- 1State Key Laboratory of Electronic Thin Films and Integrated Devices, University of Electronic Science and Technology of China, Chengdu, China
- 2Key Laboratory of Spintronics Materials, Devices and Systems of Zhejiang Province, Hangzhou, China
With the development of spintronics, garnet films with perpendicular magnetic anisotropy (PMA) have been attracting the attention of researchers for decades. In this work, bismuth-doped thulium iron garnet (Tm2BiFe5O12, TmBiIG) films of varying thickness having strong PMA effect were fabricated on substituted Gd3Ga5O12 (sGGG) (111) substrates using the pulsed laser deposition (PLD) technique. Crystallographic characterization and magnetic properties of TmBiIG films were investigated using high-resolution scanning transmission electron microscopy, X-ray diffraction, vibrating sample magnetometry, and broadband ferromagnetic resonance (FMR). A high perpendicular anisotropic field of H⊥ = 4,445 ± 7.5 Oe in a 10-nm-thick film and H⊥ = 4,582 ± 7.7 Oe in a 30-nm-thick film at room temperature were obtained and analyzed in detail. Surprisingly, an additional spin-wave mode was observed in the in-plane FMR spectra. The discrepancy between in-plane and the out-of-plane Landé g-factors established a correlation with the PMA effect in the TmBiIG films. The Landé g-factor of the TmBiIG films is much lower than that of free electrons, indicating that the strong spin–orbit coupling is caused by Tm and Bi heavy elements. The Gilbert damping factor α changed from 0.007 to 0.012 in various thicknesses of TmBiIG films.
Introduction
Spintronics based on magnetic garnets is a potential choice for data storage, logical computation, and next-generation sensing. Ferrimagnetic garnets, such as yttrium iron garnet (Sokolov et al., 2016) (Y3Fe5O12, YIG) or thulium iron garnet (Tm3Fe5O12, TmIG) have been extensively studied for spin transport and spin interactions, such as inverse spin Hall effect (Li et al., 2020) (ISHE), spin Seebeck effect (Kikkawa et al., 2013) (SSE), spin–orbit torque (Avci et al., 2017) (SOT) switching, and topological hall effect (Ahmed et al., 2019; Shao et al., 2019), due to their small Gilbert damping factor (10−4–10−2), narrow ferromagnetic resonance linewidth, and perpendicular magnetic anisotropy (PMA) effect. Recently, Avci et al. (2017) developed new devices for regulating SOTs in a TmIG/Pt heterostructure grown on (111)-oriented gadolinium gallium garnet (Gd3Ga5O12, GGG) with the PMA effect. This observation may lead to a new direction of research that can reduce the energy consumption in integrated devices and can demonstrate the importance of investigating new garnets having a reliable and powerful PMA effect.
The method for obtaining a strong PMA effect in garnet films is to introduce a large uniaxial anisotropy,
In this work, bismuth-doped thulium iron garnet (Tm2BiFe5O12, TmBiIG) films of varying thicknesses with a strong PMA effect were fabricated on 5 mm × 5 mm × 0.5 mm sGGG (111) substrates using the pulsed laser deposition (PLD) technique. The value of the perpendicular anisotropic field in different film thicknesses was determined by ferromagnetic resonance measurements. Due to the strong PMA effect of the film (Farle, 1998), an extra spin-wave mode was observed in the in-plane magnetized condition when applied magnetic field H is smaller than the net perpendicular anisotropy field
Materials and Methods
In our experiments, a 2-inch Tm2BiFe5O12 ceramic target with high purity was fabricated by a standard solid state reaction method of Tm2O3 (99.999%), Bi2O3 (99.999%), and Fe2O3 (99.999%). Before the growth, a mixture of concentrated 10 ml sulfuric acid and 10 ml hydrogen peroxide was prepared, and the sGGG substrate was immersed for 15 min to remove the oxide impurities on the surface. Then sGGG substrate was soaked in acetone and alcohol, respectively, for ultrasonic cleaning to obtain a clean substrate surface. Before depositing the films, the distance between the target and the substrate was adjusted to 70 mm. The wavelength of the KrF excimer laser used in PLD was 248 nm. Laser intensity and frequency were set to 300 mJ (2.4 J/cm2) and 5 Hz, respectively. The base vacuum level of the chamber was 8 × 10−6 Pa. During the pre-deposition process, the temperature in the chamber was raised from room temperature to 750°C at a rate of about 12°C/min. At the same time, the oxygen pressure was set to 1 Pa in order to form a high-quality TmBiIG film. Under the aforementioned conditions, the growth rate of the film was about 2 nm/min. After the deposition, the films were transferred to a thermal annealing system for recrystallization at 800°C in air atmosphere for 1 h, and the heating rates and cooling rates are 60°C/min and 4.33°C/min, respectively.
