- Advanced Materials Design and Processing Group, Department of Metallurgical and Materials Engineering, Indian Institute of Technology Jodhpur, Jodhpur, India
Sudden damage in engineering materials during service was always a challenge, and a lot of efforts have been put forward by materials scientists to resolve this issue. This motivated the design of a variety of classes of alloys such as stainless steel, deformed and partitioned steels, metastable Ti alloys, and recently developed high entropy alloys (HEA). A continuous evolving journey from single-phase to multi-phase HEAs showed exceptional work hardenability in them, which is required for improved failure resistance in metallic systems. In line with that, recently developed transformative high entropy alloys (T-HEAs) displayed interesting outcomes in terms of improved damage tolerance (σUTS ∼1.2 GPa and ductility ∼20% with 1.5% defect density) after conventional processing, severe deformation, and laser-assisted 3D printing. These outcomes were attributed to the localized WH activity within the stress concentration regions due to the activation of transformation induced plasticity (TRIP) effect near the defects. As a result, defects present in these T-HEAs act as sites for delaying the damage by showing a pronounced transformation induced crack retardation (TRICR) effect, thereby improving failure resistance during service.
Introduction
The continuous evolution of human life demands the development of newer and advanced materials for overcoming environmental and economic concerns. However, most of these metallic materials exhibit a limitation of strength-ductility tradeoff. This inverse strength-ductility relation is a major challenge in most of the day-to-day materials such as steels, Al alloys, and Ti alloys which are getting used for applications leading to sudden failures during service (Larke 1935, Kim et al., 2013; Wang et al., 1995). The easiest way to tackle this issue is via increasing the work hardenability of the material at room temperature to balance strength and ductility simultaneously. This attempt gave birth to very advanced materials for the human community, such as advanced high strength steels, twinning (TWIP)/transformation induced plasticity (TRIP) assisted steels, and ultralight Mg-Li-Ca alloys, which displayed activation of multiple deformation mechanisms during straining to increase the work hardening (WH) ability (Larke 1935, Kim et al., 2013; Wang et al., 1995, Estrin et al., 2016).
The design of single-phase high entropy alloys (HEAs) was a breakthrough in showing excessive WH in the stress-strain response owing to the dominance of solid solution strengthening during deformation. Conventional materials failed to attain these unconventional properties; hence, the concept of the equiatomic addition of elements (HEA design) in an alloy system has attracted researchers in a very short time span. Apart from suppressing the formation of intermetallic formation, HEA design realized the evolution of disordered solid solutions (DSS) (Yeh et al., 2004; Miracle and Senkov, 2017). It was found that the phase stability of these DSS can be tuned very easily by minor variations of constituent elements, and hence WH ability can be tailored further. In line with that development of multi-phase HEAs (types of HEAs such as FeMnCoCrNi based equiatomic and non-equiatomic HEAs and HEAs based on AlxCoCrFeNi and FeMnCoCr systems) was proposed in which the phases formed are either disordered solid solutions or disordered intermetallic compounds. (Yeh et al., 2004; Miracle and Senkov, 2017). The design of dual-phase non-equiatomic HEAs is a turning point toward engineering the WH ability of metallic materials due to the synergistic activation of dislocation, transformation and twinning effects during deformation (Li et al., 2016; Nene et al., 2020). An additional benefit of non-equiatomic HEA design was to devise a huge compositional space (away from the center of the phase diagram) for developing new alloys that were previously unexplored (Yeh et al., 2004; Li et al., 2016; Miracle and Senkov, 2017; Nene et al., 2020). Figure 1 reports the evolution in alloy design from conventional alloy design to complex concentrated alloy (CCA) design via HEAs with respect to the hyperdimensional compositional diagram displaying the focus of the design in each case.
