 38 (8), 2155–2165.
Anjum, H., Johari, K., Appusamy, A., Gnanasundaram, N., and Thanabalan, M. (2019). Surface Modification and Characterization of Carbonaceous Adsorbents for the Efficient Removal of Oil Pollutants. J. Hazard. Mater. 379, 120673. doi:10.1016/j.jhazmat.2019.05.066
Ateia, M., Koch, C., Jelavić, S., Hirt, A., Quinson, J., Yoshimura, C., et al. (2017). Green and Facile Approach for Enhancing the Inherent Magnetic Properties of Carbon Nanotubes for Water Treatment Applications. PloS one 12 (7), e0180636. doi:10.1371/journal.pone.0180636
Baccar, R., Bouzid, J., Feki, M., and Montiel, A. (2009). Preparation of Activated Carbon from Tunisian Olive-Waste Cakes and its Application for Adsorption of Heavy Metal Ions. J. Hazard Mater 162 (2-3), 1522–1529. doi:10.1016/j.jhazmat.2008.06.041
Bandura, L., Panek, R., Rotko, M., and Franus, W. (2016). Synthetic Zeolites from Fly Ash for an Effective Trapping of BTX in Gas Stream. Microporous Mesoporous Mater. 223, 1–9. doi:10.1016/j.micromeso.2015.10.032
Barr, D. B., Panuwet, P., Nguyen, J. V., Udunka, S., and Needham, L. L. (2007). Assessing Exposure to Atrazine and its Metabolites Using Biomonitoring. Environ. Health Perspect. 115 (10), 1474–1478. doi:10.1289/ehp.10141
Baudu, M., Raveau, D., and Guibaud, G. (2004). Application of the IAS Theory Combining to a Three Compartiments Description of Natural Organic Matter to the Adsorption of Atrazine or Diuron on Activated Carbon. Environ. Technol. 25 (7), 763–773. doi:10.1080/09593330.2004.9619367
Baup, S., Jaffre, C., Wolbert, D., and Laplanche, A. (2000). Adsorption of Pesticides onto Granular Activated Carbon: Determination of Surface Diffusivities Using Simple Batch Experiments. Adsorption 6 (3), 219–228. doi:10.1023/a:1008937210953
Bohn, T., Cocco, E., Gourdol, L., Guignard, C., and Hoffmann, L. (2011). Determination of Atrazine and Degradation Products in Luxembourgish Drinking Water: Origin and Fate of Potential Endocrine-Disrupting Pesticides. Food Addit. Contam. Part A 28 (8), 1041–1054. doi:10.1080/19440049.2011.580012
Boruah, P. K., Sharma, B., Hussain, N., and Das, M. R. (2017). Magnetically Recoverable Fe3O4/graphene Nanocomposite towards Efficient Removal of Triazine Pesticides from Aqueous Solution: Investigation of the Adsorption Phenomenon and Specific Ion Effect. Chemosphere 168, 1058–1067. doi:10.1016/j.chemosphere.2016.10.103
Brown, N. W., Roberts, E. P. L., Chasiotis, A., Cherdron, T., and Sanghrajka, N. (2004). Atrazine Removal Using Adsorption and Electrochemical Regeneration. Water Res. 38 (13), 3067–3074. doi:10.1016/j.watres.2004.04.043
Budinova, T., Ekinci, E., Yardim, F., Grimm, A., Björnbom, E., Minkova, V., et al. (2006). Characterization and Application of Activated Carbon Produced by H3PO4 and Water Vapor Activation. Fuel Process. Technol. 87 (10), 899–905. doi:10.1016/j.fuproc.2006.06.005
Chang, S.-W., Lee, S.-J., and Je, C.-H. (2005). Phytoremediation of Atrazine by Poplar Trees: Toxicity, Uptake, and Transformation. J. Environ. Sci. Health, Part B 40 (6), 801–811. doi:10.1080/03601230500227483
Chen, C., Zhou, W., and Lin, D. (2015). Sorption Characteristics of N-Nitrosodimethylamine onto Biochar from Aqueous Solution. Bioresour. Technol. 179, 359–366. doi:10.1016/j.biortech.2014.12.059
Chen, G.-C., Shan, X.-Q., Wang, Y.-S., Pei, Z.-G., Shen, X.-E., Wen, B., et al. (2008). Effects of Copper, Lead, and Cadmium on the Sorption and Desorption of Atrazine onto and from Carbon Nanotubes. Environ. Sci. Technol. 42 (22), 8297–8302. doi:10.1021/es801376w
Chen, G. C., Shan, X. Q., Zhou, Y. Q., Shen, X. E., Huang, H. L., and Khan, S. U. (2009). Adsorption Kinetics, Isotherms and Thermodynamics of Atrazine on Surface Oxidized Multiwalled Carbon Nanotubes. J. Hazard Mater 169 (1-3), 912–918. doi:10.1016/j.jhazmat.2009.04.034
Chen, W., Duan, L., and Zhu, D. (2007a). Adsorption of Polar and Nonpolar Organic Chemicals to Carbon Nanotubes. Environ. Sci. Technol. 41 (24), 8295–8300. doi:10.1021/es071230h
Chen, W., Parette, R., Zou, J., Cannon, F. S., and Dempsey, B. A. (2007b). Arsenic Removal by Iron-Modified Activated Carbon. Water Res. 41 (9), 1851–1858. doi:10.1016/j.watres.2007.01.052
Chen, X., Xia, X., Wang, X., Qiao, J., and Chen, H. (2011). A Comparative Study on Sorption of Perfluorooctane Sulfonate (PFOS) by Chars, Ash and Carbon Nanotubes. Chemosphere 83 (10), 1313–1319. doi:10.1016/j.chemosphere.2011.04.018
Chen, Y., Chen, L., Bai, H., and Li, L. (2013). Graphene Oxide-Chitosan Composite Hydrogels as Broad-Spectrum Adsorbents for Water Purification. J. Mat. Chem. A 1 (6), 1992–2001. doi:10.1039/c2ta00406b
Chen, Z., Chen, B., Zhou, D., and Chen, W. (2012). Bisolute Sorption and Thermodynamic Behavior of Organic Pollutants to Biomass-Derived Biochars at Two Pyrolytic Temperatures. Environ. Sci. Technol. 46 (22), 12476–12483. doi:10.1021/es303351e
Chingombe, P., Saha, B., and Wakeman, R. J. (2006). Sorption of Atrazine on Conventional and Surface Modified Activated Carbons. J. Colloid Interface Sci. 302 (2), 408–416. doi:10.1016/j.jcis.2006.06.065