TmBiIG films were characterized by a high-resolution X-ray diffractometer (HRXRD, Bede D1), high-resolution scanning transmission electron microscopy (STEM, FEI Talos F200X), atomic force microscope (AFM, PARK-XE7), vibrating sample magnetometry (VSM, USA LakeShore 8,603), and angular-resolved FMR measurements. The FMR spectrum was obtained by sweeping the magnetic field at a fixed microwave frequency. The derivative of microwave absorption power and the static magnetic field was defined as the intensity of the FMR spectrum, which was attained by applying a static magnetic field in-plane and out-of-plane. Coplanar waveguide (CPW) and short circuit antenna structures were composed of 20 nm Ta and 200 nm Au manufactured by photolithography. All the measurements were conducted at room temperature.
Results and Discussion
The surface morphology characterization of a 20-nm-thick TmBiIG film sample measured in the contact mode is shown in Figure 1A. The root-mean-square (rms) roughness of the film over a 2 μm × 2 µm scanned area is 0.3 nm, which indicates a smooth film surface. All samples in this work show a similar rms roughness. The in-plane XRD result along (20-2) of a 30-nm-thick film is shown in Figure 1B, the position of the diffraction peak corresponds to that in the study by Wu et al. (2018). No discernible separation of the peaks of TmBiIG films and sGGG substrates was observed, which means the sample grew coherently on the substrate through the strain. Peaks from thinner samples could not be resolved using XRD. However, considering the thickest sample in the study grow coherently on the substrate and the film of each thickness used in this work exhibits the PMA effect, all thickness samples are supposed to show a similar in-plane XRD pattern. Figure 1C displays a series of HRXRD patterns for the TmBiIG films grown on the (111) sGGG substrates at various thicknesses. The radiation line of the HRXRD is Cu-Kα. A bare substrate diffraction pattern is performed for comparison, revealing the contributions from Kα2 and Kα1 (Illig et al., 2013), which split the main (444) reflection of the substrates. According to the XRD patterns, the thickness of the TmBiIG has an obvious effect on the crystalline structure. With the decrease in the film thickness, the (444) diffraction peak of TmBiIG at an angle of 2θ becomes larger, indicating a more compressed lattice in the direction of growth, which results in a smaller lattice constant in the film’s normal direction. Through Bragg’s laws, the lattice spacing was deduced from the XRD results, and then the lattice parameter was calculated. The out-of-plane strain states
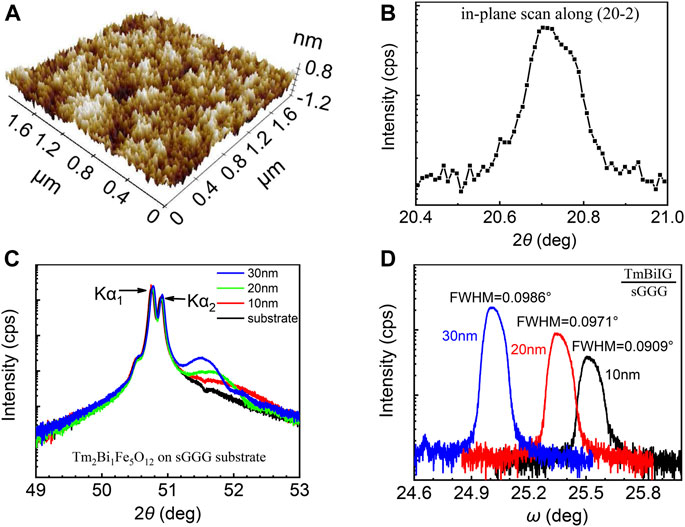
FIGURE 1. (A) AFM surface morphology of a 20-nm-thick TmBiIG film in a 2 μm × 2 µm region with rms roughness of 0.3 nm, demonstrating the ultra-flat surface. Crystallographic characterization results for the TmBiIG films; (B) HRXRD in-plane scan of the 30-nm-thick sample along the (20-2) direction; (C) HRXRD ω–2θ scans of the (444) plane of the TmBiIG/sGGG (111), and (D) rocking curve of the TmBiIG (444) for various film thicknesses.