Recent advances in the field of additive manufacturing (AM) enable the making of complex engineering geometries through layer-by-layer deposition using either electron beam (direct energy deposition, DED) or laser (laser powder bed fusion, LPBF) as energy sources based on the CAD modelling of the components. This method is specifically classified under fusion-based AM, and a lot of research is being conducted in this direction, and 3D printing of conventional alloys such as steels, Al alloys, and Ti alloys has been carried out successfully (Debroy et al., 2018). Another avenue which is just getting started in AM is solid state–based AM developed by MELD manufacturing, United States. This approach uses friction stir processing/welding (FSP/FSW) as a basis for carrying out 3D printing of metallic alloys with the help of consumable tool. This technique has already shown triumphant 3D printing of light-weight alloys such as Al alloys and Ti-based materials; however, this technology has not yet extended to advanced high strength materials such as steels (Mishra et al., 2022). Major issues faced by the research community in this domain, irrespective of the AM technique or material being used, is the presence of porosity and crack type defects in the printed parts (Debroy et al., 2018; Mishra et al., 2022). As a result, transformative high entropy alloy (T-HEA) design may provide an effective solution to the everlasting issue of defects and printability in AM of metallic alloys, which are discussed in brief in upcoming sections.
Flexible HEA Design Leading to T-HEAs
Recent attempts by Nene et al. (2020) availed the metastability of phases synergized with high configurational entropy to tune the deformation mechanisms and thereby obtain an extraordinary combination of strength and ductility and yield strength for non-equiatomic dual-phase HEAs. Furthermore, they named these HEAs as T-HEAs since these HEAs showed pronounced γ (f.c.c.) → ε (h.c.p.) transformation during plastic deformation (typically known as the TRIP effect). They claimed that the key step in their work was the addition of Si into the Fe-Mn-Co-Cr matrix with minor additions of Cu and Al, followed by the use of friction stir processing (FSP) which altered the extent of this transformation event in the material.
Interestingly it was found that the addition of Si promoted the dominance of the ε phase in the microstructure, whereas Cu and Al addition, along with Si, brought back the γ majority in the microstructure (as shown by Figures 2A–D for Fe40Mn20Co20Cr15Si5 (CS-HEA), Fe39Mn20Co20Cr15Si5Al1 (Al-HEA), and Fe38.5Mn20Co20Cr15Si5Cu1.5 (Cu-HEA)). This observation was found to be consistent after rolling (Nene et al., 2021, Figures 2E–H) and the laser powder bed fusion (Agrawal et al., 2020, Thapliyal et al., 2020, Thapliyal et al., 2021, Figures 2I–K) of these HEAs, as shown in Figure 2. It is also to be noted that the addition of B4C particles during the 3D printing of the CS-HEA (Figure 2K) for similar processing parameters showed reversed phase stability, thereby showing γ richness in the microstructure with equiaxed grain morphology. This was a major breakthrough of the work since obtaining equiaxed morphology of grains while maintaining good printability of the material is very rare in the earlier work of 3D printing of metallic materials (Agrawal et al., 2020; Thapliyal et al., 2020; Thapliyal et al., 2021).
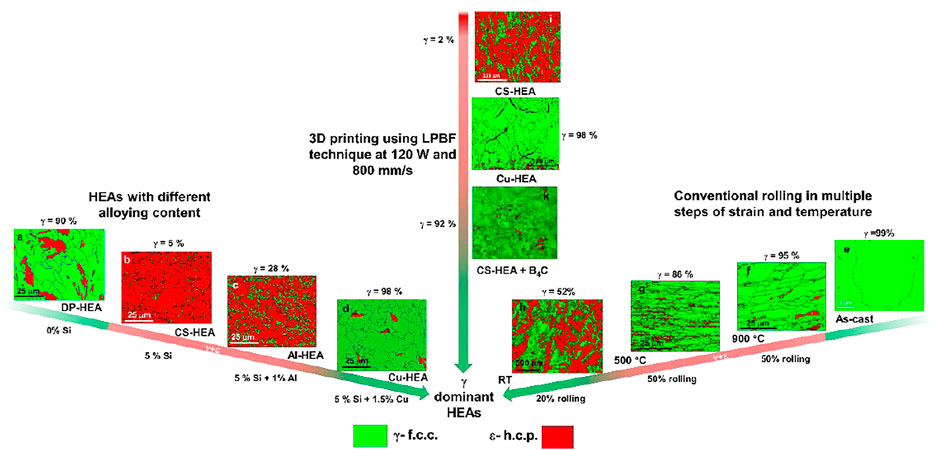
FIGURE 2. Flexible microstructural evolution in designed HEAs after (A–D) compositional variation (Nene et al., 2020), (E–H) conventional rolling (Nene et al., 2021), and (I–K) LPBF assisted 3D printing (Agrawal et al., 2020; Thapliyal et al., 2020; Thapliyal et al., 2021).