Cho, H.-H., Smith, B. A., Wnuk, J. D., Fairbrother, D. H., and Ball, W. P. (2008). Influence of Surface Oxides on the Adsorption of Naphthalene onto Multiwalled Carbon Nanotubes. Environ. Sci. Technol. 42 (8), 2899–2905. doi:10.1021/es702363e
Chowdhury, S., and Balasubramanian, R. (2014). Recent Advances in the Use of Graphene-Family Nanoadsorbents for Removal of Toxic Pollutants from Wastewater. Adv. colloid interface Sci. 204, 35–56. doi:10.1016/j.cis.2013.12.005
Clausen, L., and Fabricius, I. (2001). Atrazine, Isoproturon, Mecoprop, 2,4-D, and Bentazone Adsorption onto Iron Oxides. J. Environ. Qual. 30 (3), 858–869. doi:10.2134/jeq2001.303858x
Davies, J. E. D., and Jabeen, N. (2003). The Adsorption of Herbicides and Pesticides on Clay Minerals and Soils. Part 2. Atrazine. J. inclusion Phenom. Macrocycl. Chem. 46 (1), 57–64. doi:10.1023/a:1025604713256
Deng, H., Yu, H. M., Chen, M., and Ge, C. J. (2014). Sorption of Atrazine by Biochar Prepared from Manioc Wastes in Tropical Soils. Adv. Mater. Res. 878, 433–442. doi:10.4028/www.scientific.net/amr.878.433
Ding, L., Snoeyink, V. L., Mariñas, B. J., Yue, Z., and Economy, J. (2008). Effects of Powdered Activated Carbon Pore Size Distribution on the Competitive Adsorption of Aqueous Atrazine and Natural Organic Matter. Environ. Sci. Technol. 42 (4), 1227–1231. doi:10.1021/es0710555
Dorsey, A. (2003). Toxicological Profile for Atrazine. Atlanta, Georgia: Agency for Toxic Substances and Disease Registry.
Dutta, T., Kim, T., Vellingiri, K., Tsang, D. C. W., Shon, J. R., Kim, K.-H., et al. (2019). Recycling and Regeneration of Carbonaceous and Porous Materials through Thermal or Solvent Treatment. Chem. Eng. J. 364, 514–529. doi:10.1016/j.cej.2019.01.049
Ellerie, J. R., Apul, O. G., Karanfil, T., and Ladner, D. A. (2013). Comparing Graphene, Carbon Nanotubes, and Superfine Powdered Activated Carbon as Adsorptive Coating Materials for Microfiltration Membranes. J. Hazard. Mater. 261, 91–98. doi:10.1016/j.jhazmat.2013.07.009
Elwakeel, K. Z., Elgarahy, A. M., and Mohammad, S. H. (2017). Use of Beach bivalve Shells Located at Port Said Coast (Egypt) as a Green Approach for Methylene Blue Removal. J. Environ. Chem. Eng. 5 (1), 578–587. doi:10.1016/j.jece.2016.12.032
Ersan, G., Kaya, Y., Ersan, M. S., Apul, O. G., and Karanfil, T. (2019). Adsorption Kinetics and Aggregation for Three Classes of Carbonaceous Adsorbents in the Presence of Natural Organic Matter. Chemosphere 229, 515–524. doi:10.1016/j.chemosphere.2019.05.014
Faur, C., Métivier-Pignon, H., and Le Cloirec, P. (2005). Multicomponent Adsorption of Pesticides onto Activated Carbon Fibers. Adsorption 11 (5), 479–490. doi:10.1007/s10450-005-5607-2
Gao, Y., Jiang, Z., Li, J., Xie, W., Jiang, Q., Bi, M., et al. (2019). A Comparison of the Characteristics and Atrazine Adsorption Capacity of Co-pyrolysed and Mixed Biochars Generated from Corn Straw and Sawdust. Environ. Res. 172, 561–568. doi:10.1016/j.envres.2019.03.010
Ghaedi, M., and Kokhdan, S. N. (2012). Oxidized Multiwalled Carbon Nanotubes for the Removal of Methyl Red (MR): Kinetics and Equilibrium Study. Desalination Water Treat. 49 (1-3), 317–325. doi:10.1080/19443994.2012.719355
Ghosh, P. K., and Philip, L. (2005). Performance Evaluation of Waste Activated Carbon on Atrazine Removal from Contaminated Water. J. Environ. Sci. Health, Part B 40 (3), 425–441. doi:10.1081/pfc-200047576
Gkementzoglou, C., Kotrotsiou, O., Koronaiou, M., and Kiparissides, C. (2016). Development of a Sandwich-type Filtration Unit Packed with MIP Nanoparticles for Removal of Atrazine from Water Sources. Chem. Eng. J. 287, 233–240. doi:10.1016/j.cej.2015.11.018
Gonzalez-Serrano, E., Cordero, T., Rodriguez-Mirasol, J., Cotoruelo, L., and Rodriguez, J. J. (2004). Removal of Water Pollutants With Activated Carbons Prepared From H3PO4 Activation of Lignin From Kraft Black Liquors. Water Res. 38 (13), 3043–3050. doi:10.1016/j.watres.2004.04.048
Gopalakrishnan, A., Krishnan, R., Thangavel, S., Venugopal, G., and Kim, S.-J. (2015). Removal of Heavy Metal Ions from Pharma-Effluents Using Graphene-Oxide Nanosorbents and Study of Their Adsorption Kinetics. J. Industrial Eng. Chem. 30, 14–19. doi:10.1016/j.jiec.2015.06.005
Gupta, V. K., Gupta, B., Rastogi, A., Agarwal, S., and Nayak, A. (2011). Pesticides Removal from Waste Water by Activated Carbon Prepared from Waste Rubber Tire. Water Res. 45 (13), 4047–4055. doi:10.1016/j.watres.2011.05.016
Han, Y., Boateng, A. A., Qi, P. X., Lima, I. M., and Chang, J. (2013). Heavy Metal and Phenol Adsorptive Properties of Biochars from Pyrolyzed Switchgrass and Woody Biomass in Correlation with Surface Properties. J. Environ. Manag. 118, 196–204. doi:10.1016/j.jenvman.2013.01.001
Hatfield, S. E. (2010). Applications of Triazine Chemistry: Education, Remediation, and Drug Delivery. Doctoral dissertation (College Station, TX, USA: Texas A & M University).