Figure 2 shows a high angle annular dark field scanning transmission electron microscopy (HAADF-STEM) image of the 20-nm-thick sample. The line of dashes in Figure 2 reveals the boundary between the TmBiIG film and the sGGG substrate, identified by an energy dispersive spectrometer (EDS) surface scan. Tm, Bi, and Fe elements in the film and Gd, Ga, and Ca elements in the substrate were verified, exhibiting the slight diffusion phenomenon of the above five atoms at both ends of the interface. Figure 3 demonstrates an image of a high-resolution transmission electron microscope (HR-TEM) of the cross section from the 20-nm-thick TmBiIG film grown on sGGG, and a periodic lattice is clearly observed illustrating the good crystalline quality of the film. The well-ordered atomic structure and sharp diffraction spots illustrate good epitaxial growth of TmBiIG (111) on sGGG (111), and there is no visible dislocation or cracking in the film, even due to the in-plane strain.
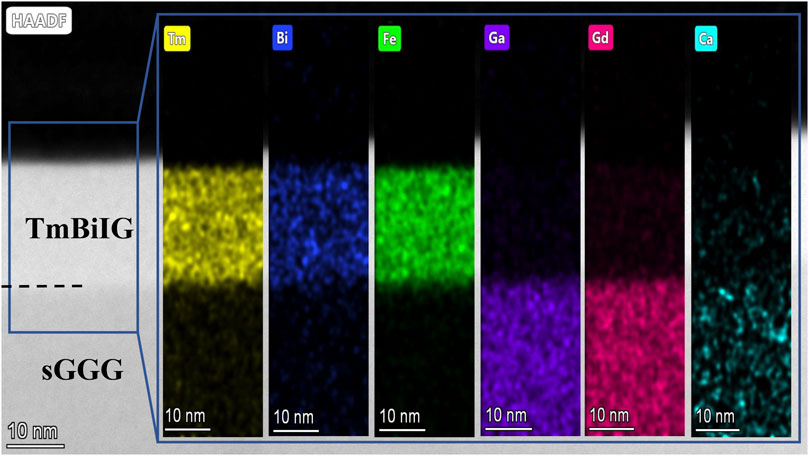
FIGURE 2. HAADF-STEM image of the 20-nm-thick TmBiIG/sGGG cross-sectional structure. The black line of dashes shows the boundary between film and substrate. EDS exhibits the location distribution of the Tm, Bi, Fe, Ga, Gd, and Ca elements by comparing different colors.
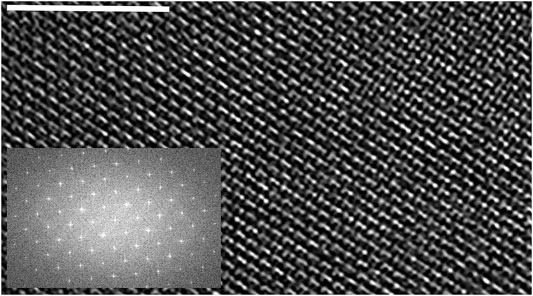
FIGURE 3. HR-TEM image from a 20-nm-thick TmBiIG/sGGG cross-sectional structure. The white scale bar corresponds to 5 nm. Inset shows the FFT from the image.