Unexpected Strength-Ductility Synergy in T-HEAs
As shown in Figures 3A-d2, these HEAs showed very unexpected stress-strain response along with the microstructural evolution with respect to variation in alloy chemistry and processing route (Agrawal et al., 2020; Nene et al., 2020; Thapliyal et al., 2020; Nene et al., 2021; Thapliyal et al., 2021). Figure 3A displays the plot of strength-ductility index (SDI = (UTS-YS)*total plastic elongation) values with respect to the total elongation obtained in a variety of T-HEAs discussed in Figure 2. SDI values indicate the WH ability (WH) of the material, and the higher the SDI value, the better the strength-ductility synergy for the material. Irrespective of the processing conditions and the alloying additions, T-HEAs showed an increase in SDI with total elongation of the material. This unexpected behavior points to the higher WH tendency in these materials with an increase in ductility, thereby concurring the conventional strength-ductility paradigm and is mainly attributed to the activation of multiple deformation modes during plastic straining.
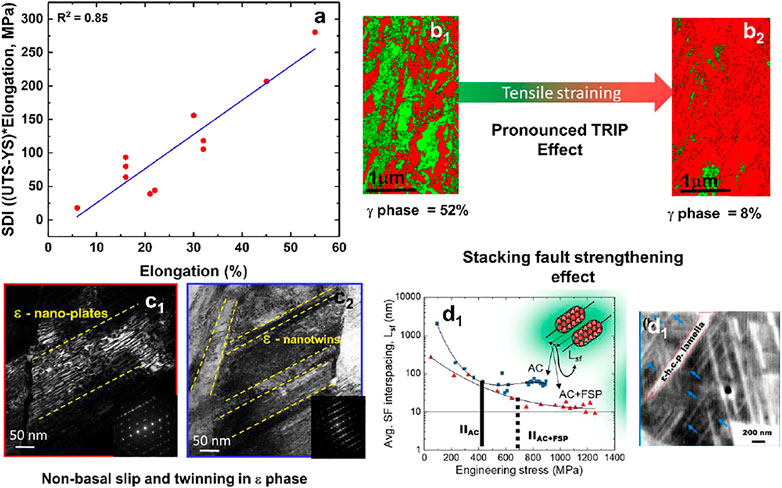
FIGURE 3. Unexpected stress-strain response in T-HEAs: (A) SDI vs total elongation plots for all T-HEAs processed with various processing routes. EBSD phase maps for Cu-HEA (b1) before and (b2) after deformation showing the TRIP effect. Bright-field TEM images showing the formation of (c1) nano-plates and (c2) nano-twins in the ε phase. (d1) Plot of avg. SF interspacing and engineering stress, and (d2) high-resolution BSE image showing SFs and ε lamella in Cu-HEA (Nene et al., 2020; Frank et al., 2021; Nene et al., 2021).
Figures 3b1-b2 show the presence of a pronounced TRIP effect during tensile straining (Nene et al., 2021), whereas Figures 3c1-c2 display the occurrence of nano-plate and nano-twin formation within the ε phase after deformation (Nene et al., 2020). Formation of plates in the early stage of deformation, whereas twinning during later stages of deformation is attributed to pronounced non-basal activity within the ε phase (Nene et al., 2020) owing to a low c/a ratio (1.49–1.55). These evidences point toward the co-existence of TRIP as a primary mechanism in the γ phase, whereas twinning and non-basal slip in the ε phase, thereby contributing to high WH ability.
Moreover, Figures 3d1-d2 denote the occurrence of stacking fault (SF) strengthening (Frank et al., 2021) in these T-HEAs, which is considered to be rare in conventional materials. The presence of SF strengthening was captured through in-situ neutron diffraction studies by calculating the variation in the Avg. SF interspacing (LSF) as a function of engineering stress (σeng, Figure 3d1). It is confirmed that the plateau in the LSF vs. σeng corresponds to the onset of transformation induced deformation and before which SF strengthening is dominant. This is because the plateau in Figure 3d1 is associated with the critical LSF value at which arrays of faults are accumulated with spacing dimensions (LSF) that can be detected as HCP martensite during neutron diffraction. It is to be noted that SF strengthening is predominantly active in those compositions where the stability of SFs is large, that is, SF energy (γ) is very low. Most of these T-HEAs have γ values lying within the range of 6–30 mJ/m2and hence show the occurrence of SF strengthening with varying extent based on the γ values (Frank et al., 2021).