He, H., Liu, Y., You, S., Liu, J., Xiao, H., and Tu, Z. (2019). A Review on Recent Treatment Technology for Herbicide Atrazine in Contaminated Environment. Int. J. Environ. Res. Public Health 16 (24), 5129. doi:10.3390/ijerph16245129
Hyung, H., and Kim, J.-H. (2008). Natural Organic Matter (NOM) Adsorption to Multi-Walled Carbon Nanotubes: Effect of NOM Characteristics and Water Quality Parameters. Environ. Sci. Technol. 42 (12), 4416–4421. doi:10.1021/es702916h
Inyang, M., Gao, B., Zimmerman, A., Zhang, M., and Chen, H. (2014). Synthesis, Characterization, and Dye Sorption Ability of Carbon Nanotube-Biochar Nanocomposites. Chem. Eng. J. 236, 39–46. doi:10.1016/j.cej.2013.09.074
Jablonowski, N. D., Köppchen, S., Hofmann, D., Schäffer, A., and Burauel, P. (2009). Persistence of 14C-Labeled Atrazine and its Residues in a Field Lysimeter Soil after 22years. Environ. Pollut. 157 (7), 2126–2131. doi:10.1016/j.envpol.2009.02.004
Jablonowski, N. D., Schäffer, A., and Burauel, P. (2011). Still Present after All These Years: Persistence Plus Potential Toxicity Raise Questions about the Use of Atrazine. Environ. Sci. Pollut. Res. 18 (2), 328–331. doi:10.1007/s11356-010-0431-y
Jain, S., Yamgar, R., and Jayaram, R. V. (2009). Photolytic and Photocatalytic Degradation of Atrazine in the Presence of Activated Carbon. Chem. Eng. J. 148 (2-3), 342–347. doi:10.1016/j.cej.2008.09.006
Ji, L., Chen, W., Duan, L., and Zhu, D. (2009). Mechanisms for Strong Adsorption of Tetracycline to Carbon Nanotubes: a Comparative Study Using Activated Carbon and Graphite as Adsorbents. Environ. Sci. Technol. 43 (7), 2322–2327. doi:10.1021/es803268b
Jia, Y., Wang, R., Fane, A. G., and Krantz, W. B. (2005). Effect of Air Bubbling on Atrazine Adsorption in Water by Powdered Activated Carbons–Competitive Adsorption of Impurities. Sep. Purif. Technol. 46 (1-2), 79–87. doi:10.1016/j.seppur.2005.04.018
Jiang, Z.-Y., Ma, Y.-K., Ke, Q.-F., Chu, L.-F., Guo, C.-X., and Guo, Y.-P. (2021). Hydrothermal Deposition of CoFe2O4 Nanoparticles on Activated Carbon Fibers Promotes Atrazine Removal via Physical Adsorption and Photo-Fenton Degradation. J. Environ. Chem. Eng. 9 (5), 105940. doi:10.1016/j.jece.2021.105940
Karakoyun, N., Kubilay, S., Aktas, N., Turhan, O., Kasimoglu, M., Yilmaz, S., et al. (2011). Hydrogel–Biochar Composites for Effective Organic Contaminant Removal from Aqueous Media. Desalination 280 (1-3), 319–325. doi:10.1016/j.desal.2011.07.014
Kasozi, G. N., Zimmerman, A. R., Nkedi-Kizza, P., and Gao, B. (2010). Catechol and Humic Acid Sorption onto a Range of Laboratory-Produced Black Carbons (Biochars). Environ. Sci. Technol. 44 (16), 6189–6195. doi:10.1021/es1014423
Keiluweit, M., and Kleber, M. (2009). Molecular-Level Interactions in Soils and Sediments: The Role of Aromatic π-Systems. Environ. Sci. Technol. 43 (10), 3421–3429. doi:10.1021/es8033044
Kim, J., Cote, L. J., Kim, F., Yuan, W., Shull, K. R., and Huang, J. (2010). Graphene Oxide Sheets at Interfaces. J. Am. Chem. Soc. 132 (23), 8180–8186. doi:10.1021/ja102777p
Kovaios, I. D., Paraskeva, C. A., Koutsoukos, P. G., and Payatakes, A. C. (2006). Adsorption of Atrazine on Soils: Model Study. J. Colloid Interface Sci. 299 (1), 88–94. doi:10.1016/j.jcis.2006.01.057
Ku, S. H., and Park, C. B. (2013). Myoblast Differentiation on Graphene Oxide. Biomaterials 34 (8), 2017–2023. doi:10.1016/j.biomaterials.2012.11.052