In the existing works (Kubota et al., 2013; Zanjani and Onbasli, 2019), the film will be subjected to tensile strain when the film lattice constant
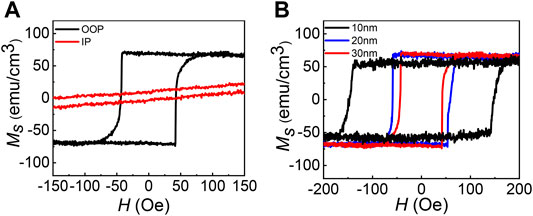
FIGURE 4. (A) Out-of-plane (black line) and in-plane (red line) hysteresis loops of a 30-nm-thick sample in narrow range. (B) Out-of-plane hysteresis loop of 10-, 20-, and 30-nm-thick films in the narrow range. The paramagnetic contribution from the substrate sGGG has been removed in the raw data.
In the TmBiIG/sGGG system, the main contributors to the effective anisotropy energy density are shape anisotropy
Eq. 2 displays the free energy of the TmBiIG film:
where (θ, φ) and (
When θ = θH = 90°,
Moreover,
where
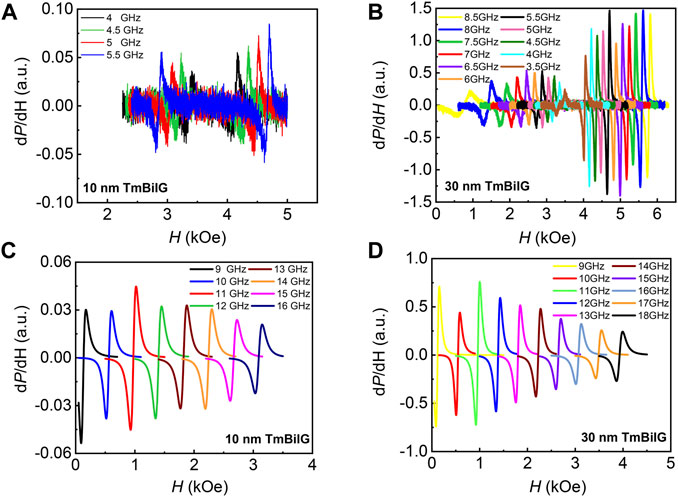
FIGURE 5. FMR measurement of the TmBiIG film. In-plane ferromagnetic resonance spectra of (A) 10- and (B) 30-nm-thick samples. Out-of-plane ferromagnetic resonance spectra of (C) 10 nm thickness in 9–16 GHz (1 GHz interval) and (D) 30 nm thickness in 9–18 GHz (1 GHz interval).
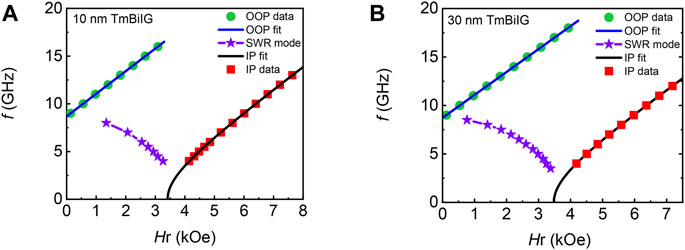
FIGURE 6. Resonance magnetic field dependence on the frequency in (A) 10- and (B) 30-nm-thick films. An extra spin-wave mode (SWR) was detected in the in-plane ferromagnetic resonance spectra.
However, the experimental results show that the perpendicular anisotropic field H⊥ of the 10-nm-thick film is slightly smaller than that of the 30-nm-thick film. This is due to the 4πMS in the 10-nm-thick film being smaller than that in the 30-nm-thick film, which is shown in Figure 4B.
The comparison of the H⊥ value between the TmBiIG/sGGG system and other ferrimagnetic garnet systems are shown in Table 1. It should be noted that the H⊥ value in the TmBiIG/sGGG system is larger than that in the TmIG/sGGG system when the film has the same thickness (10 nm). This is due to the extra growth-induced anisotropy introduced by Bi doping. As a heavy element with strong spin–orbit interaction, Bi3+ ions have a profound influence on the local symmetry, super-exchange interaction, and spin–orbit coupling in the nearby Fe3+ ions. The order of priority for the Bi3+ ions in the dodecahedron position affects the uniaxial component of free energy of the adjacent Fe3+ ions in the octahedron and the tetrahedron positions, thus resulting in an additional magnetic anisotropy (Hansen and Witter, 1985).