Damage Tolerance in T-HEAs
Defects are an integral part of the engineering materials and lead to their sudden failures during service. Defects such as pores, voids, cracks and impurities are the sites for the initiation of stress concentration which impose propagation of these defects leading to ultimate failure. There appeared typical types of failures such as tensile, fatigue, creep, and corrosion fatigue which have their identification marks in the microstructures. Many attempts were made by the researchers to minimize the defect density during the fabrication or processing of engineering materials to improve their damage tolerance (Debroy et al., 2018; Mishra et al., 2022).
Recent advancements in the field of manufacturing brought to the floor LPBF technology to carry out 3D printing of the engineering components. LPBF was found to be a very potent technique to obtain complex engineering shapes with extreme precision; however, with a major drawback of porosity in the components irrespective of the metallic system used for printing. This limitation of porosity has restricted the use of these components for service due to the probability of sudden failures during application (Debroy et al., 2018; Mishra et al., 2022).
Our recent work on the fatigue behavior of transformative Cu-HEA (Liu et al., 2019) showed an exceptional endurance (∼750 MPa) limit in ultrafine grained microstructure owing to the unique phenomenon named “transformation induced crack retardation (TRICR)” as shown in Figures 4A–C. This phenomenon is a result of the local γ→ε transformation (TRIP effect) within the crack tip plastic zone which promoted WH activity and diverted the crack path (as shown in Figures 4B,C). It is to be noted that the plausibility of local transformation events near the defects is heavily dependent on the critical stress required for transformation (σTRIP) for a particular alloy. It is reported in earlier studies on T-HEAs (Mishra and Nene, 2021) that decreased metastability of the γ phase promotes the controlled TRIP effect and hence increases the σTRIP value. Thus, stress concentration within the plastic zone (σplastic zone) of the defects should exceed the σTRIP value for successful activation of the TRICR event in the material. As the stress concentration near the defects, in general, is of higher magnitudes, a higher magnitude of σTRIP value can also lead to local transformation activity in the material.
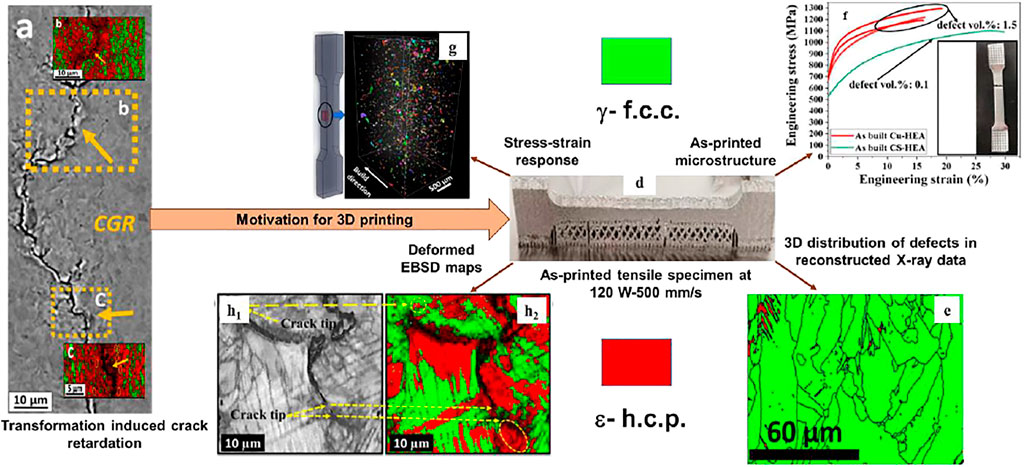
FIGURE 4. Damage tolerance in Cu-HEA after 3D printing: (A) Transformation induced crack retardation in ultrafine grained Cu-HEA. (B and C) Localized γ→ε transformation within the crack tip plastic zone (Liu et al., 2019). (D) 3D printed Cu-HEA tensile specimen using LPBF technique at the parameters of 120 W and 500 mm/s (Thapliyal et al., 2020). (E) X-ray microscopy 3D reconstruction representing the distribution of microcracks and pores within as-built Cu-HEA (Thapliyal et al., 2020). (F) Engineering stress–strain response of as-build Cu-HEA (Thapliyal et al., 2020). (G) EBSD phase map showing the microstructure of as-built Cu-HEA. (h1 and h2) EBSD phase map showing localized γ→ε transformation near the crack type defects in as-deformed Cu-HEA (Thapliyal et al., 2020).