Kudaybergenov, K. K., Ongarbayev, E. K., and Mansurov, Z. A. (2010). Carbonaceous Composites from Agricultural Wastes for Adsorption of Hydrocarbon Contamination in Water. Eur. Chem. Tech. J. 12 (2), 151–156. doi:10.18321/ectj38
Kulikova, N. A., and Perminova, I. V. (2002). Binding of Atrazine to Humic Substances from Soil, Peat, and Coal Related to Their Structure. Environ. Sci. Technol. 36 (17), 3720–3724. doi:10.1021/es015778e
Laird, D. A., and Koskinen, W. C. (2008). Triazine Soil Interactions. triazine Herbic. 50, 275–299. doi:10.1016/b978-044451167-6.50024-6
Lee, S., Moon, B. J., Lee, H. J., Bae, S., Kim, T.-W., Jung, Y. C., et al. (2018). Enhancement of Adsorption Performance for Organic Molecules by Combined Effect of Intermolecular Interaction and Morphology in Porous rGO-Incorporated Hydrogels. ACS Appl. Mat. Interfaces 10 (20), 17335–17344. doi:10.1021/acsami.7b19102
Li, X., Ma, X., Huang, R., Xie, X., Guo, L., and Zhang, M. (2018). Synthesis of a Molecularly Imprinted Polymer on mSiO2 @Fe3 O4 for the Selective Adsorption of Atrazine. J. Sep. Sci. 41, 2837–2845. doi:10.1002/jssc.201800146
Li, Y., Du, Q., Liu, T., Peng, X., Wang, J., Sun, J., et al. (2013). Comparative Study of Methylene Blue Dye Adsorption onto Activated Carbon, Graphene Oxide, and Carbon Nanotubes. Chem. Eng. Res. Des. 91 (2), 361–368.
Lin, D., and Xing, B. (2008). Adsorption of Phenolic Compounds by Carbon Nanotubes: Role of Aromaticity and Substitution of Hydroxyl Groups. Environ. Sci. Technol. 42 (19), 7254–7259. doi:10.1021/es801297u
Liu, N., Charrua, A. B., Weng, C.-H., Yuan, X., and Ding, F. (2015). Characterization of Biochars Derived from Agriculture Wastes and Their Adsorptive Removal of Atrazine from Aqueous Solution: A Comparative Study. Bioresour. Technol. 198, 55–62. doi:10.1016/j.biortech.2015.08.129
Lladó, J., Lao-Luque, C., Ruiz, B., Fuente, E., Solé-Sardans, M., and Dorado, A. D. (2015). Role of Activated Carbon Properties in Atrazine and Paracetamol Adsorption Equilibrium and Kinetics. Process Saf. Environ. Prot. 95, 51–59.
Lu, H., Zhang, W., Yang, Y., Huang, X., Wang, S., and Qiu, R. (2012). Relative Distribution of Pb2+ Sorption Mechanisms by Sludge-Derived Biochar. Water Res. 46 (3), 854–862. doi:10.1016/j.watres.2011.11.058
Lupul, I., Yperman, J., Carleer, R., and Gryglewicz, G. (2015). Adsorption of Atrazine on Hemp Stem-Based Activated Carbons with Different Surface Chemistry. Adsorption 21 (6), 489–498. doi:10.1007/s10450-015-9689-1
Ma, L., and Selim, H. M. (1996). Atrazine Retention and Transport in Soils. Rev. Environ. Contam. Toxicol. 1996, 129–173. doi:10.1007/978-1-4612-2354-2_2
Mahía, J., Martín, A., Carballas, T., and Díaz-Raviña, M. (2007). Atrazine Degradation and Enzyme Activities in an Agricultural Soil under Two Tillage Systems. Sci. Total Environ. 378 (1-2), 187–194.
Maliyekkal, S. M., Sreeprasad, T. S., Krishnan, D., Kouser, S., Mishra, A. K., Waghmare, U. V., et al. (2013). Graphene: a Reusable Substrate for Unprecedented Adsorption of Pesticides. Small 9 (2), 273–283. doi:10.1002/smll.201201125
Mamchenko, A. V., Yakimova, T. I., and Koganovskii, A. M. (1982). The Mechanism of the Filling of the Micropores in Activated Charcoals during the Adsorption of Organic Substances Dissolved in Water. Russ. J. Phys. Chem. A 56 (5), 741–743.