TABLE 1. Comparison of magnetic anisotropy parameters based on the recent works. Partial reference data have been converted from the SI units to the CGS units in the article. It should be pointed out that the sGGG* listed in the table has a larger lattice constant than the substrate used in this work.
As shown in Figures 5A,B, a special spin-wave mode exists at low frequencies and for low magnetic fields in all thickness films, which is also observed in other materials due to the strong perpendicular anisotropy (Barsukov et al., 2014; He et al., 2016; Glowinski et al., 2017; Devolder et al., 2018). This special spin mode was observed using the in-plane FMR measurements and is usually known as the smallest spin mode in bubble films (Portis, 1963; Dötsch et al., 1978). Unlike the Kittel mode, the magnitude of the resonance field is inversely related to the resonance frequency as this spin-wave mode occurs when the magnetic moment is not fully oriented in the in-plane, indicating that the magnetic moments are not processed uniformly (Hoekstra et al., 1977; Dötsch et al., 1978).
From the fitting analysis in Eqs 3, 4, the gyromagnetic ratio of the in-plane and the out-of-plane magnetized of various thicknesses of the TmBiIG film is obtained. The values of
where

TABLE 2. Gyromagnetic ratio and Landé g-factor under in-plane and out-of-plane magnetized condition of the TmBiIG film with various thicknesses.
Based on the Bruno theory (Bruno, 1989) and the Justin M. Shaw experiments (Shaw et al., 2013), when the Curie temperature (
where ξ is the spin–orbit coupling parameter, A is a pre-factor which is a function of the electronic structure, and
Gilbert damping factor α and the FMR linewidth
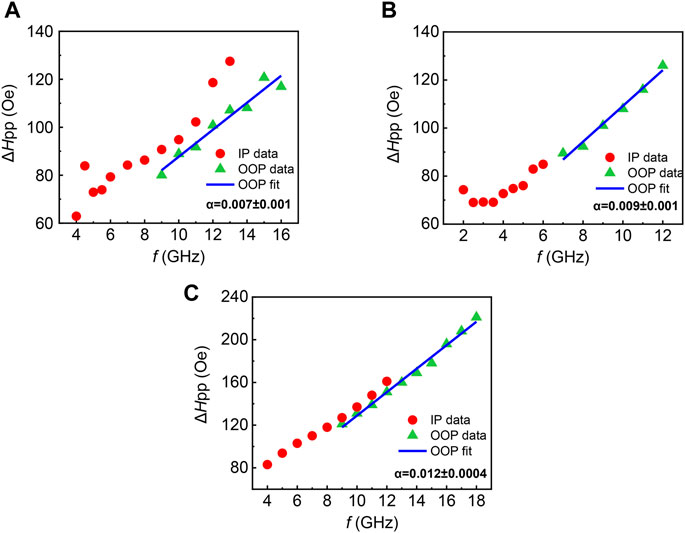
FIGURE 7. Relationship between the frequency and the FMR linewidth in (A) 10-, (B) 20-, and (C) 30-nm-thick samples under in-plane and out-of-plane magnetized condition. The Gilbert damping factor in each thickness film and its error bar are shown in the figure, respectively.
The values of Gilbert damping α in 10-, 20-, and 30-nm-thick TmBiIG films are 0.007 ± 0.001, 0.009 ± 0.001, and 0.012 ± 0.004, demonstrating that the TmBiIG/sGGG system is of the same order as that of the TmIG/sGGG system, which has been recently reported to be in the range of 0.015–0.025 (Ciubotariu et al., 2019), suggesting that the doping of Bi element has no significant influence on α. In addition, no clear thickness dependence of the damping constant was observed.
Conclusion
In summary, in this work, ultrathin TmBiIG films with strong PMA were fabricated on [111]-oriented sGGG substrate using pulsed laser deposition. The perpendicular anisotropic field of TmBiIG films with different thicknesses was determined by ferromagnetic resonance measurements. The anisotropic gyromagnetic ratio and the anisotropic g-factor are extracted from the in-plane and the out-of-plane FMR measurements, respectively. The appearance of the spin-wave mode in-plane is also related to the strong perpendicular anisotropy. The outstanding PMA effect and the relatively small Gilbert damping (α) indicate the potential application of the ultrathin TmBiIG films in the field of spintronic devices.