Earlier work on Cu-HEA (Nene et al., 2021) displayed a very high deformation storage capacity and controlled TRIP effect due to σTRIP values ranging from 750 MPa to 1.8 GPa based on the microstructure (Mishra and Nene, 2021). This wide range of σTRIP motivated us to utilize this unique characteristic in Cu-HEA to apply to the 3D printed materials fabricated using LPBF. This is because defects are an inherent part of the 3D printed components, and if such a local transformation event can be triggered near those defects, the damage can be delayed and resistance to failure can be improved during service.
Figure 4D shows the 3D printed tensile specimen using the LPBF technique at parameters of 120 W and 500 mm/s for Cu-HEA, whereas Figure 4E displays the complete dominance of the γ phase after 3D printing in the EBSD phase map. Figure 4G shows higher strength and comparable ductility for as-printed Cu-HEA despite having a higher value of defect density (1.5%, Figure 4G) compared to as-printed CS-HEA. In conventional materials, higher defect density after 3D printing promotes early failure (at lower strength), thereby showing poor damage tolerance. This emphasizes the presence of higher damage tolerance in Cu-HEA after 3D printing, which is mainly attributed to the pronounced TRICR effect associated with local γ→ε transformation near the crack (as depicted in the EBSD phase map in Figures 4h1-h2) type defects as discussed earlier (Thapliyal et al., 2020). Hence, 3D printing of Cu-HEA displayed a new pathway in realizing not only work hardenable but also damage tolerant materials for 3D printing.
Future Prospects of Transformative Alloy Design for Metal Additive Manufacturing
3D printing of metallic components is picking huge interest among researchers due to its high potential in producing complex engineering geometries in a much more cost-effective manner with minimized processing steps. Various methods of metallic 3D printing such as DED and LPBF were attempted to obtain acceptable 3D components with metallic systems such as stainless steel, Ti-6Al-4V, and Al-Si alloys. Despite having successful 3D printing using either of these techniques for earlier mentioned materials, there appeared issues associated with porosity, and reflectivity under lesser and dramatic changes in the alloy composition due to selective evaporation of certain elements under high energy beams (Debroy et al., 2018; Mishra et al., 2022). Solid-state AM showed some hopes of minimizing these issues in fusion-based AM; however, displayed constraints on the printability of high strength alloys.
Transformative alloy design can be an effective path to rectify all these problems in metal additive manufacturing irrespective of the printing of components using fusion or solid-state route. Using this approach, the defects present in 3D printed components can themselves act as failure delaying sites by the activation of the TRICR effect near the flaws. The delay in failure due to local plastic deformation near the defects can therefore be termed as defect induced plasticity (DIP) effect. The occurrence of the DIP effect also appears to be very sensitive to alloy chemistry getting 3D printed owing to metastability controlled γ→ε transformation near the defects in transformative alloys. Hence the design of alloy compositions which not only show good printability but also tunable metastability should be carried out as a first step toward this effort.
Second, a systematic study of the defects has to be performed to verify the effectiveness of the DIP effect. It is expected that certain morphology, size, and distribution of the defects may be useful for displaying a pronounced TRICR effect in the material and hence the optimum DIP effect. Parametric variation during metal 3D printing can be employed to vary the defect nature, fraction, and distribution in the microstructure of the material to realize the optimum defects promoting the DIP effect. Successful implementation of this transformative alloy design and processing approach will enable solving the everlasting issue of porosity in AM components. This is because materials having optimized metastability and defective nature can give a pronounced DIP effect during service. Hence, defects present in the AM components can become local sites to give plasticity, thereby delaying the failure of the components during service. This effort thus will improve the performance and sustainability of 3D components during service. Figure 5 captures the futuristic transformative high entropy alloy design strategy leading to materials availability for sustainable metal additive manufacturing.