Mandal, A., Singh, N., and Purakayastha, T. J. (2017). Characterization of Pesticide Sorption Behaviour of Slow Pyrolysis Biochars as Low Cost Adsorbent for Atrazine and Imidacloprid Removal. Sci. Total Environ. 577, 376–385. doi:10.1016/j.scitotenv.2016.10.204
Moh, H. T., Tan, I. A. W., and Lim, L. L. P. (2013). Removal of Atrazine from Water Using Oil Palm Shell Based Adsorbents: Equilibrium and Kinetic Study. J. Civ. Eng. Sci. Technol. 4 (2), 18–23. doi:10.33736/jcest.114.2013
Mohammadi, A., Sandberg, M., Venkatesh, G., Eskandari, S., Dalgaard, T., Joseph, S., et al. (2019). Environmental Performance of End-Of-Life Handling Alternatives for Paper-and-Pulp-Mill Sludge: Using Digestate as a Dource of Energy or for Biochar Production. Energy 182, 594–605. doi:10.1016/j.energy.2019.06.065
Moreno-Castilla, C. (2004). Adsorption of Organic Molecules From Aqueous Solutions on Carbon Materials. Carbon 42 (1), 83–94. doi:10.1016/j.carbon.2003.09.022
Mudhoo, A., and Garg, V. K. (2011). Sorption, Transport and Transformation of Atrazine in Soils, Minerals and Composts: a Review. Pedosphere 21 (1), 11–25. doi:10.1016/s1002-0160(10)60074-4
Mukherjee, A., Zimmerman, A. R., and Harris, W. (2011). Surface Chemistry Variations Among a Series of Laboratory-Produced Biochars. Geoderma 163 (3-4), 247–255. doi:10.1016/j.geoderma.2011.04.021
Murphy, E. M., Zachara, J. M., Smith, S. C., Phillips, J. L., and Wietsma, T. W. (1994). Interaction of Hydrophobic Organic Compounds with Mineral-Bound Humic Substances. Environ. Sci. Technol. 28 (7), 1291–1299. doi:10.1021/es00056a017
Nápoles-Armenta, J., Vidales-Contreras, J. A., Leyva-Soto, L. A., Meza-Escalante, E. R., Díaz-Tenorio, L. M., García-Gómez, C., et al. (2021). The Influence of the Configuration of Two Electrochemical Reactors on the Process of Removing Atrazine from Water. Sustainability 13 (9), 5267.
Nguyen, T. H., Cho, H.-H., Poster, D. L., and Ball, W. P. (2007). Evidence for a Pore-Filling Mechanism in the Adsorption of Aromatic Hydrocarbons to a Natural Wood Char. Environ. Sci. Technol. 41 (4), 1212–1217. doi:10.1021/es0617845
Oh, S.-Y., and Seo, Y.-D. (2016). Sorption of Halogenated Phenols and Pharmaceuticals to Biochar: Affecting Factors and Mechanisms. Environ. Sci. Pollut. Res. 23 (2), 951–961. doi:10.1007/s11356-015-4201-8
Ouyang, W., Zhao, X., Tysklind, M., and Hao, F. (2016). Typical Agricultural Diffuse Herbicide Sorption with Agricultural Waste-Derived Biochars Amended Soil of High Organic Matter Content. Water Res. 92, 156–163. doi:10.1016/j.watres.2016.01.055
Pelekani, C., and Snoeyink, V. L. (2000). Competitive Adsorption between Atrazine and Methylene Blue on Activated Carbon: the Importance of Pore Size Distribution. Carbon 38 (10), 1423–1436. doi:10.1016/s0008-6223(99)00261-4
Penn, C. J., Gonzalez, J. M., and Chagas, I. (2018). Investigation of Atrazine Sorption to Biochar with Titration Calorimetry and Flow-Through Analysis: Implications for Design of Pollution-Control Structures. Front. Chem. 6, 307. doi:10.3389/fchem.2018.00307
Pyrzynska, K. (2011). Carbon Nanotubes as Sorbents in the Analysis of Pesticides. Chemosphere 83 (11), 1407–1413. doi:10.1016/j.chemosphere.2011.01.057
Qu, X., Brame, J., Li, Q., and Alvarez, P. J. J. (2013). Nanotechnology for a Safe and Sustainable Water Supply: Enabling Integrated Water Treatment and Reuse. Acc. Chem. Res. 46 (3), 834–843. doi:10.1021/ar300029v
Rachel, N. Y., Daouda, K., Abdelaziz, B., Gaelle, D. D. E., Abdelrani, Y., and Mbadcam, K. J. (2016). International Journal of Engineering Sciences & Research Rechnology Influence of Modification of the Activated Carbon on the Adsorption of Atrazine: Equilibrium Study and Kinetics.
Rambabu, N., Guzman, C. A., Soltan, J., and Himabindu, V. (2012). Adsorption Characteristics of Atrazine on Granulated Activated Carbon and Carbon Nanotubes. Chem. Eng. Technol. 35 (2), 272–280. doi:10.1002/ceat.201100376
Ramesha, G. K., Vijaya Kumara, A., Muralidhara, H. B., and Sampath, S. (2011). Graphene and Graphene Oxide as Effective Adsorbents toward Anionic and Cationic Dyes. J. colloid interface Sci. 361 (1), 270–277. doi:10.1016/j.jcis.2011.05.050
Rashed, M. N. (2013). Adsorption Technique for the Removal of Organic Pollutants from Water and Wastewater. Org. pollutants-monitoring, risk Treat. 7, 167–194.