Data Availability Statement
The original contributions presented in the study are included in the article/Supplementary Material, further inquiries can be directed to the corresponding authors.
Author Contributions
XZ performed sample preparation and wrote the manuscript; LJ designed and supervised this work; DZ and LZ performed FMR measurements; XT and ZZ took part in data analysis; HM and BL provided editing and writing assistance. All authors read and contributed to the manuscript.
Funding
This work was supported by the National Key Research and Development Plan under Grant No. 2016YFA0300801, National Key Scientific Instrument and Equipment Development Project No. 51827802, the National Natural Science Foundation of China under Grant No. 62171096, and the Sichuan Science and Technology Support Project under Grant Nos. 2021YFG0347 and 2021YFG0091.
Conflict of Interest
The authors declare that the research was conducted in the absence of any commercial or financial relationships that could be construed as a potential conflict of interest.
Publisher’s Note
All claims expressed in this article are solely those of the authors and do not necessarily represent those of their affiliated organizations, or those of the publisher, the editors, and the reviewers. Any product that may be evaluated in this article, or claim that may be made by its manufacturer, is not guaranteed or endorsed by the publisher.
References
Ahmed, A. S., Lee, A. J., Bagues, N., McCullian, B. A., Thabt, A. M. A., Perrine, A., et al. (2019). Spin-Hall Topological Hall Effect in Highly Tunable Pt/Ferrimagnetic-Insulator Bilayers. Nano Lett. 19, 2265. doi:10.1021/acs.nanolett.9b02265
Avci, C. O., Quindeau, A., Pai, C.-F., Mann, M., Caretta, L., Tang, A. S., et al. (2017). Beach, Current-Induced Switching in a Magnetic Insulator. Nat. Mater. 16, 309. doi:10.1038/nmat4812
Barsukov, I., Fu, Y., Gonçalves, A. M., Spasova, M., Farle, M., Sampaio, L. C., et al. (2014). Field-dependent Perpendicular Magnetic Anisotropy in CoFeB Thin Films. Appl. Phys. Lett. 105, 152403. doi:10.1063/1.4897939
Bruno, P. (1989). Tight-binding Approach to the Orbital Magnetic Moment and Magnetocrystalline Anisotropy of Transition-Metal Monolayers. Phys. Rev. B: Condens. Matter Mater. Phys. 39, 865. doi:10.1103/physrevb.39.865
Ciubotariu, O., Semisalova, A., Lenz, K., and Albrecht, M. (2019). Strain-induced Perpendicular Magnetic Anisotropy and Gilbert Damping of Tm3Fe5O12 Thin Films. Sci. Rep. 9, 17474. doi:10.1038/s41598-019-53255-6
Crossley, S., Quindeau, A., Swartz, A. G., Rosenberg, E. R., Beran, L., Avci, C. O., et al. (2019). Ferromagnetic Resonance of Perpendicularly Magnetized Tm3Fe5O12/Pt Heterostructures. Appl. Phys. Lett. 115, 172402. doi:10.1063/1.5124120
Devolder, T., Couet, S., Swerts, J., and Kar, G. S. (2018). Gilbert Damping of High Anisotropy Co/Pt Multilayers. J. Phys. D: Appl. Phys. 51, 135002. doi:10.1088/1361-6463/aaaf30
Dötsch, H., Röschmann, P., and Schilz, W. (1978). Ferrimagnetic Resonance Spectra of Magnetic Bubble Films at Low Microwave Frequencies. Appl. Phys. A: Mater. Sci. Process. 15, 167.
Farle, M. (1998). Ferromagnetic Resonance of Ultrathin Metallic Layers. Rep. Prog. Phys. 61, 755. doi:10.1088/0034-4885/61/7/001
Fu, J., Hua, M., Wen, X., Xue, M., Ding, S., Wang, M., et al. (2017). Epitaxial Growth of Y3Fe5O12 Thin Films with Perpendicular Magnetic Anisotropy. Appl. Phys. Lett. 110. doi:10.1063/1.4983783
Glowinski, H., Zywczak, A., Wrona, J., Krysztofik, A., Goscianska, I., Stobiecki, T., et al. (2017). CoFeB/MgO/CoFeB Structures with Orthogonal Easy Axes: Perpendicular Anisotropy and Damping. J. Phys. Condens. Matter 29, 485803.