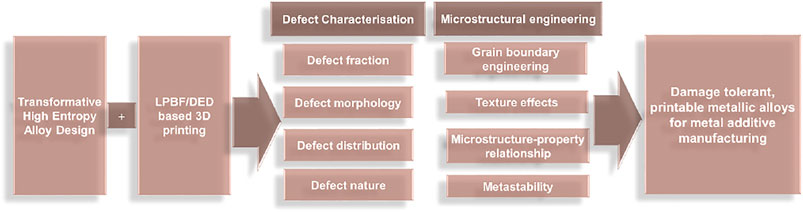
FIGURE 5. Futuristic transformative high entropy alloy design for sustainable metal additive manufacturing.
Summary
In summary, transformative high entropy alloy (T-HEAs) design has proven to be a very effective pathway in obtaining flexible microstructural evolution promoting the unexpected strength-ductility synergy at room temperature. Moreover, engineered metastability in these T-HEAs made them prone to have exceptional properties such as high damage tolerance and acceptable printability during additive manufacturing. This cocktail effect in T-HEAs is attributed to the synergistic activation of multiple deformation modes such as TRIP, non-basal slip assisted plasticity in the ε phase, and stacking fault (SF) strengthening at different stages of deformation. As a result, T-HEAs can be considered as potential futuristic candidates for realizing stronger, printable, and damage tolerant materials for sustainable metal additive manufacturing.
Data Availability Statement
The raw data supporting the conclusions of this article will be made available by the authors, without undue reservation.
Author Contributions
SN has conceptualized and drafted the manuscript. SN has contributed as a leading researcher in most of the work reported in the manuscript on T-HEAs.
Conflict of Interest
The author declares that the research was conducted in the absence of any commercial or financial relationships that could be construed as a potential conflict of interest.
Publisher’s Note
All claims expressed in this article are solely those of the authors and do not necessarily represent those of their affiliated organizations, or those of the publisher, the editors, and the reviewers. Any product that may be evaluated in this article, or claim that may be made by its manufacturer, is not guaranteed or endorsed by the publisher.
Acknowledgments
The author is thankful to Prof. R.S. Mishra for providing eminent guidance for the research work on high entropy alloys. SN also thanks Dr. Subhasis Sinha, Dr. Michael Frank, Dr. K. Liu, Dr. Saket Thapliyal, and Ms. Priyanshi Agrawal for their constant support and help in all the research work performed at the University of North Texas.
References
Agrawal, P., Thapliyal, S., Nene, S. S., Mishra, R. S., McWilliams, B. A., and Cho, K. C. (2020). Excellent Strength-Ductility Synergy in Metastable High Entropy Alloy by Laser Powder Bed Additive Manufacturing. Addit. Manuf. 32, 101098. doi:10.1016/j.addma.2020.101098
DebRoy, T., Wei, H. L., Zuback, J. S., Mukherjee, T., Elmer, J. W., Milewski, J. O., et al. (2018). Additive Manufacturing of Metallic Components - Process, Structure and Properties. Prog. Mater. Sci. 92, 112–224. doi:10.1016/j.pmatsci.2017.10.001
Estrin, Y., Nene, S. S., Kashyap, B. P., Prabhu, N., and Al-Samman, T. (2016). New Hot Rolled Mg-4Li-1Ca Alloy: A Potential Candidate for Automotive and Biodegradable Implant Applications. Mater. Lett. 173, 252–256. doi:10.1016/j.matlet.2016.03.052
Frank, M., Nene, S. S., Chen, Y., Thapliyal, S., Shukla, S., Liu, K., et al. (2021). Direct Evidence of the Stacking Fault-Mediated Strain Hardening Phenomenon. Appl. Phys. Lett. 119, 081906. doi:10.1063/5.0062153
Kim, H., Suh, D.-W., and Kim, N. J. (2013). Fe-Al-Mn-C Lightweight Structural Alloys: a Review on the Microstructures and Mechanical Properties. Sci. Technol. Adv. Mater. 14, 014205. doi:10.1088/1468-6996/14/1/014205