Ren, X., Chen, C., Nagatsu, M., and Wang, X. (2011). Carbon Nanotubes as Adsorbents in Environmental Pollution Management: a Review. Chem. Eng. J. 170 (2-3), 395–410. doi:10.1016/j.cej.2010.08.045
Roccaro, P., Sgroi, M., and Vagliasindi, F. G. (2013). Removal of Xenobiotic Compounds from Wastewater for Environment Protection: Treatment Processes and Costs. Chem. Eng. Trans. 32. doi:10.3303/CET1332085
Salvestrini, S., Sagliano, P., Iovino, P., Capasso, S., and Colella, C. (2010). Atrazine Adsorption by Acid-Activated Zeolite-Rich Tuffs. Appl. Clay Sci. 49 (3), 330–335. doi:10.1016/j.clay.2010.04.008
Sanchez, V. C., Jachak, A., Hurt, R. H., and Kane, A. B. (2012). Biological Interactions of Graphene-Family Nanomaterials: an Interdisciplinary Review. Chem. Res. Toxicol. 25 (1), 15–34. doi:10.1021/tx200339h
Serafini, C. G., Clerici, N. J., Della-Flora, I. K., Dupont, G. K., da Costa Cabrera, L., and Daroit, D. J. (2022). Effects of Atrazine on Soil Microbial Indicators and the Evaluation of Herbicide Attenuation in Microcosms. J. Soils Sediments 2022, 1–11. doi:10.1007/s11368-021-03121-8
Shao, S., Feng, Y., Yu, H., Li, J., Li, G., and Liang, H. (2017). Presence of an Adsorbent Cake Layer Improves the Performance of Gravity-Driven Membrane (GDM) Filtration System. Water Res. 108, 240–249. doi:10.1016/j.watres.2016.10.081
Sharma, R. K., Kumar, A., and Joseph, P. E. (2008). Removal of Atrazine from Water by Low Cost Adsorbents Derived from Agricultural and Industrial Wastes. Bull. Environ. Contam. Toxicol. 80 (5), 461–464. doi:10.1007/s00128-008-9389-6
Shi, B., Zhuang, X., Yan, X., Lu, J., and Tang, H. (2010). Adsorption of Atrazine by Natural Organic Matter and Surfactant Dispersed Carbon Nanotubes. J. Environ. Sci. 22 (8), 1195–1202. doi:10.1016/s1001-0742(09)60238-2
Shirmardi, M., Alavi, N., Lima, E. C., Takdastan, A., Mahvi, A. H., and Babaei, A. A. (2016). Removal of Atrazine as an Organic Micro-pollutant from Aqueous Solutions: a Comparative Study. Process Saf. Environ. Prot. 103, 23–35. doi:10.1016/j.psep.2016.06.014
Singh, N., and Gupta, S. K. (2016). Adsorption of Heavy Metals: a Review. Int. J. Innov. Res. Sci. Eng. Technol. 5 (2), 2267–2281.
Sitko, R., Turek, E., Zawisza, B., Malicka, E., Talik, E., Heimann, J., et al. (2013). Adsorption of Divalent Metal Ions from Aqueous Solutions Using Graphene Oxide. Dalton Trans. 42 (16), 5682–5689. doi:10.1039/c3dt33097d
Smith, S. C., and Rodrigues, D. F. (2015). Carbon-based Nanomaterials for Removal of Chemical and Biological Contaminants from Water: a Review of Mechanisms and Applications. Carbon 91, 122–143. doi:10.1016/j.carbon.2015.04.043
Sohi, S. P. (2012). Carbon Storage with Benefits. Science 338 (6110), 1034–1035. doi:10.1126/science.1225987
Spokas, K. A. (2010). Review of the Stability of Biochar in Soils: Predictability of O:C Molar Ratios. Carbon Manag. 1 (2), 289–303. doi:10.4155/cmt.10.32
Spokas, K. A., Koskinen, W. C., Baker, J. M., and Reicosky, D. C. (2009). Impacts of Woodchip Biochar Additions on Greenhouse Gas Production and Sorption/Degradation of two Herbicides in a Minnesota Soil. Chemosphere 77 (4), 574–581. doi:10.1016/j.chemosphere.2009.06.053
Sun, K., Jin, J., Keiluweit, M., Kleber, M., Wang, Z., Pan, Z., et al. (2012). Polar and Aliphatic Domains Regulate Sorption of Phthalic Acid Esters (PAEs) to Biochars. Bioresour. Technol. 118, 120–127. doi:10.1016/j.biortech.2012.05.008
Sun, K., Keiluweit, M., Kleber, M., Pan, Z., and Xing, B. (2011). Sorption of Fluorinated Herbicides to Plant Biomass-Derived Biochars as a Function of Molecular Structure. Bioresour. Technol. 102 (21), 9897–9903. doi:10.1016/j.biortech.2011.08.036
Taghizade Firozjaee, T., Mehrdadi, N., Baghdadi, M., and Nabi Bidhendi, G. R. (2018). Application of Nanotechnology in Pesticides Removal from Aqueous Solutions-A Review. Int. J. Nanosci. Nanotechnol. 14 (1), 43–56.
Tan, G., Sun, W., Xu, Y., Wang, H., and Xu, N. (2016). Sorption of Mercury (II) and Atrazine by Biochar, Modified Biochars and Biochar Based Activated Carbon in Aqueous Solution. Bioresour. Technol. 211, 727–735. doi:10.1016/j.biortech.2016.03.147
Tang, W. W., Zeng, G. M., Gong, J. L., Liu, Y., Wang, X. Y., and Liu, Y. Y. (2012). Simultaneous Adsorption of Atrazine and Cu(II) From Wastewater by Magnetic Multi-Walled Carbon Nanotube. Chem. Eng. J. 211, 470–478. doi:10.1016/j.cej.2012.09.102
Tao, Y., Han, S., Zhang, Q., Yang, Y., Shi, H., Akindolie, M. S., et al. (2020). Application of Biochar with Functional Microorganisms for Enhanced Atrazine Removal and Phosphorus Utilization. J. Clean. Prod. 257, 120535. doi:10.1016/j.jclepro.2020.120535
Tao, Y., Hu, S., Han, S., Shi, H., Yang, Y., Li, H., et al. (2019). Efficient Removal of Atrazine by Iron-Modified Biochar Loaded Acinetobacter Lwoffii DNS32. Sci. Total Environ. 682, 59–69. doi:10.1016/j.scitotenv.2019.05.134
Tripathi, A., and Ranjan, M. R. (2015). Heavy Metal Removal from Wastewater Using Low Cost Adsorbents. J. Bioremed Biodeg 6 (6), 315. doi:10.4172/2155-6199.1000315
Upadhyayula, V. K. K., Deng, S., Mitchell, M. C., and Smith, G. B. (2009). Application of Carbon Nanotube Technology for Removal of Contaminants in Drinking Water: a Review. Sci. total Environ. 408 (1), 1–13. doi:10.1016/j.scitotenv.2009.09.027
Vonberg, D., Vanderborght, J., Cremer, N., Pütz, T., Herbst, M., and Vereecken, H. (2014). 20 Years of Long-Term Atrazine Monitoring in a Shallow Aquifer in Western Germany. Water Res. 50, 294–306. doi:10.1016/j.watres.2013.10.032
Wang, G. S. (2005). Effects of Natural Organic Matter on Adsorption Capacity for Atrazine by Activated Carbon. J. Chin. Inst. Environ. Eng. 15, 81–89.