Hansen, P., and Krumme, J. P. (1984). Magnetic and Magneto-Optical Properties of Garnet Films. Thin Solid Films 114, 69. doi:10.1016/0040-6090(84)90337-7
Hansen, P., and Witter, K. (1985). Growth-induced Uniaxial Anisotropy of Bismuth-Substituted Iron-Garnet Films. J. Appl. Phys. 58, 454. doi:10.1063/1.335645
Hansen, P., Witter, K., and Tolksdorf, W. (1983). Magnetic and Magneto-Optic Properties of lead- and Bismuth-Substituted Yttrium Iron Garnet Films. Phys. Rev. B 27, 6608. doi:10.1103/physrevb.27.6608
He, C., Navabi, A., Shao, Q., Yu, G., Wu, D., Zhu, W., et al. (2016). Spin-torque Ferromagnetic Resonance Measurements Utilizing Spin Hall Magnetoresistance in W/Co40Fe40B20/MgO Structures. Appl. Phys. Lett. 109, 202404. doi:10.1063/1.4967843
Hoekstra, B., van Stapele, R. P., and Robertson, J. M. (1977). Spin‐wave Resonance Spectra of Inhomogeneous Bubble Garnet Films. J. Appl. Phys. 48, 382. doi:10.1063/1.323339
Illig, A. J., Chantler, C. T., and Payne, A. T. (2013). Voigt Profile Characterization of copperKα. J. Phys. B: Mol. Opt. Phys. 46, 235001. doi:10.1088/0953-4075/46/23/235001
Khodadadi, B., Rai, A., Sapkota, A., Srivastava, A., Nepal, B., Lim, Y., et al. (2020). Conductivitylike Gilbert Damping Due to Intraband Scattering in Epitaxial Iron. Phys. Rev. Lett. 124, 157201. doi:10.1103/physrevlett.124.157201
Kikkawa, T., Uchida, K., Shiomi, Y., Qiu, Z., Hou, D., Tian, D., et al. (2013). Longitudinal Spin Seebeck Effect Free from the Proximity Nernst Effect. Phys. Rev. Lett. 110, 067207. doi:10.1103/PhysRevLett.110.067207
Kubota, M., Shibuya, K., Tokunaga, Y., Kagawa, F., Tsukazaki, A., Tokura, Y., et al. (2013). Systematic Control of Stress-Induced Anisotropy in Pseudomorphic Iron Garnet Thin Films. J. Magn. Magn. Mater. 339, 63. doi:10.1016/j.jmmm.2013.02.045
Li, M., Jin, L., Zhong, Z., Tang, X., Yang, Q., Zhang, L., et al. (2020). Impact of Interfacial Chemical State on Spin Pumping and Inverse Spin Hall Effect in YIG/Pt Hybrids. Phys. Rev. B 102, 174435. doi:10.1103/physrevb.102.174435
Lin, Y., Jin, L., Zhang, H., Zhong, Z., Yang, Q., Rao, Y., et al. (2020). Bi-YIG Ferrimagnetic Insulator Nanometer Films with Large Perpendicular Magnetic Anisotropy and Narrow Ferromagnetic Resonance Linewidth. J. Magn. Magn. Mater. 496, 165886. doi:10.1016/j.jmmm.2019.165886
Portis, A. M. (1963). Low-Lying Spin Wave Modes in Ferromagnetic Films. Appl. Phys. Lett. 2, 69. doi:10.1063/1.1753779
Shao, Q., Liu, Y., Yu, G., Kim, S. K., Che, X., Tang, C., et al. (2019). Topological Hall Effect at above Room Temperature in Heterostructures Composed of a Magnetic Insulator and a Heavy Metal. Nat. Electron. 2, 182. doi:10.1038/s41928-019-0246-x
Shaw, J. M., Nembach, H. T., and Silva, T. J. (2013). Measurement of Orbital Asymmetry and Strain in Co90Fe10/Ni Multilayers and Alloys: Origins of Perpendicular Anisotropy. Phys. Rev. B 87, 054416. doi:10.1103/physrevb.87.054416
Shaw, J. M., Nembach, H. T., Weiler, M., Silva, T. J., Schoen, M., Sun, J. Z., et al. (2015). Perpendicular Magnetic Anisotropy and Easy Cone State in Ta/Co60Fe20B20/MgO. IEEE Magn. Lett. 6, 1. doi:10.1109/lmag.2015.2438773