Li, Z., Pradeep, K. G., Deng, Y., Raabe, D., and Tasan, C. C. (2016). Metastable High-Entropy Dual-phase Alloys Overcome the Strength-Ductility Trade-Off. Nature 534, 227–230. doi:10.1038/nature17981
Liu, K., Nene, S. S., Frank, M., Sinha, S., and Mishra, R. S. (2019). Extremely High Fatigue Resistance in an Ultrafine Grained High Entropy Alloy. Appl. Mater. Today 15, 525–530. doi:10.1016/j.apmt.2019.04.001
Miracle, D. B., and Senkov, O. N. (2017). A Critical Review of High Entropy Alloys and Related Concepts. Acta Mater. 122, 448–511. doi:10.1016/j.actamat.2016.08.081
Mishra, R. S., and Nene, S. S. (2021). Some Unique Aspects of Mechanical Behavior of Metastable Transformative High Entropy Alloys. Metall. Mater Trans. A 52, 889–896. doi:10.1007/s11661-021-06138-3
Mishra, R. S., Haridas, R. S., and Agrawal, P. (2022). Friction Stir-Based Additive Manufacturing. Sci. Technol. Weld. Join. 27, 141–165. doi:10.1080/13621718.2022.2027663
Nene, S. S., Agrawal, P., Frank, M., Watts, A., Shukla, S., Morphew, C., et al. (2021). Transformative High Entropy Alloy Conquers the Strength-Ductility Paradigm by Massive Interface Strengthening. Scr. Mater. 203, 114070. doi:10.1016/j.scriptamat.2021.114070
Nene, S. S., Frank, M., Agrawal, P., Sinha, S., Liu, K., Shukla, S., et al. (2020). Microstructurally Flexible High Entropy Alloys: Linkages between Alloy Design and Deformation Behavior. Mater. Des. 194, 108968. doi:10.1016/j.matdes.2020.108968
Thapliyal, S., Agrawal, P., Agrawal, P., Nene, S. S., Mishra, R. S., McWilliams, B. A., et al. (2021). Segregation Engineering of Grain Boundaries of a Metastable Fe-Mn-Co-Cr-Si High Entropy Alloy with Laser-Powder Bed Fusion Additive Manufacturing. Acta Mater. 219, 117271. doi:10.1016/j.actamat.2021.117271
Thapliyal, S., Nene, S. S., Agrawal, P., Wang, T., Morphew, C., Mishra, R. S., et al. (2020). Damage-tolerant, Corrosion-Resistant High Entropy Alloy with High Strength and Ductility by Laser Powder Bed Fusion Additive Manufacturing. Addit. Manuf. 36, 101455. doi:10.1016/j.addma.2020.101455
Wang, L., Makhlouf, M., and Apelian, D. (1995). Aluminium die casting alloys: alloy composition, microstructure, and properties-performance relationships. Int. Mater. Rev. 40, 221–238. doi:10.1179/imr.1995.40.6.221
Keywords: transformative high entropy alloys, additive manufacturing, transformation induced plasticity, severe deformation, transformation induced crack retardation
Citation: Nene SS (2022) Some Distinct Features of Transformative High Entropy Alloys for Metal Additive Manufacturing. Front. Mater. 9:873911. doi: 10.3389/fmats.2022.873911
Received: 11 February 2022; Accepted: 22 April 2022;
Published: 02 June 2022.
Edited by:
Ravi Sankar Kottada, Indian Institute of Technology Madras, IndiaReviewed by:
Shengguo Ma, Taiyuan University of Technology, ChinaNiraj Chawake, Indian Institute of Technology Kanpur, India
Rajesh Korla, Indian Institute of Technology Hyderabad, India
Copyright © 2022 Nene. This is an open-access article distributed under the terms of the Creative Commons Attribution License (CC BY). The use, distribution or reproduction in other forums is permitted, provided the original author(s) and the copyright owner(s) are credited and that the original publication in this journal is cited, in accordance with accepted academic practice. No use, distribution or reproduction is permitted which does not comply with these terms.
*Correspondence: S.S. Nene, c3NuZW5lQGlpdGouYWMuaW4=