Wang, G. S. (2003). Temperature Dependence on Activated Carbon Adsorption of Atrazine. J. Chin. Inst. Environ. Eng. 13 (1), 45–53.
Wang, P., Liu, X., Yu, B., Wu, X., Xu, J., Dong, F., et al. (2020). Characterization of Peanut-Shell Biochar and the Mechanisms Underlying its Sorption for Atrazine and Nicosulfuron in Aqueous Solution. Sci. Total Environ. 702, 134767. doi:10.1016/j.scitotenv.2019.134767
Weber, J. B. (1993). Ionization and Sorption of Fomesafen and Atrazine by Soils and Soil Constituents. Pestic. Sci. 39 (1), 31–38. doi:10.1002/ps.2780390105
Wei, I. T. A., Pueh, L. L. L., Rosli, N. A., and Huang, L. T. (2013). Derivation of Oil Palm Shell-Based Adsorbent Using H2SO4 Treatment for Removal of Atrazine from Aqueous Solutions. Malays. J. Civ. Eng. 25 (1).
Wei, X., Wu, Z., Du, C., Wu, Z., Ye, B.-C., and Cravotto, G. (2017). Enhanced Adsorption of Atrazine on a Coal-Based Activated Carbon Modified with Sodium Dodecyl Benzene Sulfonate under Microwave Heating. J. Taiwan Inst. Chem. Eng. 77, 257–262. doi:10.1016/j.jtice.2017.04.004
Wei, X., Wu, Z., Wu, Z., and Ye, B.-C. (2018). Adsorption Behaviors of Atrazine and Cr(III) onto Different Activated Carbons in Single and Co-solute Systems. Powder Technol. 329, 207–216. doi:10.1016/j.powtec.2018.01.060
Welhouse, G. J., and Bleam, W. F. (1993). Atrazine Hydrogen-Bonding Potentials. Environ. Sci. Technol. 27 (3), 494–500. doi:10.1021/es00040a007
Weng, C.-H., Lin, Y.-T., and Tzeng, T.-W. (2009). Removal of Methylene Blue from Aqueous Solution by Adsorption onto Pineapple Leaf Powder. J. Hazard. Mater. 170 (1), 417–424. doi:10.1016/j.jhazmat.2009.04.080
Wu, S., He, H., Li, X., Yang, C., Zeng, G., Wu, B., et al. (2018). Insights into Atrazine Degradation by Persulfate Activation Using Composite of Nanoscale Zero-Valent Iron and Graphene: Performances and Mechanisms. Chem. Eng. J. 341, 126–136. doi:10.1016/j.cej.2018.01.136
Xue, Y., Gao, B., Yao, Y., Inyang, M., Zhang, M., Zimmerman, A. R., et al. (2012). Hydrogen Peroxide Modification Enhances the Ability of Biochar (Hydrochar) Produced from Hydrothermal Carbonization of Peanut Hull to Remove Aqueous Heavy Metals: Batch and Column Tests. Chem. Eng. J. 200-202, 673–680. doi:10.1016/j.cej.2012.06.116
Yacob, A. R., and Al Swaidan, H. M. (2012). Phosphoric Acid Effect on Prepared Activated Carbon from Saudi Arabia’s Date Frond Waste. Appl. Mech. Mater. 110, 2124–2130.
Yan, X. M., Shi, B. Y., Lu, J. J., Feng, C. H., Wang, D. S., and Tang, H. X. (2008). Adsorption and Desorption of Atrazine on Carbon Nanotubes. J. Colloid Interface Sci. 321 (1), 30–38. doi:10.1016/j.jcis.2008.01.047
Yang, F., Gao, Y., Sun, L., Zhang, S., Li, J., and Zhang, Y. (2018). Effective Sorption of Atrazine by Biochar Colloids and Residues Derived from Different Pyrolysis Temperatures. Environ. Sci. Pollut. Res. 25 (19), 18528–18539. doi:10.1007/s11356-018-2077-0
Yang, F., and Jiang, H. (2014). Amino Modification of Biochar for Enhanced Adsorption of Copper Ions From Synthetic Wastewater. Water Res. 48, 396–405. doi:10.1016/j.watres.2013.09.050
Yang, K., Wu, W., Jing, Q., and Zhu, L. (2008). Aqueous Adsorption of Aniline, Phenol, and Their Substitutes by Multi-Walled Carbon Nanotubes. Environ. Sci. Technol. 42 (21), 7931–7936. doi:10.1021/es801463v
Yang, K., Zhu, L., and Xing, B. (2006). Adsorption of Polycyclic Aromatic Hydrocarbons by Carbon Nanomaterials. Environ. Sci. Technol. 40 (6), 1855–1861. doi:10.1021/es052208w
Yang, Y., Shu, L., Wang, X., Xing, B., and Tao, S. (2011). Impact of De-ashing Humic Acid and Humin on Organic Matter Structural Properties and Sorption Mechanisms of Phenanthrene. Environ. Sci. Technol. 45 (9), 3996–4002. doi:10.1021/es2003149
Yunus, I. S., Harwin, , , Kurniawan, A., Adityawarman, D., and Indarto, A. (2012). Nanotechnologies in Water and Air Pollution Treatment. Environ. Technol. Rev. 1 (1), 136–148. doi:10.1080/21622515.2012.733966
Zadaka, D., Nir, S., Radian, A., and Mishael, Y. G. (2009). Atrazine Removal from Water by Polycation-Clay Composites: Effect of Dissolved Organic Matter and Comparison to Activated Carbon. Water Res. 43 (3), 677–683. doi:10.1016/j.watres.2008.10.050
Zhang, L., Zhang, Y., Xiao, K., Shi, J., Du, X., Wang, L., et al. (2021). The Intimate Coupling of Photocatalysis and Biodegradation for the Degradation and Mineralization of Atrazine in Water. New J. Chem. 45 (29), 13029–13039. doi:10.1039/d1nj00134e