Sokolov, N. S., Fedorov, V. V., Korovin, A. M., Suturin, S. M., Baranov, D. A., Gastev, S. V., et al. (2016). Thin Yttrium Iron Garnet Films Grown by Pulsed Laser Deposition: Crystal Structure, Static, and Dynamic Magnetic Properties. J. Appl. Phys. 119, 023903. doi:10.1063/1.4939678
Soumah, L., Beaulieu, N., Qassym, L., Carretero, C., Jacquet, E., Lebourgeois, R., et al. (2018). Ultra-low Damping Insulating Magnetic Thin Films Get Perpendicular. Nat. Commun. 9, 3355. doi:10.1038/s41467-018-05732-1
Tang, C., Sellappan, P., Liu, Y., Xu, Y., Garay, J. E., and Shi, J. (2016). Anomalous Hall Hysteresis in Tm3Fe5O12/Ptwith Strain-Induced Perpendicular Magnetic Anisotropy. Phys. Rev. B 94, 140403. doi:10.1103/physrevb.94.140403
Tivakornsasithorn, K., Liu, X., Li, X., Dobrowolska, M., and Furdyna, J. K. (2014). Magnetic Anisotropy in Ultrathin Fe Films on GaAs, ZnSe, and Ge (001) Substrates. J. Appl. Phys. 116, 043915. doi:10.1063/1.4891253
Vilela, G., Chi, H., Stephen, G., Settens, C., Zhou, P., Ou, Y., et al. (2020). Strain-tuned Magnetic Anisotropy in Sputtered Thulium Iron Garnet Ultrathin Films and TIG/Au/TIG Valve Structures. J. Appl. Phys. 127, 115302. doi:10.1063/1.5135012
Woltersdorf, G., Kiessling, M., Meyer, G., Thiele, J. U., and Back, C. H. (2009). Damping by Slow Relaxing Rare Earth Impurities in Ni80Fe20. Phys. Rev. Lett. 102, 257602. doi:10.1103/physrevlett.102.257602
Wu, C. N., Tseng, C. C., Lin, K. Y., Cheng, C. K., Yeh, S. L., Fanchiang, Y. T., et al. (2018). High-quality Single-crystal Thulium Iron Garnet Films with Perpendicular Magnetic Anisotropy by off-axis Sputtering. AIP Adv. 8, 055904. doi:10.1063/1.5006673
Keywords: bismuth-doped thulium iron garnet, perpendicular magnetic anisotropy, spintronic materials, Landé g-factor, ferromagnetic resonance spectrum
Citation: Zhang X, Jin L, Zhang D, Liu B, Meng H, Zhang L, Zhong Z and Tang X (2022) Strong Perpendicular Anisotropy and Anisotropic Landé Factor in Bismuth-Doped Thulium Garnet Thin Films. Front. Mater. 9:879711. doi: 10.3389/fmats.2022.879711
Received: 20 February 2022; Accepted: 28 March 2022;
Published: 28 April 2022.
Edited by:
Jijie Huang, Sun Yat-sen University, ChinaReviewed by:
Yanda Ji, Nanjing University of Aeronautics and Astronautics, ChinaDi Zhang, Los Alamos National Laboratory (DOE), United States
Copyright © 2022 Zhang, Jin, Zhang, Liu, Meng, Zhang, Zhong and Tang. This is an open-access article distributed under the terms of the Creative Commons Attribution License (CC BY). The use, distribution or reproduction in other forums is permitted, provided the original author(s) and the copyright owner(s) are credited and that the original publication in this journal is cited, in accordance with accepted academic practice. No use, distribution or reproduction is permitted which does not comply with these terms.
*Correspondence: Lichuan Jin, bGljaHVhbmpAdWVzdGMuZWR1LmNu; Bo Liu, bGl1Ym9fc21AMTYzLmNvbQ==