Zhang, P., Sun, H., Yu, L., and Sun, T. (2013). Adsorption and Catalytic Hydrolysis of Carbaryl and Atrazine on Pig Manure-Derived Biochars: Impact of Structural Properties of Biochars. J. Hazard. Mater. 244-245, 217–224. doi:10.1016/j.jhazmat.2012.11.046
Zhang, S., Shao, T., Kose, H. S., and Karanfil, T. (2010). Adsorption of Aromatic Compounds by Carbonaceous Adsorbents: a Comparative Study on Granular Activated Carbon, Activated Carbon Fiber, and Carbon Nanotubes. Environ. Sci. Technol. 44 (16), 6377–6383. doi:10.1021/es100874y
Zhang, W., Wang, L., and Sun, H. (2011). Modifications of Black Carbons and Their Influence on Pyrene Sorption. Chemosphere 85 (8), 1306–1311. doi:10.1016/j.chemosphere.2011.07.042
Zhang, W., Zheng, J., Zheng, P., and Qiu, R. (2015). Atrazine Immobilization on Sludge Derived Biochar and the Interactive Influence of Coexisting Pb(II) or Cr(VI) Ions. Chemosphere 134, 438–445. doi:10.1016/j.chemosphere.2015.05.011
Zhang, Y., Cao, B., Zhao, L., Sun, L., Gao, Y., Li, J., et al. (2018). Biochar-supported Reduced Graphene Oxide Composite for Adsorption and Coadsorption of Atrazine and Lead Ions. Appl. Surf. Sci. 427, 147–155. doi:10.1016/j.apsusc.2017.07.237
Zhao, J., Liu, L., and Li, F. (2015). Graphene Oxide: Physics and Applications. London, UK: Springer, 161.
Zhao, L., Yang, F., Jiang, Q., Zhu, M., Jiang, Z., Tang, Y., et al. (2018). Characterization of Modified Biochars Prepared at Low Pyrolysis Temperature as an Efficient Adsorbent for Atrazine Removal. Environ. Sci. Pollut. Res. 25 (2), 1405–1417. doi:10.1007/s11356-017-0492-2
Zhao, X., Ouyang, W., Hao, F., Lin, C., Wang, F., Han, S., et al. (2013). Properties Comparison of Biochars from Corn Straw with Different Pretreatment and Sorption Behaviour of Atrazine. Bioresour. Technol. 147, 338–344. doi:10.1016/j.biortech.2013.08.042
Zhao, N., Zhao, C., Lv, Y., Zhang, W., Du, Y., Hao, Z., et al. (2017). Adsorption and Coadsorption Mechanisms of Cr (VI) and Organic Contaminants on H3PO4 Treated Biochar. Chemosphere 186, 422–429. doi:10.1016/j.chemosphere.2017.08.016
Zheng, H., Wang, Z., Zhao, J., Herbert, S., and Xing, B. (2013). Sorption of Antibiotic Sulfamethoxazole Varies with Biochars Produced at Different Temperatures. Environ. Pollut. 181, 60–67. doi:10.1016/j.envpol.2013.05.056
Zhu, D., Kwon, S., and Pignatello, J. J. (2005). Adsorption of Single-Ring Organic Compounds to Wood Charcoals Prepared under Different Thermochemical Conditions. Environ. Sci. Technol. 39 (11), 3990–3998. doi:10.1021/es050129e
Zhu, D., and Pignatello, J. J. (2005). Characterization of Aromatic Compound Sorptive Interactions with Black Carbon (Charcoal) Assisted by Graphite as a Model. Environ. Sci. Technol. 39 (7), 2033–2041. doi:10.1021/es0491376
Keywords: metabolites, mechanism, herbicide, biochar, graphene
Citation: Ahmad M, Riaz U, Iqbal S, Ahmad J, Rasheed H, Al-Farraj ASF and Al-Wabel MI (2022) Adsorptive Removal of Atrazine From Contaminated Water Using Low-Cost Carbonaceous Materials: A Review. Front. Mater. 9:909534. doi: 10.3389/fmats.2022.909534
Received: 31 March 2022; Accepted: 18 May 2022;
Published: 27 June 2022.
Edited by:
Mohamed S. Mohy Eldin, City of Scientific Research and Technological Applications, EgyptReviewed by:
Mohammad Boshir Ahmed, Gwangju Institute of Science and Technology, South KoreaCopyright © 2022 Ahmad, Riaz, Iqbal, Ahmad, Rasheed, Al-Farraj and Al-Wabel. This is an open-access article distributed under the terms of the Creative Commons Attribution License (CC BY). The use, distribution or reproduction in other forums is permitted, provided the original author(s) and the copyright owner(s) are credited and that the original publication in this journal is cited, in accordance with accepted academic practice. No use, distribution or reproduction is permitted which does not comply with these terms.
*Correspondence: Munir Ahmad, YW11bmlyQGtzdS5lZHUuc2E=
Disclaimer: All claims expressed in this article are solely those of the authors and do not necessarily represent those of their affiliated organizations, or those of the publisher, the editors and the reviewers. Any product that may be evaluated in this article or claim that may be made by its manufacturer is not guaranteed or endorsed by the publisher.
Research integrity at Frontiers
Learn more about the work of our research integrity team to safeguard the quality of each article we publish.